Tandem-column extraction chromatography for Nd separation: minimizing mass-independent isotope fractionation for ultrahigh-precision Nd isotope-ratio analysis†
Received
22nd October 2021
, Accepted 2nd December 2021
First published on 2nd December 2021
Abstract
The short-lived 146Sm–142Nd isotope system traces key early planetary differentiation processes that occurred during the first 500 million-years of the solar system history. The variations of 142Nd/144Nd in terrestrial samples, typically within a range of ±20 ppm, are determined using high-precision mass spectrometry that requires quantitative separation of Nd from all other elements in the sample, including the neighboring lanthanides. Recent improvements in mass spectrometry have pushed the analytical precision of 142Nd/144Nd measurements down to ∼2 ppm. Non-mass-dependent isotope fractionation produced during Nd separation, however, is a major factor limiting the quality of the 142Nd data. Popular chemical separation methods using Ln resins have unpredictable nuclear field shift effects that generate anomalous Nd isotope ratios. In order to solve this problem and potentially resolve small 142Nd/144Nd variations within ±5 ppm, in this study, we present a new two-step column separation method that effectively removes the isobaric interferents of Ce, Pr and Sm, with a recovery rate of Nd greater than 98%. JNdi-1 standard solutions doped with these interfering elements and geological reference materials are tested to document the performance of this method. A set of titanite samples from the Pilbara Craton in western Australia were also investigated to test the potential isotope fractionation effects. The same samples were processed using our method and the widely used Ln method. In contrast to the nuclear field shift effects observed from the samples using the Ln method, the results based on our new method show no detectable isotope fractionation, which further confirms the reliability of this new column chemistry scheme that is optimized for ppm-level precision Nd isotope ratio measurement, especially for resolving small variations in 142Nd/144Nd caused by the decay of 146Sm.
1. Introduction
The decay of now extinct 146Sm with a half-life of 103 My1 produces 142Nd. Variations in 142Nd isotope abundance thus reflect processes that fractionated Sm from Nd that occurred in the first ∼500 million-years (My) of the solar system history. This time window provides important insights into early differentiation events on planetesimals and the Moon as well as the early Earth's initial differentiation and the formation of the first crust. Variations in 142Nd/144Nd are conventionally reported in parts per million (ppm) deviation relative to the JNdi-1 Nd standard2 that is assumed to represent the average 142Nd/144Nd in the modern mantle (μ142Nd = [(142Nd/144Ndsample/142Nd/144Ndstandard) − 1]). In rocks from the oldest terrestrial terranes, variations of μ142Nd ranging from −15 to +20 ppm have been measured by thermal ionization mass spectrometry (TIMS), with a typical isotope ratio precision of ±4 to 8 ppm over 3–6 months (external reproducibility, i.e., intermediate precision)3–7 or multi-collector ICP-MS.8 The magnitude of 142Nd anomalies in Archean rocks appears to decrease with time due to mantle mixing and eventually becomes hidden within the measurement precision by ∼2.5 Gy.8,9
In modern rocks, despite the evidence from other isotope ratios such as 3He/4He or 182W/184W that suggests preservation to the modern day of differentiated products in Earth's interior produced in the first ∼100 My of Earth's history,10,11 only extremely limited 142Nd/144Nd variability has been resolved in modern mantle-derived samples.12,13 In order to tackle this problem and also to improve data precision, recent work on techniques for 142Nd/144Nd analysis, as well as this study, has improved the measurement precision to ∼±2–3 ppm.8,14,15 With this level of measurement precision, wet chemistry preparation has become the bottleneck for ppm-level precision 142Nd/144Nd measurements. Small non-mass-dependent isotope fractionation (i.e., nuclear field shift effect) during the chemical separation of Nd, which was negligible in the past when mass spectrometric procedures limited isotope ratio precisions to >±5 ppm, however, can be greater than the current measurement precision (2–3 ppm) and thus create isotopic differences between the measured 142Nd/144Nd and that present in the original rock sample. A reliable Nd separation protocol that repeatedly provides quantitative separation of Nd with limited or no isotope fractionation is thus needed for the 142Nd/144Nd isotope measurements of ppm-level precision.
Natural silicate rocks have similar Ce, Nd and Sm mass fractions and given the isobaric interference of 142Ce and 144Sm on 142Nd/144Nd ratios, an ideal chemical separation would achieve ppm level Ce/Nd or Sm/Nd ratios.14 Widely used methods that effectively remove Ce and Sm include the 2-methyllactic acid (MLA) method,4,16 Ce oxidation-Ln method,17 liquid–liquid extraction coupled with Ln columns,18 and repeated tandem TRU-Ln2-DGA-Ln2 columns.19 These methods generally involve many different sets of columns, which requires significant effort for the analyst (ranging from 20 hours to 4 days). Some recent studies have found that unpredictable Nd mass-independent isotope fractionation occurs in the Ln columns, which is interpreted as a consequence of the nuclear field shift effect.14,20 High Nd recovery (>95%) is crucial for minimizing this effect.21 The MLA and liquid–liquid extraction methods, in comparison, have not been reported to generate significant isotope fractionation, but the column recovery rates are inconsistent (60–90%), which makes these methods less ideal.4,14,18 In this study, we present a two-step chemical separation scheme that yields a high-purity, high-recovery Nd separation in a relatively short time (∼14 hours total column chromatography time), with the most important advantage of showing no detectable mass-independent Nd isotope fractionation, which is especially useful for increasing efforts towards the search for small 142Nd/144Nd variations within ∼±5 ppm.
2. Experimental section
2.1 Materials and reagents
Ultrapure water (Milli-Q, Merck-Millipore) with a resistance of 18 MΩ cm produced from deionized water was used throughout as the dilutant for all acids. Hydrochloric and nitric acids were double distilled from ACS grade reagents using a sub-boiling PFA distiller (Savillex). Ultrapure, pre-distilled, hydrofluoric acid (Optima) was used without in-house distillation. Sodium bromate (Aldrich, purity > 99.5%) was used to prepare a 10 M HNO3–20 mM NaBrO3 solution.
One cation exchange resin (AG 50 W-X8, 200–400 mesh, Bio-Rad) and two extraction chromatographic resins (Ln-spec resin and DGA-N resin, 50–100 μm, Eichrom) were used for Nd separation. Four types of disposable polypropylene columns were used, including two slightly different 2 ml columns both with an 8 mm inner diameter (Poly-Prep®, Bio-Rad, and Eichrom) for light rare earth element (LREE, e.g., La, Ce, Pr, Nd, and Sm) separation from major elements, a 1.2 ml column with a 7 mm inner diameter for Ce removal (Mini Bio-Spin®, Rio-Rad), and a 1 ml column with a 5 mm inner diameter (Muromac Mini-column S, Muromachi Chemicals) for final purification of Nd. AG50W-X8 and Ln resins were wetted in water and cleaned with 6 M HCl prior to use. The wetted DGA-N resin was soaked in 2 M HNO3 for at least overnight and then sonicated for an hour. Some foam-like suspended materials were discarded. Because the DGA-N resin tends to float in nitric acid, a tightly fit top frit is necessary for packing the columns.
2.2 Samples
Geological reference materials and natural rock samples are investigated in order to test the efficiency of column chemistry. The reference materials include basaltic rock powders BHVO-2 and BCR-2 and the Nd isotope standard JNdi-1. Two fractions of BHVO-2 and BCR-2 each weighing ∼100 mg were dissolved and processed through the column chemistry protocol. Aliquots (∼2 μg) of JNdi-1 were doped with similar amounts of interference elements (i.e., Ce, Pr and Sm) and treated as unknown materials. A set of granitic rocks from the eastern Pilbara Craton were also investigated as a case study. Titanite grains (∼5 mg per aliquot) separated from these rocks were dissolved and processed through column chemistry for each sample.
2.3 Decomposition of silicate samples
The decomposition of the samples follows the dissolution method described in ref. 14. Approximately 100 mg basaltic rock powders were dissolved in a 15 ml PFA vial with a mixture of 5 ml 29 M HF and 1 ml 14 M HNO3. The PFA vials were capped and placed on a hotplate set to 130 °C for at least 48 hours. The titanite grains were dissolved in a 1 ml HF–HNO3 mixture due to the smaller sample size. After evaporation to dryness, the samples were treated with 5 ml concentrated HNO3 twice to remove fluorides, followed by 5 ml concentrated HCl with the samples taken to dryness in between the acid additions. The samples were then re-dissolved in 6 M HCl at 130 °C on a hotplate overnight in order to be completely converted to the chloride form. Once a clear solution was achieved, the samples were dried and re-dissolved in 2 ml 2.5 M HCl or 3 ml 3 M HNO3 for column loading.
2.4 Column chemistry
The chemical separation of Nd in this study includes two steps (Fig. 1): the first step is to separate Nd along with the bulk of LREEs from the rock's matrix elements; the second step is to purify Nd from its isobaric interference elements (i.e., Ce and Sm), with the goal of reaching ppm level Ce/Nd and Sm/Nd ratios.
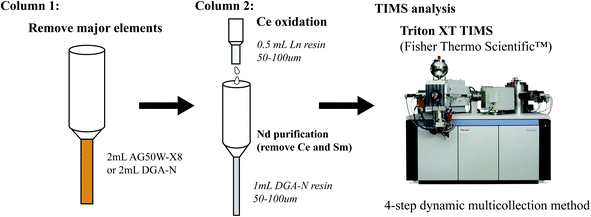 |
| Fig. 1 A schematic showing the two-step column chemistry protocol. | |
2.4.1 First-step column.
Two types of columns were explored for the first step separation: a conventional cation exchange column (2 ml AG50W-X8) to separate the bulk LREEs, named the “primary cation exchange column”, similar to that described in ref. 14 and an extraction chromatographic column (2 ml DGA-N resin) to extract Nd, modified after ref. 22, named the “primary DGA column”.
(1) Primary cation exchange column.
Bio-Rad Poly-Prep columns packed with 2 ml AG50W-X8 cation exchange resin were used for the primary separation of REEs from other elements. The procedure for this column is outlined in ref. 12. The resin bed was cleaned with 40 ml 6 M HCl and back washed with 2.5 M HCl prior to loading the samples. The sample in 2 ml of 2.5 M HCl was loaded onto the column, and the major elements were eluted with 13 ml 2.5 M HCl. The fraction containing REEs was then collected with 10 ml 6 M HCl.
(2) Primary DGA column.
For samples less than ∼100 mg, the DGA resin has been shown to be effective for the primary extraction of Nd.22,23 Two milliliters of DGA-N resin were packed in an Eichrom polypropylene column, cleaned with 50 ml 0.05 M HCl, and subsequently conditioned with 3 M HNO3. Because rare earth cations have much higher distribution coefficients in HNO3 than in HCl,23 the sample solution in 3 ml 3 M HNO3 was loaded into the primary DGA column. The major elements were first eluted with 20 ml 3 M HNO3; Pb and Sr (and potentially excess Ca) were then washed with an additional 20 ml 10 M HNO3. Following this step, HCl was used in order to elute the rare earth elements. Before changing the acid medium from strong HNO3 to HCl, the column must be completely clear of the 10 M HNO3 in order to avoid the generation of gas bubbles in the resin bed. The light rare earth elements La, Ce and Pr can be eluted with 28 ml 2.5 M HCl. The Nd fraction was then collected with 24 ml 2.5 M HCl. The columns can be cleaned with 50 ml 0.05 M HCl and stored wet in 0.05 M HNO3 for reuse.
2.4.2 Second-step tandem columns.
The second step columns are a set of tandem columns consisting of a Ce oxidation column and a second-stage DGA column. The Ce oxidation column was modified after ref. 12 and 24. Ln resin (0.5 ml, 50–100 μm) was loaded in a Bio-Rad Mini Bio-Spin polypropylene column. In this column, Ce3+ is oxidized to Ce4+ which complexes with the HDEHP of the Ln resin and remains absorbed on the resin, and therefore can be effectively separated from other trivalent REEs. The second-stage DGA column used a Muromac Mini-column S polypropylene column filled with 1 ml DGA-N resin (50–100 μm). The volume of the resin is precisely set based on a bed length of 4.5 cm and the HCl is accurately titrated at 2.50 M, as the LREE elution using the DGA resin is very sensitive to the molarity of HCl and also the volume of the resin.
The resin bed of the Ce oxidation column was cleaned successively with 5 ml 6 M HCl, 2 ml H2O, and 6 ml 10 M HNO3, and then conditioned with 2 ml 10 M HNO3–20 mM NaBrO3. At the same time, the pre-cleaned (with 30 ml 0.05 M HCl) second-stage DGA column was conditioned with 3 ml 10 M HNO3. Once both columns are cleaned and conditioned, the second-stage DGA column was placed with its reservoir right underneath the Ce oxidation column. The upper Ce oxidation column functions as a filter of Ce which only drips Ce-free REEs onto the second-stage DGA column.
The fraction containing LREEs from the primary column was re-dissolved in 0.4 ml 10 M HNO3–20 mM NaBrO3, and loaded onto the Ce oxidation column. LREEs were subsequently eluted with 3 ml 10 M HNO3–20 mM NaBrO3. The total 3.4 ml elution containing LREEs and Na (from sodium bromate) drips directly into the second-stage DGA column. Once the Ce-column elution was completely into the lower column, the upper Ce oxidation column was removed, and 10 ml 3 M HNO3 was added into the lower DGA column in order to remove any remaining matrix elements. Lanthanum, Ce and Pr were eluted using 14 ml 2.5 M HCl, and Nd was finally collected in 11 ml 2.5 M HCl. The procedure for the second-step tandem columns is summarized in Table 1. In order to reduce organic materials in the Nd fraction for TIMS analysis, the eluent was dried down and treated with concentrated HNO3 three times.
Table 1 Nd purification scheme using the tandem LN-DGA columns
Procedures |
Eluting reagent |
Eluting volume (mL) |
Column cleaning
|
LN column |
6 M HCl, H2O, 10 M HNO3 |
5 ml, 2 ml, 6 ml |
DGA column |
0.05 M HCl |
30 ml |
![[thin space (1/6-em)]](https://www.rsc.org/images/entities/char_2009.gif) |
Column conditioning
|
LN column |
10 M HNO3–20 mM NaBrO3 |
2 ml |
DGA column |
10 M HNO3 |
3 ml |
![[thin space (1/6-em)]](https://www.rsc.org/images/entities/char_2009.gif) |
Columns in tandem
|
Loading sample |
10 M HNO3–20 mM NaBrO3 |
0.4 ml |
Rinse |
10 M HNO3–20 mM NaBrO3 |
3 ml |
![[thin space (1/6-em)]](https://www.rsc.org/images/entities/char_2009.gif) |
Remove upper LN column
|
Rinse Na, Ba and other matrices |
3 M HNO3 |
10 ml |
Rinse La, Ce, and Pr |
2.5 M HCl |
14 ml |
Collect Nd |
2.5 M HCl |
11 ml |
![[thin space (1/6-em)]](https://www.rsc.org/images/entities/char_2009.gif) |
Optional Sm separation
|
Rinse |
2.5 M HCl |
2 ml |
Collect Sm |
0.5 M HCl |
4 ml |
2.5 Thermal ionization mass spectrometry
The Nd isotopic compositions were measured using a Triton XT TIMS (Thermo Scientific) with a double filament assembly using pre-degassed zone-refined Re filaments (H. Cross), at the Earth and Planets Laboratory, Carnegie Institution for Science, using procedures that followed those described in ref. 12 and 14. The recovery rate of the separated Nd sample was checked on a Thermo Scientific iCAP-Q quadrupole inductively coupled plasma mass spectrometer (Q-ICP-MS). The dried Nd sample was re-dissolved with 3 M HCl, and a sample amount of 600–800 ng of Nd (1–2 μl) was loaded onto the middle third of the filament, after 1 μl 0.1 M H3PO4, using melted parafilm as a dam to stop the sample spreading across the whole filament. The sample is loaded as a spot as small as possible on the filament (1–2 mm width) in order to minimize the potential domain mixing effect during sample evaporation.25
The Nd isotopic data were collected using a four-step dynamic routine with center masses of 143, 144, 145 and 146, which minimizes the bias of different cup efficiencies.12 The mass fractionation was corrected using the exponential law, applied to the time-corrected, measured 146Nd/144Nd ratios relative to 146Nd/144Nd = 0.7219.12,26 In this way, we can obtain dynamic ratios for 142Nd/144Nd, 143Nd/144Nd, 145Nd/144Nd and 148Nd/144Nd. A full sample measurement takes about 12 hours, which consists of 30 blocks, with 30 4-step cycles (8.39 s integration time each step) in each block (1800 dynamic 142Nd/144Nd ratios). The measurement was aborted early if the sample filament was highly fractionated (e.g., 146Nd/144Nd > 0.725) or the 142Nd signal dropped below 10−11 A. Most runs yielded a steady 142Nd signal between 5 and 7 × 10−11 A for more than 700 cycles, and those measurements that lasted less than 450 cycles were rejected.
3. Results and discussion
3.1 Performance of the separation scheme
3.1.1 Column yields and purity.
The first-step column.
We used two schemes for the primary separation of the Nd fraction from other elements in the sample: a traditional and widely used cation exchange column and an extraction chromatographic column using DGA-N resin. To avoid any potential isotopic fractionation in column chemistry, we used a small column with a low length-to-diameter ratio (∼5
:
1), and thus the eluent from the cation exchange column is not a pure LREE, but also contains Ca, Sr, Ba and other REEs.24 In comparison, in the DGA primary column, the major elements (e.g., Na, Mg, Al, Fe, Ca, K, etc.) were effectively removed using 3 M HNO3. Ba and Sr were also removed using 3 M and 10 M HNO3, respectively. The elution profile for the primary DGA column determined using 100 mg BCR-2 is given in Fig. 2. A recent study documented the retention of Ca in the DGA column tail into the Nd fraction.22 However, this was not seen in our elution profile, which possibly reflects our longer resin bed (5 cm vs. 2 cm). Our longer resin bed also yielded a better separation of Nd. In general, >99.5% Pr and >99.9% La and Ce were removed, with a final Nd recovery of >99.8%. The last 2 ml of 2.5 M HCl that contains ∼0.5% of the total Nd may be discarded in order to avoid collecting any potential trace Sm (isobaric interference of Nd). Because the aim of the primary column is to remove the major elements from the sample for further purification using the second-step tandem columns, our protocol was optimized for the recovery of Nd. Since both columns were able to recover >99% Nd, we estimate a minimum capacity of ∼100 mg for the basaltic rock sample, which is generally sufficient for bulk-rock 142Nd isotope ratio analysis (typically ∼2 μg Nd in the 100 mg rock sample).
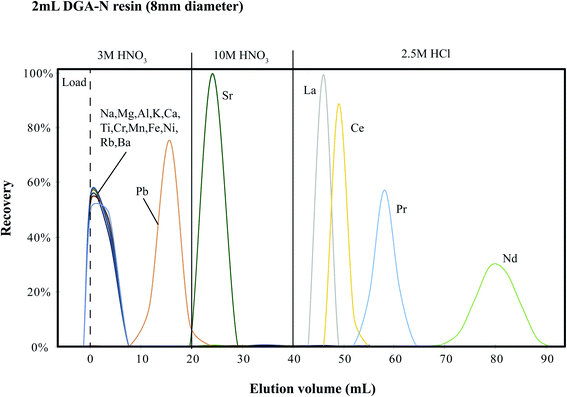 |
| Fig. 2 Elution profile of the 2 ml primary DGA column. | |
The total sample preparation procedure for the traditional cation exchange column takes ∼3.5 hours, while the primary DGA column takes ∼10 hours. The cation exchange column elutes the bulk REEs, which is less likely to cause Nd isotopic fractionation. Therefore, for the ppm-level ultrahigh-precision 142Nd/144Nd analysis, we prefer use of the cation exchange column as the first step. For 143Nd/144Nd analysis alone, which does not require sub-5 ppm precision or is compromised by the presence of moderate amounts of Ce or Pr, our primary DGA column can be used as a one-step scheme for Nd extraction.
Second-step tandem columns.
Taking advantage of the contrasting distribution coefficients of Na+ and REEs in 10 M HNO3,23 the eluent containing REEs from the oxidation Ln column can be directly loaded onto the second stage DGA column without a lengthy dry-down, where Na (from NaBrO3) is not absorbed during loading while the REEs are retained in the resin. Fig. 3 shows the elution profile of the second-stage DGA column determined with a synthetic REE solution mixed with Ba (∼2 μg each element). Excess Na (∼50 μg) was also added in order to test its capacity for removing a large amount of sodium. The second-stage DGA column is similar to the primary DGA column, except that the volume of the resin bed was reduced by half (1 ml) with a 10
:
1 length-to-diameter ratio (∼4.5 × 45 mm). The tandem columns remove >99.9% La, Ce and Pr, with a Nd recovery of >98%. According to our elution profile, a recovery of Nd as high as 99.7% can be achieved (Fig. 3). Because our second-step column is optimized for the purity of Nd, the tail (∼2 ml) of the Nd elution curve (∼1.5% total Nd) was thus not collected in order to avoid any trace Sm present in the collection. With this tandem column scheme, we were able to achieve a separation factor of Ce/Nd and Sm/Nd of about 1 × 10−6. The total process including resin cleaning takes ∼10 hours.
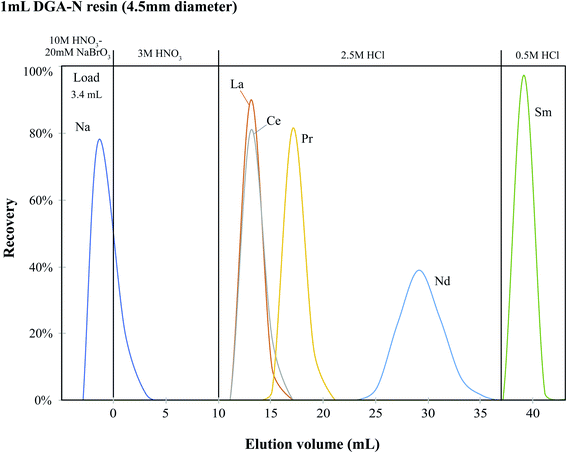 |
| Fig. 3 Elution profile of the 1 ml second-stage DGA column. | |
3.1.2 Blank.
The chemical blank was determined using a 150Nd enriched isotope tracer. The total procedure blank for Nd, including sample digestion, column chemistry and filament loading, is 20 pg, which is negligible compared with the typical amounts of Nd (600–800 ng) used for a single 142Nd measurement.
3.2 Doped JNdi-1 and geological reference materials
The column separation method was verified by four aliquots of doped JNdi-1 neodymium standard solutions and dissolutions of two geological reference materials (BHVO-2 and BCR-2). A summary of the μNd results is given in Table 2, with the full ratios given in ESI Table S1.† Four aliquots of 2 μg JNdi-1 solution were doped with 2 μg Ce, Pr and Sm, similar to the compositional range of natural rocks. Each analysis is performed on a separate aliquot. The doped JNdi-1 analyses yielded 140Ce/144Nd and 147Sm/144Nd ratios during TIMS analysis which were mostly <1 ppm, with two outliers between 4 and 6 ppm. The doped JNdi-1 analyses yielded identical isotopic ratios to the unprocessed pure JNdi-1 standards, with average dynamic μ142Nd, μ143Nd, μ145Nd, and μ148Nd values (relative to the standard, in ppm) of −0.6 ± 3.1, −1.7 ± 3.5, 1.2 ± 2.8, and 0.3 ± 8.9, respectively.
Table 2 The μNd values (in ppm) and interference/Nd ratios of processed geological reference materials and titanite samples
Sample |
μ
142Nd |
2SE |
μ
143Nd |
2SE |
μ
145Nd |
2SE |
μ
148Nd |
2SE |
140Ce/144Nd |
147Sm/144Nd |
Doped Jndi-1
|
B34F3 doped JNdi-1 a1 |
−0.6 |
1.9 |
−0.1 |
1.4 |
1.1 |
1.2 |
−5.3 |
2.7 |
4.0 × 10−6 |
2.3 × 10−7 |
B34F4 doped JNdi-1 b1 |
−0.9 |
2.0 |
−3.9 |
1.6 |
−0.7 |
1.4 |
5.6 |
3.1 |
−2.4 × 10−7 |
8.8 × 10−7 |
B36F5 doped JNdi-1 a2 |
−2.2 |
2.6 |
−0.6 |
2.0 |
2.5 |
1.8 |
1.0 |
4.0 |
9.1 × 10−7 |
8.3 × 10−7 |
B36F6 doped JNdi-1 b2 |
1.5 |
2.8 |
−2.1 |
2.2 |
2.0 |
1.7 |
0.0 |
4.1 |
8.9 × 10−7 |
6.3 × 10−6 |
Mean and 2SD
|
−0.6
|
3.1
|
−1.7
|
3.5
|
1.2
|
2.8
|
0.3
|
8.9
|
|
|
![[thin space (1/6-em)]](https://www.rsc.org/images/entities/char_2009.gif) |
BHVO-2
|
B31F16 BHVO-2 d1 |
−0.2 |
2.2 |
1702.7 |
1.8 |
0.8 |
1.4 |
−3.5 |
3.1 |
3.9 × 10−7 |
4.3 × 10−7 |
B33F5 BHVO2 d1 |
−0.9 |
2.1 |
1701.2 |
1.6 |
1.0 |
1.3 |
−1.6 |
3.0 |
−2.0 × 10−7 |
6.8 × 10−7 |
B33F6 BHVO2 d2 |
−0.4 |
3.4 |
1702.3 |
2.6 |
−2.0 |
2.3 |
−2.5 |
5.3 |
8.7 × 10−7 |
−2.7 × 10−7 |
Mean and 2SD
|
−0.5
|
0.8
|
1702.1
|
1.6
|
−0.1
|
3.3
|
−2.5
|
1.9
|
|
|
![[thin space (1/6-em)]](https://www.rsc.org/images/entities/char_2009.gif) |
BCR-2
|
B34F7 BCR-2 d1 |
0.1 |
2.2 |
1017.0 |
1.6 |
−0.3 |
1.4 |
−1.4 |
3.3 |
1.5 × 10−6 |
4.7 × 10−7 |
B33 F13 BCR-2 d2 |
−2.6 |
2.3 |
1015.8 |
1.8 |
−1.0 |
1.3 |
−1.9 |
3.0 |
5.4 × 10−7 |
5.3 × 10−7 |
B33 F14 BCR-2 d2 |
−2.7 |
2.2 |
1018.1 |
1.6 |
−0.6 |
1.4 |
−0.4 |
3.4 |
5.0 × 10−7 |
1.4 × 10−7 |
Mean and 2SD
|
−1.7
|
3.2
|
1017.0
|
2.3
|
−0.6
|
0.7
|
−1.2
|
1.5
|
|
|
![[thin space (1/6-em)]](https://www.rsc.org/images/entities/char_2009.gif) |
Pilbara titanite “Ln group”
|
B28F14 039ttn |
6.3 |
1.6 |
−383.2 |
1.2 |
7.8 |
1.1 |
−8.5 |
2.6 |
1.3 × 10−6 |
2.1 × 10−7 |
B28F16 116ttn |
5.3 |
2.3 |
−422.9 |
1.7 |
6.4 |
1.5 |
−5.1 |
3.5 |
1.2 × 10−6 |
2.1 × 10−7 |
B28F17 134ttn |
4.6 |
2.5 |
−388.6 |
1.9 |
5.5 |
1.7 |
−7.0 |
3.9 |
6.2 × 10−7 |
1.3 × 10−7 |
B28F18 167ttn |
11.0 |
1.6 |
−796.5 |
1.3 |
7.6 |
1.1 |
−8.5 |
2.6 |
6.3 × 10−6 |
1.2 × 10−7 |
B29F8 039ttn_2 |
6.5 |
1.8 |
−379.9 |
1.4 |
11.0 |
1.2 |
−11.5 |
2.7 |
1.7 × 10−6 |
1.7 × 10−6 |
B29F7 043ttn_2 |
3.6 |
1.6 |
1118.6 |
1.3 |
7.2 |
1.1 |
−9.8 |
2.5 |
2.5 × 10−6 |
3.3 × 10−7 |
B29F6 116ttn_2 |
6.7 |
2.4 |
−422.6 |
1.8 |
3.8 |
1.5 |
−7.3 |
3.5 |
1.8 × 10−6 |
1.8 × 10−7 |
B29F5 134ttn_2 |
2.3 |
3.1 |
−392.9 |
2.3 |
6.5 |
2.1 |
−5.1 |
4.9 |
1.7 × 10−6 |
−6.1 × 10−7 |
B29F4 167ttn_2 |
7.4 |
1.8 |
−799.6 |
1.4 |
8.7 |
1.2 |
−14.2 |
2.7 |
2.6 × 10−6 |
2.1 × 10−7 |
Mean and 2SD
|
6.0
|
5.0
|
|
|
7.2
|
4.0
|
−8.6
|
5.9
|
|
|
![[thin space (1/6-em)]](https://www.rsc.org/images/entities/char_2009.gif) |
Pilbara titanite “DGA group”
|
B33 F15 ttn043 d2 |
−4.0 |
2.2 |
2083.7 |
1.7 |
−1.5 |
1.4 |
1.7 |
3.2 |
7.4 × 10−8 |
1.4 × 10−5 |
B33 F16 ttn116 d2 |
−4.2 |
2.1 |
−218.5 |
1.6 |
−1.5 |
1.3 |
−2.6 |
3.0 |
6.1 × 10−6 |
4.5 × 10−7 |
B33 F17 ttn039 d2 |
−2.8 |
1.7 |
−360.7 |
1.3 |
−0.5 |
1.2 |
−2.8 |
2.5 |
−9.9 × 10−8 |
1.3 × 10−6 |
B33 F18 ttn167 d2 |
−3.6 |
2.0 |
−511.8 |
1.5 |
0.0 |
1.2 |
−3.1 |
2.8 |
7.4 × 10−6 |
2.0 × 10−7 |
B33F20 ttn134 d2 |
−3.2 |
1.6 |
−477.6 |
1.3 |
−0.8 |
1.1 |
−3.1 |
2.4 |
−2.3 × 10−7 |
4.0 × 10−6 |
Mean and 2SD
|
−3.5
|
1.2
|
|
|
−0.8
|
1.3
|
−2.0
|
4.1
|
|
|
For the geological reference materials, BHVO-2 and BCR-2, the two separate dissolutions of each rock were processed using different first-step columns – the primary cation exchange column and the primary DGA column. The BHVO-2 and BCR-2 analyses also yielded sub-ppm level Ce/Nd and Sm/Nd ratios, with average μ142Nd values of −0.5 ± 0.8 ppm and −1.7 ± 3.2 ppm, in good agreement with the published high-precision TIMS and MC-ICPMS data15,21,27 (Table 2). Our μ143Nd values of BHVO-2 and BCR-2 are +1702.1 ± 1.6 ppm and 1017.0 ± 2.3 ppm, respectively, which are compatible with the most precise reported values so far, +1702.6 ± 2.5 ppm (ref. 14) (“MLA method”, TIMS 4-step dynamic) and +1698.8 ± 2.3 ppm (ref. 8) (MC-ICPMS) for BHVO-2, and +1014.6 ± 6.2 ppm (ref. 17) (TIMS 3-step dynamic) for BCR-2. Collectively, these Nd isotope results for doped JNdi-1 and the geological reference materials further confirm the effectiveness of our two-stage column chemistry scheme.
3.3 Mass-independent isotope fractionation during column chemistry
Our study presents an overall long-term (over 6 month) μ142Nd and μ143Nd reproducibility (i.e., intermediate precision) of ±2.5 ppm and ±1.0 ppm (2SD, n = 20) for JNdi-1 standards (ESI Table S2†). Our precision is among the best with the current generation of instrumentation, which is 2–3 ppm for 142Nd/144Nd.15,18,21 Based on this level of precision, the sample μ142Nd values with the confidence interval outside of the ±3 ppm range would be considered as resolvable. However, potential isotopic fractionation during chemical separation that differs in mass dependence from the exponential dependence assumed during mass spectrometry, even if just a few ppm, could be significant and result in inaccurate data. Recent high-precision studies that measured multiple Nd isotopes (4-step dynamic TIMS and MC-ICPMS) have documented anomalous correlations among the relative abundance of Nd isotopes, and attributed it to the nuclear field shift effect during column chemistry, especially for slim columns using fine-grained (25–50 μm) Ln resin that is commonly used for Nd separation.14,20,21 The study of ref. 21 used a very long and slim column (80 cm length × 0.24 cm inner diameter) and found that the front and tail fractions of the Nd elution profile have an opposite nuclear field shift effect. Therefore, a non-ideal recovery that misses either fraction will produce inaccurate data.20,28 Although Garçon et al.14 used a much shorter column (6 cm × 0.4 cm) with > 80% recovery, isotopic fractionations of up to 8 ppm on 143Nd/144Nd were noticed, which is significant for ultrahigh-precision Nd analysis with a reproducibility (i.e., intermediate precision) of ±2–3 ppm.
The BHVO-2 samples processed using the Ln method in ref. 14 yielded positive μ142Nd (+4.0 ± 4.3 ppm) and μ143Nd values (+1704.6 ± 3.8 ppm). Although the results are within the precision of JNdi-1 measurement results, the mean μ142Nd value of the BHVO-2 population is statistically different.14 At face value, this difference would imply that a mantle reservoir formed very early in the solar system history was preserved and isolated for more than 4 billion years underneath Hawaii. However, positive correlations between the μ142Nd and μ143Nd values as well as μ145Nd and μ143Nd values are observed,14 which suggests that the positive μ142Nd may be a result of isotope fractionation during column chemistry. In comparison, our data determined using the DGA method yielded lower μ142Nd and μ143Nd values (−0.5 ± 0.8 ppm and +1702.1 ± 1.6 ppm) with no clear correlation (Fig. 4).
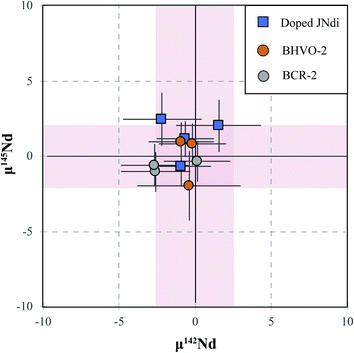 |
| Fig. 4
μ
142Nd vs. μ145Nd plot of geological reference materials and doped JNdi-1 standard solutions. Pink shades represent the 6 month reproducibility (i.e., intermediate precision, 2SD) of JNdi-1 standard measurements, μ142Nd of ±2.5 ppm and μ145Nd of ±2 ppm. | |
In order to further test the potential isotope fractionation using different column chemistry methods, we compare the Nd isotopic compositions of titanite separated from a suite of granite samples in the eastern Pilbara Craton using two different chemistry methods. One set of samples were processed using the popular fine-grain-size Ln column similar to ref. 14 (referred to as the “Ln group”, 6 × 0.24 cm, 25–50 μm); another set of the same samples were processed using our 2-stage DGA method (referred to as the “DGA group”). The recovery rates of both methods are between 90 and 98%. The procedure used for TIMS measurement is the same for both groups. The Ln group yielded consistent positive μ142Nd values mostly between +4 and +7 ppm with an extreme value at +11 ppm. The μ145Nd and μ148Nd values are +7.2 ± 4.0 ppm and −8.6 ± 5.9 ppm, respectively, also well outside the ∼±3 ppm range determined by repeated analyses of JNdi-1 standards. A positive correlation between μ142Nd and μ145Nd and a negative correlation between μ142Nd and μ148Nd were observed (Fig. 5). In contrast, the processed JNdi-1 standards in the DGA group yielded μ142,145,148Nd values that are unresolvable from the JNdi-1 values. The titanite samples processed using the DGA separation overlap in 145Nd/144Nd and 148Nd/144Nd with the JNdi-1 standard, but have 142Nd/144Nd consistently offset to lower values averaging μ142Nd = −3.5 ± 1.2 ppm, most likely reflecting a deficit in 142Nd/144Nd in their host whole rock – a sign of a progenitor that experienced LREE-enrichment during the lifetime of 146Sm. The 150Nd/144Nd ratios, unlike other multi-dynamic Nd isotopic ratios, were measured statically, and show an intermediate precision expressed as 2SD, over 6 months of JNdi-1 runs, of ±69 ppm, and are thus not sufficiently precise to resolve the sample from isotope standard values. The contrasting results of the same samples processed using different chemical separation methods suggest that the anomalous 142Nd/144Nd and other stable isotope ratios of the Ln group are likely artefacts caused by isotopic fractionation during the chemical separation process. The DGA method described in this study appears to be more reliable for minimizing the potential isotope fractionation during column chemistry, especially useful for resolving small 142Nd/144Nd anomalies (e.g., <5 ppm).
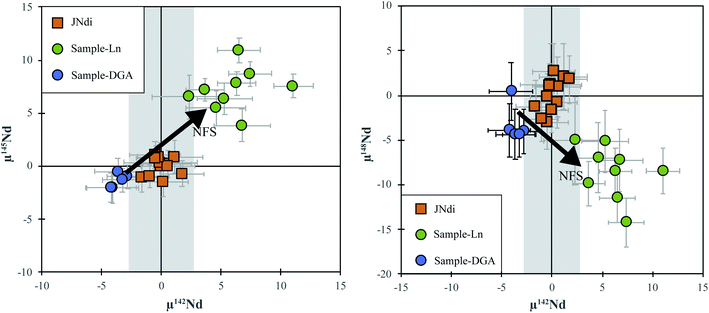 |
| Fig. 5
μ
142Nd vs. μ145,148Nd correlations showing the isotope fractionation effects caused by non-ideal column chemistry. NFS indicates the nuclear field shift (mass-independent isotope fractionation). Grey shades indicate the 6 month μ142Nd reproducibility (i.e., intermediate precision, ±2.5 ppm) for JNdi-1. | |
4. Conclusions
This study presents a two-step chemical separation scheme for ppm-level precision 142Nd/144Nd isotope ratio measurement. This column chemistry involves a first-step cation exchange column and a second-step tandem chromatographic extraction Ln-DGA column. This method effectively separates Ce, Pr and Sm from Nd, with a high Nd recovery of >98%, a low procedural blank, and a relatively short total process time of ∼14 hours. We report highly precise 142,143Nd/144Nd data for geological reference materials that are in good agreement with previous results, and a 6 month reproducibility (i.e., intermediate precision) of 2.5 ppm for 142Nd/144Nd. Compared with other commonly used column methods for Nd separation, the presented method has consistently high yields and enables effective removal of interferences with less effort, and most importantly has little mass-independent isotope fractionation effect. Samples analysed through the commonly used Ln method show clear nuclear field shift effects, while no detectable isotope fractionation was observed with our presented method. Therefore, we suggest that the chemical separation method presented here is effective for the accurate and precise determination of 142Nd/144Nd isotope composition and especially useful for the increasing effort in searching for isotope variations within ±5 ppm.
Conflicts of interest
There are no conflicts to declare.
Acknowledgements
This study was supported by a Carnegie Postdoctoral Fellowship to D. Wang. We thank M. Horan and M. Jordan for their support in the chemistry clean room, and T. Mock is thanked for maintaining the mass spectrometer at the peak condition at the Earth and Planets Laboratory, Carnegie Institution for Science. Two anonymous reviewers are thanked for their helpful comments that improved the clarity of the manuscript.
References
- A. Friedman, J. Milsted, D. Metta, D. Henderson, J. Lerner and A. Harkness,
et al., Alpha decay half lives of 148Gd 150Gd and 146Sm, Radiochim. Acta, 1966, 5(4), 192–194 CrossRef CAS.
- T. Tanaka, S. Togashi, H. Kamioka, H. Amakawa, H. Kagami and T. Hamamoto,
et al., JNdi-1: a neodymium isotopic reference in consistency with LaJolla neodymium, Chem. Geol., 2000, 168(3), 279–281 CrossRef.
- G. Caro, B. Bourdon, J.-L. Birck and S. Moorbath,
146Sm–142Nd evidence from Isua metamorphosed sediments for early differentiation of the Earth's mantle, Nature, 2003, 423(6938), 428–432 CrossRef CAS PubMed.
- M. Boyet and R. W. Carlson,
142Nd evidence for early (>4.53 Ga) global differentiation of the silicate Earth, Science, 2005, 309(5734), 576–581 CrossRef CAS PubMed.
- V. C. Bennett, A. D. Brandon and A. P. Nutman, Coupled 142Nd-143Nd Isotopic Evidence for Hadean Mantle Dynamics, Science, 2007, 318(5858), 1907–1910 CrossRef CAS PubMed.
- J. O'Neil, R. W. Carlson, D. Francis and R. K. Stevenson, Neodymium-142 Evidence for Hadean Mafic Crust, Science, 2008, 321(5897), 1828–1831 CrossRef PubMed.
- H. Rizo, M. Boyet, J. Blichert-Toft, J. O'Neil, M. T. Rosing and J.-L. Paquette, The elusive Hadean enriched reservoir revealed by 142 Nd deficits in Isua Archaean rocks, Nature, 2012, 491(7422), 96–100 CrossRef CAS PubMed.
- N. S. Saji, K. Larsen, D. Wielandt, M. Schiller, M. M. Costa and M. J. Whitehouse,
et al., Hadean geodynamics inferred from time-varying 142Nd/144Nd in the early Earth rock record, Geochem Persp Let, 2018, 43–48 CrossRef CAS PubMed.
- H. Rizo, M. Boyet, J. Blichert-Toft and M. T. Rosing, Early mantle dynamics inferred from 142Nd variations in Archean rocks from southwest Greenland, Earth Planet. Sci. Lett., 2013, 377–378, 324–335 CrossRef CAS.
- H. Rizo, R. J. Walker, R. W. Carlson, M. Touboul, M. F. Horan and I. S. Puchtel,
et al., Early Earth differentiation investigated through 142Nd, 182W, and highly siderophile element abundances in samples from Isua, Greenland, Geochim. Cosmochim. Acta, 2016, 175, 319–336 CrossRef CAS.
- A. Mundl, M. Touboul, M. G. Jackson, J. M. D. Day, M. D. Kurz and V. Lekic,
et al., Tungsten-182 heterogeneity in modern ocean island basalts, Science, 2017, 356(6333), 66–69 CrossRef CAS PubMed.
- M. F. Horan, R. W. Carlson, R. J. Walker, M. Jackson, M. Garçon and M. Norman, Tracking Hadean processes in modern basalts with 142-Neodymium, Earth Planet. Sci. Lett., 2018, 484, 184–191 CrossRef CAS.
- B. J. Peters, R. W. Carlson, J. M. D. Day and M. F. Horan, Hadean silicate differentiation preserved by anomalous 142Nd/144Nd ratios in the Réunion hotspot source, Nature, 2018, 555(7694), 89–93 CrossRef CAS PubMed.
- M. Garçon, M. Boyet, R. W. Carlson, M. F. Horan, D. Auclair and T. D. Mock, Factors influencing the precision and accuracy of Nd isotope measurements by thermal ionization mass spectrometry, Chem. Geol., 2018, 476, 493–514 CrossRef.
- E. Hyung and S. B. Jacobsen, The 142Nd/144Nd variations in mantle-derived rocks provide constraints on the stirring rate of the mantle from the Hadean to the present, PNAS, 2020, 117(26), 14738–14744 CrossRef CAS PubMed.
-
G. R. Choppin, Separation of the Lanthanides by Ion Exchange with Alpha-Hydroxy Isobutyric Acid, University of California Radiation Laboratory, 1956 Search PubMed.
- C.-F. Li, X.-C. Wang, Y.-L. Li, Z.-Y. Chu, J.-H. Guo and X.-H. Li, Ce–Nd separation by solid-phase micro-extraction
and its application to high-precision 142Nd/144Nd measurements using TIMS in geological materials, J. Anal. At. Spectrom., 2015, 30(4), 895–902 RSC.
- G. Caro, B. Bourdon, J.-L. Birck and S. Moorbath, High-precision 142Nd/144Nd measurements in terrestrial rocks: Constraints on the early differentiation of the Earth's mantle, Geochim. Cosmochim. Acta, 2006, 70(1), 164–191 CrossRef CAS.
- C. Pin and A. Gannoun, A triple tandem columns extraction chromatography method for isolation of highly purified neodymium prior to 143Nd/144Nd and 142Nd/144Nd isotope ratios determinations, J. Anal. At. Spectrom., 2019, 34(2), 310–318 RSC.
- N. S. Saji, D. Wielandt, J. C. Holst and M. Bizzarro, Solar system Nd isotope heterogeneity: Insights into nucleosynthetic components and protoplanetary disk evolution, Geochim. Cosmochim. Acta, 2020, 281, 135–148 CrossRef CAS.
- N. S. Saji, D. Wielandt, C. Paton and M. Bizzarro, Ultra-high-precision Nd-isotope measurements of geological materials by MC-ICPMS, J. Anal. At. Spectrom., 2016, 31(7), 1490–1504 RSC.
- Z.-Y. Chu, M.-J. Wang, C.-F. Li, Y.-H. Yang, J.-J. Xu and W. Wang,
et al., Separation of Nd from geological samples by a single TODGA resin column for high precision Nd isotope analysis as NdO+ by TIMS, J. Anal. At. Spectrom., 2019, 34(10), 2053–2060 RSC.
- A. Pourmand and N. Dauphas, Distribution coefficients of 60 elements on TODGA resin: Application to Ca, Lu, Hf, U and Th isotope geochemistry, Talanta, 2010, 81(3), 741–753 CrossRef CAS PubMed.
- S. Kagami and T. Yokoyama, Chemical separation of Nd from geological samples for chronological studies using 146Sm–142Nd and 147Sm–143Nd systematics, Anal. Chim. Acta, 2016, 937, 151–159 CrossRef CAS PubMed.
- D. Upadhyay, E. E. Scherer and K. Mezger, Fractionation and mixing of Nd isotopes during thermal ionization mass spectrometry: implications for high precision 142Nd/144Nd analyses, J. Anal. At. Spectrom., 2008, 23(4), 561–568 RSC.
- G. J. Wasserburg, S. B. Jacobsen, D. J. DePaolo, M. T. McCulloch and T. Wen, Precise determination of SmNd ratios, Sm and Nd isotopic abundances in standard solutions, Geochim. Cosmochim. Acta, 1981, 45(12), 2311–2323 CrossRef CAS.
- C. Burkhardt, L. E. Borg, G. A. Brennecka, Q. R. Shollenberger, N. Dauphas and T. Kleine, A nucleosynthetic origin for the Earth's anomalous 142Nd composition, Nature, 2016, 537(7620), 394–398 CrossRef CAS PubMed.
- S. Wakaki and T. Tanaka, Stable isotope analysis of Nd by double spike thermal ionization mass spectrometry, Int. J. Mass Spectrom., 2012, 323, 45–54 CrossRef.
Footnote |
† Electronic supplementary information (ESI) available. See DOI: 10.1039/d1ja00365h |
|
This journal is © The Royal Society of Chemistry 2022 |
Click here to see how this site uses Cookies. View our privacy policy here.