DOI:
10.1039/D1MA00493J
(Paper)
Mater. Adv., 2022,
3, 2056-2062
Conductive gold nanoparticle assembly linked through interactions between the radical cations of ethylene- and propylene-3,4-dioxythiophene mixed tetramer thiolate†
Received
5th June 2021
, Accepted 1st January 2022
First published on 5th January 2022
Abstract
A conjugated mixed tetramer consisting of ethylenedioxythiophene (EDOT (E)) and propylenedioxythiophene (ProDOT (P)) dimers (E2P2) with a methylthio end-capping group at the EDOT side was directly introduced via a thiolate linker on a gold nanoparticle (AuNP) surface covered with dodecylamine by a ligand exchange reaction. The molar fraction of the thiolate of E2P2 in the dodecylamine protecting group of AuNPs reached up to 0.35. Cyclic voltammetry of the AuNPs showed two couples of anodic and cathodic peaks due to the E2P2 moiety, suggesting that the radical cation of the E2P2 unit could be generated on the AuNPs. In practice, the formation of stable radical cations of E2P2 on the AuNPs was observed in dichloromethane by chemical oxidation with AgSbF6. The conductivity of the drop-cast film of the neutral AuNPs was moderate (∼10−4 S cm−1), while the conductivity after doping with iodine vapor was increased to ∼10−2 S cm−1. Similarly, the drop-cast film of the dichloromethane solution of the chemically oxidized AuNPs having an E2P2 radical cation moiety showed better conductivity than the iodine-doped film. Accordingly, the π-dimer between the E2P2 radical cation moieties on the neighboring AuNPs was considered to form an effective conduction path in the AuNP assembly.
Introduction
Gold nanoparticles (AuNPs) have attracted growing interest because of their unique functions arising from the particle size and properties of the surrounding groups on the surface.1 A wide range of applications in the fields of catalysis,2 electronics,3 photonics,4 sensing,5 and medicine6 have been investigated. Furthermore, the facile preparation and post-modification methods of surface-decorated AuNPs are optimized for excellent efficiency. Particularly, practical applications in stretchable conductors7 and electrodes in solution-processable transistors8 were demonstrated based on the high electrical conductivity of citrate- and phthalocyanine-capped AuNP assemblies, respectively. The advantages of nanoparticle-based conductors are their solution processability and intrinsic ability for self-organization due to their spherical structure.
Switching the electrical conductivity of AuNP assemblies by external stimuli is an intriguing challenge. To this end, various AuNPs modified with π-conjugated oligomers and polymers having direct connections via thiolate,9–15 phosphine,16 or N-heterocyclic carbene17 anchor groups were prepared. The conductivity switching behaviors derived from the changes in the effective conjugation path and length of the π-systems on the AuNPs have been shown. For example, a 25-fold enhancement of conductivity in a device using diarylethene-based AuNP assemblies was observed by photoirradiation.12 Diarylethenes are well-known photochromic materials.18 Ring closure at the dithienylethene moiety after irradiation results in a better conduction path between the AuNPs. In contrast, the conductivity of diphenylphosphinoterthiophene-capped AuNP assemblies showed an ∼103-fold enhancement after electrochemical oxidation.16 In this case, the direct linkage between AuNPs through sexithiophene was formed by the oxidative coupling reaction at the α-position of the terminal thiophene unit of the terthiophene moiety. Accordingly, the direct linkage of AuNPs with π-conjugated systems is considered to be effective for achieving higher conductivity. However, the formation of covalent bonds is an irreversible process that limits conductivity switching.
Another promising method to control the conductivity switching of AuNPs is the utilization of the face-to-face interaction between π-radical cations (π-dimer) as an emerging non-covalent interaction for self-assembly.19,20 The π-dimer is believed to form effective conduction paths in conductive, positively charged (p-doped) π-conjugated polymers.21,22 In particular, as models of p-doped states for the representative conductive polymers of polythiophenes, radical cation π-dimers of various oligothiophenes were intensively investigated23 and hence, they are considered to be one of the best candidates. Although most radical cations of oligothiophenes are prone to form covalently bonded dimers of the precursor oligomer in the condensed phase as previously utilized in the formation of the direct linkage between AuNPs,16 appropriately modified oligothiophenes with a reversible one-electron oxidation have the potential to construct AuNP assemblies with redox-switchable conductivity.
We are interested in the basic properties and their applications to the supramolecular chemistry of the π-dimers of conjugated oligomer radical cations.24–31 As such, AuNP 1 protected by thiolates of methylthio end-capped quaterthiophene partially annelated with bicyclo[2.2.2]octene units (Fig. 1) was prepared in our previous study.32 The conductivity of the drop-cast film of 1 was below the measurement limit (<10−6 S cm−1) of the voltage–current monitor and interdigitated array (IDA) electrode used, while more than 104-fold enhancement of conductivity was recorded after exposure of iodine vapor to the film. The desired π-dimer formation between the generated radical cations might lead to the formation of an effective conduction path. However, it could not be excluded that unwanted reactions such as the formation of other cationic states and/or bond formation at the β-position of the terminal thiophene ring also proceeded in the solid-state oxidation with I2 vapor. Thus, it was difficult to provide further assessment of the ability of the π-dimer linker as a molecular wire. In this study, we prepared AuNP 2 covered with thiolates of the methylthio end-capped mixed tetramer consisting of ethylenedioxythiophene (EDOT (E)) and propylenedioxythiophene (ProDOT (P)) dimers in addition to dodecylamine capping groups (Fig. 1). The radical cation state of the mixed tetramer (E2P2) unit on the AuNP surface was stable in dichloromethane. Conductive AuNP assemblies were formed in the cast film prepared from the solution.
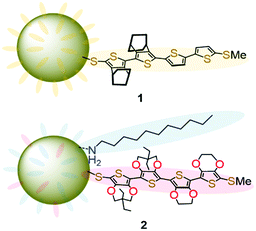 |
| Fig. 1 Schematic representations of AuNPs 1 and 2. | |
Results and discussion
Molecular design and synthesis
The electron-donating dioxy substituents in EDOT33,34 and ProDOT35,36 highly stabilized the radical cation state even with a relatively shorter oligomer length. Given that core-to-core (co)tunneling is considered to be the principal conduction mechanism of an AuNP assembly,37 the use of oligomers with moderate length would be ideal. However, the synthesis of even tetramers of EDOT (E4) without bulky end-capping groups is difficult owing to their poor solubility in common organic solvents. Alternatively, in the case of ProDOT, solubilizing side chains can be introduced in a symmetrical manner at the central carbon in the propylene constituent.38 However, in our previous studies, we have found that the methylthio-end-capped tetramer of ProDOT (P4) with a dihexyl side chain (3) does not have π-dimerization ability in the radical cationic state35 partly owing to the intermolecular steric repulsion between the side chains. In contrast, we have also found that the combined EDOT–ProDOT mixed tetramer 4a having the E2P2 structure with a methylthio end-capping group at both ends can form a radical cation π-dimer.28 Furthermore, significant exchange interactions between nitronyl nitroxide spin labels through the π-dimers of E2P2 radical cations were proved in a later study.30 This proposed that the ability of the π-dimer as a molecular wire is comparable or even superior to that of covalently linked conjugated oligomers. Thus, we chose thiolate of 4 as the surrounding group of AuNPs.
For the synthesis of the precursor thiol 4b, the reduction of thiocyanate 4c is a convenient process. Since we had already prepared the precursor 4d,30 we first attempted thiocyanation of 4d using potassium thiocyanide and bromine, as in the case of the previous synthesis of 1.32 However, the reaction presented a complex mixture (Scheme 1a). Probably, 4d suffered from undesired one-electron oxidation with Br2 (reduction potential +0.07 V vs. Fc/Fc+),39 because the E2P2 moiety should have a lower oxidation potential judging from that for 4a (−0.11 V vs. Fc/Fc+)28 due to multiple electron-donating oxy-substituents. Thus, we conducted thiocyanation of 4d with n-BuLi, zinc thiocyanate, and N-chlorosuccinimide (NCS)40 instead (Scheme 1b). After the thiocyanation, the 1H NMR signal of the terminal thiophene proton of 4d (δ 6.37 ppm)30 disappeared, while the characteristic 13C NMR signal of cyanide carbon was observed at 93.3 ppm. The yield (54%) was moderate, mainly because of the difficulty of the first lithiation step of the electron-rich E2P2 unit. Thus, some unreacted 4d was recovered under these basic conditions.
 |
| Scheme 1 Synthesis of 4c. | |
In the previous synthesis of AuNPs 1,32 the precursor thiol was prepared through the reduction of thiocyanate with lithium aluminum hydride (LAH). The product was tolerant to oxidation during the work-up procedure using hydrochloric acid and ether in air. In the presence of this thiol, the Brust method,41 which involved the reduction of tetrachloroauric(III) acid with sodium borohydride (NaBH4), was applied to produce 1. However, thiol 4b was observed to be air-sensitive and the standard work-up after the reaction of 4c with LAH produced a mixture which included the corresponding disulfide dimer based on the mass spectra and unidentified by-products (Scheme 2a). Therefore, we chose the ligand exchange reaction of AuNPs (∼2.5 nm particle size) capped with dodecylamine (DDA) (AuNPs–DDA)42 with in situ generated 4b under a nitrogen atmosphere (Scheme 2b). The reaction was conducted in toluene, and therefore, soluble tetrabutylammonium borohydride was used as the reducing agent instead of NaBH4. In this reaction, the rate of replacement of the DDA ligands with the thiolate of 4 seemed to be saturated in the middle stage. The molar fraction of the thiolate, including the remaining DDA on AuNPs after 24 h, reached up to ∼0.35, based on a rough estimation of the 1H NMR spectra (see Fig. S1 in the ESI†). However, the precise control of the reaction rate was difficult because of the air sensitivity of 4b, and the molar fraction was varied from 0.15 to 0.35. Hereafter, these AuNPs will be designated as 2. The 1H NMR signals of the oligothiophene moiety in 2 and the alkyl chains of DDA broadened mainly due to the restricted mobility of these moieties on the AuNP surface in the solution. AuNP 2 was observed to be stable in air. Accordingly, once the thiolate coordinated to the AuNP surface, the formation of the disulfide, as observed in the attempted synthesis of 4b, was suppressed.
 |
| Scheme 2 Synthesis of AuNPs 2. | |
Basic properties of AuNPs 2
The electronic absorption spectra of AuNPs 2 in dichloromethane are shown in Fig. 2 together with those of 4c and AuNPs–DDA for comparison. The absorption maximum of 4c was observed at 443 nm with the vibrational fine structure as observed in EDOT and ProDOT oligomers. Additionally, AuNPs–DDA absorbed up to 800 nm with a typical surface plasmon absorption (SPA) band around 530 nm. Since it is known that AuNPs with a diameter less than 2 nm lose the distinctive SPA band,43 the synthesized AuNPs–DDA was confirmed to have a particle size of more than 2 nm. In contrast, for 2, the vibrational fine structure of the maximum absorption band of the E2P2 moiety broadened with a 15 nm red-shift relative to that of 4c. In addition, although no apparent SPA band was detected, the absorption edge reached 800 nm, similar to that of AuNPs–DDA. In the case of 1 (average particle size: 4.2 nm), a similar red-shift and absorption edge reaching the near-IR region was also observed, whereas SPA was identified as a shoulder band.32 Collectively, the broad absorption maximum of 2 at 458 nm without shoulder peaks indicated that unreacted 4c and uncoordinated species involving the neutral E2P2 moiety, such as the disulfide dimer, were sufficiently washed off. Furthermore, no apparent SPA band for 2 was observed, suggesting that the particle size of 2 decreased upon the ligand exchange reaction.
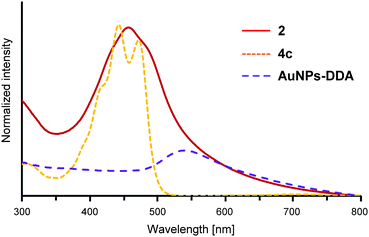 |
| Fig. 2 Electronic absorption spectra of 2, 4c, and AuNPs–DDA. | |
To characterize 2, transmission electron microscopy (TEM) was conducted using a thin carbon film grid. As shown in Fig. 3a (for particle size and distribution, see Fig. S2, ESI†), the average particle size was observed to be 1.6 ± 0.5 nm. Thus, the decrease in particle size upon the ligand exchange reaction was confirmed. This is in sharp contrast to the result that the same ligand exchange of 2.5 nm AuNPs–DDA with 9-(4-mercaptophenylethynyl)anthracene appeared to retain the particle size.42 The size decrease in 2 might be caused by the more bulky ProDOT unit with a diethyl side chain than the phenyl unit near the AuNP surface. The sterically demanding group near the AuNP surface, as utilized in the synthesis of gold nanoclusters,44 could enlarge the curvature of the particles by steric repulsion. Furthermore, in our previous study, AuNPs covered with methylthio end-capped-terthiophene thiolate without substituents at the β-position showed a self-assembled structure probably owing to the interactions between the methythio group and neighboring AuNPs (Fig. 3b).32 However, for AuNPs 1, such an interaction was restricted due to the presence of the bulky bicyclo[2.2.2]octene unit at the β-position of thiophene rings45 near the AuNP surface side. Similarly, no apparent self-assembled structure was observed in 2 as most particles were scattered. Thus, in the neutral state, the presence of the sterically demanding ProDOT units might also restrain the interparticle interaction between the outer methylthio group and Au atoms in the neighboring AuNPs.
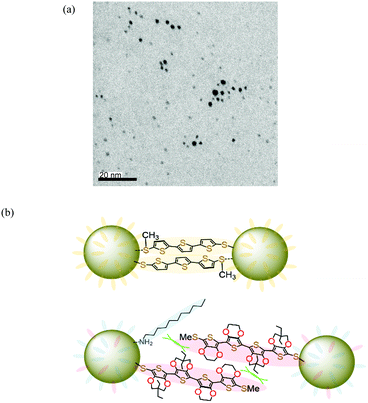 |
| Fig. 3 (a) A TEM image of AuNPs 2. (b) Schematic representations of the inter-particle interactions of AuNPs covered with methylthio end-capped terthiophene thiolate. In the case of 2, such an interaction would be restricted. | |
Cyclic voltammetry (CV) of 2 (Fig. 4a) in CH2Cl2 containing 0.1 M tetrabutylammonium hexafluorophosphate (TBAPF6) showed two couples of anodic and cathodic peaks. The averages of the first (−0.09 V (vs. Fc/Fc+)) and second (+0.20 V (vs. Fc/Fc+)) peaks (Epa1 = −0.06 V, Epc1 = −0.12 V; Epa2 = +0.23 V, Epc2 = +0.17 V) of 2 were slightly shifted to the positive potential side compared with those of 4a (Eox11/2 = −0.11 V, Eox21/2 = +0.05 V (vs. Fc/Fc+)28), suggesting that the observed CV wave is due to the oxidation process of E2P2 moiety. To predict the HOMO level of 2, DFT calculations of the single E2P2 thiolate on 12 (=3 × 4) Au atoms in a (111) arrangement and one Au adatom placed on a 2-fold hollow46,47 were conducted using the M06 method (LanL2DZ for Au and 6-31G(d) for other elements). The calculated HOMO was spread on the E2P2 moiety (Fig. 4b) and the level (−4.72 eV) was almost identical to that of 4a (−4.75 eV), supporting the interpretation of the results of CV. Furthermore, given the observation of anodic and cathodic couple at a low oxidation potential, the stable radical cation of the E2P2 moiety could be generated using a mild oxidizing agent.
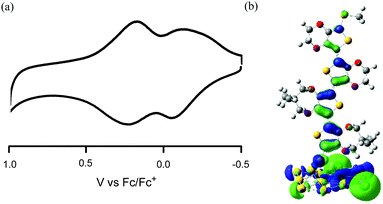 |
| Fig. 4 (a) CV of 2 in CH2Cl2 with 0.1 M TBAPF6. Scan rate: 0.1 V s−1. (b) HOMO of E2P2 thiolate of the Au13 cluster. | |
The chemical one-electron oxidation of the E2P2 moiety on AuNPs 2 in CH2Cl2 was performed by adding a solution of AgSbF6 until the absorption band due to the neutral E2P2 moiety disappeared. As shown in Fig. 5, two broad absorption bands appeared at 688 nm and ∼1140 nm owing to this oxidation reaction. These bands were similar to those of the radical cation 4a˙+ (717 nm, 1228 nm),28 and therefore could be assigned to the SOMO–LUMO and HOMO–SOMO transitions of the radical cation of the E2P2 moiety on the AuNPs. No apparent diagnostic absorption bands for the π-dimer formation28 between E2P2 moieties on the same AuNP surface was observed probably due to the repulsion between ProDOT units.
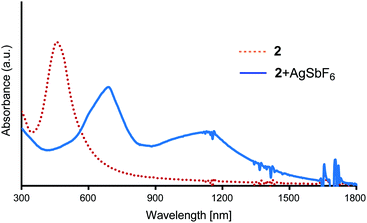 |
| Fig. 5 Electronic absorption spectra of 2 oxidized with AgSbF6. | |
To confirm the presence of the radical cation moiety, an ESR spectrum was obtained. The oxidized 2 in CH2Cl2 exhibited a signal with a g value of 2.0028 at room temperature (Fig. S3, ESI†). The M06 calculations of the one-electron oxidized state of the single E2P2 thiolate of Au13 (Fig. S4, ESI†) showed that the spin density is spread over the entire E2P2 and Au atoms, supporting the results of the UV-vis-NIR and ESR spectra.
A TEM image of the chemically oxidized 2 showed a self-assembled network structure over hundreds of nanometers (Fig. 6a) in contrast to the neutral 2 (Fig. 3a). The network seemed to be three-dimensionally linked; therefore, the average interparticle distance could not be determined. Nevertheless, in the enlarged image (Fig. 6b), some interparticle distances appeared to be ∼2 nm. The distances are comparable to that between the Au atom and the sulfur atom of the methylthio group in the calculated model as shown in Fig. S4 (ESI†). Collectively, these results suggest that the radical cation of the E2P2 moiety contributed to the enhancement of interparticle interactions, probably due to the radical cation π-dimer formation. However, the ratio of the π-dimerized E2P2 moiety compared to the free radical cation moiety on the AuNP surface was considered to be too small, leading to undetectable diagnostic absorption bands for the π-dimer formation.28 However, based on the conductivity of the chemically oxidized 2 film, an interaction between the radical cation of the E2P2 moiety formed effective conduction paths.
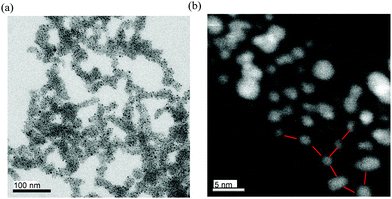 |
| Fig. 6 (a) TEM image (500 × 500 nm) of chemically oxidized AuNPs 2 and (b) the enlarged image (black/white inverted for clarity). The red bars corresponding to the 2 nm scale are added to indicate interparticle distance. | |
Conductivity of assembly of AuNPs 2 and their oxidized species
The conductivities of the drop-cast films of AuNPs 2 prepared from a dichloromethane solution were investigated. The IDA electrodes purchased were used for the measurements and, as reported previously,32 the saturated values for the film dried after adding a few drops of solution48 were considered. As described in the introduction, the conductivity of the drop-cast film of 1 was below the limit (<10−6 S cm−1) of the instrument used. Under the same measurement conditions, however, the conductivities of independently prepared two samples of neutral 2 with three repeated measurements ranged 1–2 × 10−4 S cm−1 (Fig. 7a). Given that the length of the remaining DDA ligands (∼1.5 nm) is shorter than that of E2P2 thiolate, 2 with a moderate molar fraction of DDA might allow closer proximity than 1, which facilitates core-to-core tunneling conduction.48
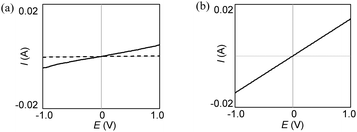 |
| Fig. 7 A current–potential response of (a) the 2 film before (dashed line) and after I2-doping (solid line) and (b) 2 + AgSbF6 on an IDA electrode. | |
The conductivities of the drop-cast films after doping using iodine vapor were enhanced, as observed in the case of AuNPs 1. The I2-doped films of 2 reached 5–9 × 10−3 S cm−1, respectively. These values are comparable to those of the iodine-doped film of 1 (3.7 × 10−2 S cm−1). Furthermore, the drop-cast films of 2 (independently prepared two samples) + AgSbF6 had the same order of conductivity (1–3 × 10−2 S cm−1) as shown in Fig. 7b. Comparing the iodine-doped and pre-oxidized films prepared from the same sample of 2, the pre-oxidized film had better conductivity. Therefore, it can be concluded that the interparticle interaction between the E2P2 radical cation moieties on the neighboring AuNPs formed an effective conduction path. Judging from the inter-particle distance shown in Fig. 6b, the formation of a π-dimer between the radical cation moiety was most likely.
Conclusions
In this study, we succeeded in the partial replacement of dodecylamine on AuNPs with methylthio end-capped EDOT and diethyl–ProDOT mixed tetramer E2P2 thiolate. The radical cation state of the E2P2 moiety on the AuNPs was stable in a dichloromethane solution at room temperature. The drop-cast film from this solution formed a self-assembled network structure over hundreds of nanometers. Based on the interparticle distance, a π-dimer between the radical cation moieties on the respective AuNPs were formed. To our knowledge, this is the first example of solution-processable AuNP assembly linked through the interaction between radical cations. The conductivity of the radical-cation-capped AuNP assembly was on the order of 10−2 S cm−1, which was slightly better than that of the iodine-doped AuNP film. Although the conductivity was moderate possibly due to the limitation derived from the small particle size,13 it can be concluded that the radical cation π-dimer was utilized to form effective conduction paths in the AuNP assembly.
Conflicts of interest
There are no conflicts to declare.
Acknowledgements
This work was supported by a Grant-in-Aid for Scientific Research (C) (No. 17K05977) from the Japan Society for the Promotion of Science (JSPS).
References
- M.-C. Daniel and D. Astruc, Gold nanoparticles: assembly, supramolecular chemistry, quantum-size-related properties, and applications toward biology, catalysis, and nanotechnology, Chem. Rev., 2004, 104, 293–346 CrossRef CAS PubMed.
- T. Ishida, T. Murayama, A. Taketoshi and M. Haruta, Importance of size and contact structure of gold nanoparticles for the genesis of unique catalytic processes, Chem. Rev., 2020, 120, 464–525 CrossRef CAS PubMed.
- Y. Ko, C. H. Kwon, S. W. Lee and J. Cho, Nanoparticle-based electrodes with high charge transfer efficiency through ligand exchange layer-by-layer assembly, Adv. Mater., 2020, 32, 1–26 Search PubMed.
- J. Olesiak-Banska, M. Waszkielewicz, P. Obstarczyk and M. Samoc, Two-photon absorption and photoluminescence of colloidal gold nanoparticles and nanoclusters, Chem. Soc. Rev., 2019, 48, 4087–4117 RSC.
- K. Saha, S. S. Agasti, C. Kim, X. Li and V. M. Rotello, Gold nanoparticles in chemical and biological sensing, Chem. Rev., 2012, 112, 2739–2779 CrossRef CAS PubMed.
- D. A. Giljohann, D. S. Seferos, W. L. Daniel, M. D. Massich, P. C. Patel and C. A. Mirkin, Gold nanoparticles for biology and medicine, Angew. Chem., Int. Ed., 2010, 49, 3280–3294 CrossRef CAS PubMed.
- Y. Kim, J. Zhu, B. Yeom, M. Di Prima, X. Su, J.-G. Kim, S. J. Yoo, C. Uher and N. a. Kotov, Stretchable nanoparticle conductors with self-organized conductive pathways, Nature, 2013, 500, 59–63 CrossRef CAS PubMed.
- T. Minari, Y. Kanehara, C. Liu, K. Sakamoto, T. Yasuda, A. Yaguchi, S. Tsukada, K. Kashizaki and M. Kanehara, Room-temperature printing of organic thin-film transistors with π-junction gold nanoparticles, Adv. Funct. Mater., 2014, 24, 4886–4892 CrossRef CAS.
- W. Huang, G. Masuda, S. Maeda, H. Tanaka and T. Ogawa, Molecular junctions composed of oligothiophene dithiol-bridged gold nanoparticles exhibiting photoresponsive properties, Chem. – Eur. J., 2005, 12, 607–619 CrossRef CAS PubMed.
- S. Taniguchi, M. Minamoto, M. M. Matsushita, T. Sugawara, Y. Kawada and D. Bethell, Electron transport in networks of gold nanoparticles connected by oligothiophene molecular wires, J. Mater. Chem., 2006, 16, 3459 RSC.
- M. Ikeda, N. Tanifuji, H. Yamaguchi, M. Irie and K. Matsuda, Photoswitching of conductance of diarylethene-Au nanoparticle network, Chem. Commun., 2007, 1355–1357 RSC.
- K. Matsuda, H. Yamaguchi, T. Sakano, M. Ikeda, N. Tanifuji and M. Irie, Conductance photoswitching of diarylethene-gold nanoparticle network induced by photochromic reaction, J. Phys. Chem. C, 2008, 112, 17005–17010 CrossRef CAS.
- G. Zotti, B. Vercelli and a. Berlin, Gold nanoparticles linked by pyrrole- and thiophene-based thiols. electrochemical, optical, and conductive properties, Chem. Mater., 2008, 20, 397–412 CrossRef CAS.
- M. Quintiliani, M. Bassetti, C. Pasquini, C. Battocchio, M. Rossi, F. Mura, R. Matassa, L. Fontana, M. V. Russo and I. Fratoddi, Network assembly of gold nanoparticles linked through fluorenyl dithiol bridges, J. Mater. Chem. C, 2014, 2, 2517–2527 RSC.
- T. Toyama, K. Higashiguchi, T. Nakamura, H. Yamaguchi, E. Kusaka and K. Matsuda, Photoswitching of conductance of diarylethene-gold nanoparticle network based on the alteration of π-conjugation, J. Phys. Chem. Lett., 2016, 7, 2113–2118 CrossRef CAS PubMed.
- B. C. Sih, A. Teichert and M. O. Wolf, Electrodeposition of oligothiophene-linked gold nanoparticle films, Chem. Mater., 2004, 16, 2712–2718 CrossRef CAS.
- N. Sun, S. Zhang, F. Simon, A. M. Steiner, J. Schubert, Y. Du, Z. Qiao, A. Fery and F. Lissel, Poly(3-hexylthiophene)s functionalized with N-heterocyclic carbenes as robust and conductive ligands for the stabilization of gold nanoparticles, Angew. Chem., Int. Ed., 2021, 60, 3912–3917 CrossRef CAS PubMed.
- M. Irie, T. Fukaminato, K. Matsuda and S. Kobatake, Photochromism of diarylethene molecules and crystals: memories, switches, and actuators, Chem. Rev., 2014, 114, 12174–12277 CrossRef CAS PubMed.
- T. Nishinaga and K. Komatsu, Persistent π radical cations: self-association and its steric control in the condensed phase, Org. Biomol. Chem., 2005, 3, 561–569 RSC.
- D.-W. Zhang, J. Tian, L. Chen, L. Zhang and Z.-T. Li, Dimerization of conjugated radical cations: an emerging non-covalent interaction for self-assembly, Chem. – Asian J., 2015, 10, 56–68 CrossRef CAS PubMed.
- L. L. Miller and K. R. Mann, π-Dimers and π-stacks in solution and in conducting polymers, Acc. Chem. Res., 1996, 29, 417–423 CrossRef CAS.
- D. D. Graf, R. G. Duan, J. P. Campbell, L. L. Miller and K. R. Mann, From monomers to π-stacks. a comprehensive study of the structure and properties of monomeric, π-dimerized, and π-stacked forms of the cation radical of 3′,4′-dibutyl-2,5′′-diphenyl-2,2′:5′,2′′-terthiophene, J. Am. Chem. Soc., 1997, 119, 5888–5899 CrossRef CAS.
-
T. Nishinaga, in Organic Redox Systems, ed. T. Nishinaga, Wiley, 2016, pp. 383–410 Search PubMed.
- D. Yamazaki, T. Nishinaga, N. Tanino and K. Komatsu, Terthiophene radical cations end-capped by bicyclo[2.2.2]octene units: formation of bent π-dimers mutually attracted at the central position, J. Am. Chem. Soc., 2006, 128, 14470–14471 CrossRef CAS PubMed.
- M. Tateno, M. Takase, M. Iyoda, K. Komatsu and T. Nishinaga, Steric control in the π-dimerization of oligothiophene radical cations annelated with bicyclo[2.2.2]octene units, Chem. – Eur. J., 2013, 19, 5457–5467 CrossRef CAS PubMed.
- T. Nishinaga, T. Kageyama, M. Koizumi, K. Ando, M. Takase and M. Iyoda, Effect of substituents on the structure, stability, and π-dimerization of dithienylpyrrole radical cations, J. Org. Chem., 2013, 78, 9205–9213 CrossRef CAS PubMed.
- M. Hasegawa, K. Kobayakawa, H. Matsuzawa, T. Nishinaga, T. Hirose, K. Sako and Y. Mazaki, Macrocyclic oligothiophene with stereogenic [2.2]paracyclophane scaffolds: chiroptical properties from π-transannular interactions, Chem. – Eur. J., 2017, 23, 3267–3271 CrossRef CAS PubMed.
- T. Nishinaga and Y. Sotome, Stable radical cations and their π-dimers prepared from ethylene- and propylene-3,4-dioxythiophene co-oligomers: combined experimental and theoretical investigations, J. Org. Chem., 2017, 82, 7245–7253 CrossRef CAS PubMed.
- T. Akahane, M. Takase, Y. Mazaki and T. Nishinaga, π-Dimerization ability of conjugated oligomer dication diradicaloids composed of dithienylpyrrole and benzodithiophene units, Heteroat. Chem., 2018, 29, 1–8 CrossRef.
- T. Nishinaga, Y. Kanzaki, D. Shiomi, K. Matsuda, S. Suzuki and K. Okada, Radical cation π-dimers of conjugated oligomers as molecular wires: an analysis based on nitronyl nitroxide spin labels, Chem. – Eur. J., 2018, 24, 11717–11728 CrossRef CAS PubMed.
- T. Fujiwara, A. Muranaka, T. Nishinaga, S. Aoyagi, N. Kobayashi, M. Uchiyama, H. Otani and M. Iyoda, Preparation, spectroscopic characterization and theoretical study of a three-dimensional conjugated 70 π-electron thiophene 6-mer radical cation π-dimer, J. Am. Chem. Soc., 2020, 142, 5933–5937 CrossRef CAS PubMed.
- M. Tateno, M. Takase and T. Nishinaga, Synthesis and conductive properties of gold nanoparticles protected by partially bicyclo[2.2.2]octene-annelated and methylthio end-capped oligothiophene thiolates, Chem. Mater., 2014, 26, 3804–3810 CrossRef CAS.
- J. J. Apperloo, L. B. Groenendaal, H. Verheyen, M. Jayakannan, R. a J. Janssen, A. Dkhissi, D. Beljonne, R. Lazzaroni and J.-L. Brédas, Optical and redox properties of a series of 3,4-ethylenedioxythiophene oligomers, Chem. – Eur. J., 2002, 8, 2384–2396 CrossRef CAS.
- P. M. Burrezo, B. Pelado, R. P. Ortiz, P. De La Cruz, J. T. L. Navarrete, F. Langa and J. Casado, Robust ethylenedioxythiophene-vinylene oligomers from fragile thiophene-vinylene cores: Synthesis and optical, chemical and electrochemical properties of multicharged shapes, Chem. – Eur. J., 2015, 21, 1713–1725 CrossRef PubMed.
- C. Lin, T. Endo, M. Takase, M. Iyoda and T. Nishinaga, Structural, optical, and electronic properties of a series of 3,4-propylenedioxythiophene oligomers in neutral and various oxidation states, J. Am. Chem. Soc., 2011, 133, 11339–11350 CrossRef CAS PubMed.
- N. B. Teran and J. R. Reynolds, Discrete donor-acceptor conjugated systems in neutral and oxidized states: implications toward molecular design for high contrast electrochromics, Chem. Mater., 2017, 29, 1290–1301 CrossRef CAS.
- A. Zabet-Khosousi and A.-A. Dhirani, Charge transport in nanoparticle assemblies, Chem. Rev., 2008, 108, 4072–4124 CrossRef CAS PubMed.
- P. M. Beaujuge and J. R. Reynolds, Color control in π-conjugated organic polymers for use in electrochromic devices, Chem. Rev., 2010, 110, 268–320 CrossRef CAS PubMed.
- N. G. Connelly and W. E. Geiger, Chemical redox agents for organometallic chemistry, Chem. Rev., 1996, 96, 877–910 CrossRef CAS PubMed.
- K. Takagi, H. Takachi and K. Sasaki, Syntheses of organic n,n-dialkyldithiocarbamates or organic thiocyanates from organozincs and corresponding thio-anions via the inversion of electronic reactivity of the anions with NCS-oxidation, J. Org. Chem., 1995, 60, 6552–6556 CrossRef CAS.
- M. Brust, M. Walker, D. Bethell, D. J. Schiffrin and R. Whyman, Synthesis of thiol-derivatised gold nanoparticles in a two-phase liquid-liquid system, J. Chem. Soc., Chem. Commun., 1994, 801–802 RSC.
- T. Zdobinsky, P. Sankar Maiti and R. Klajn, Support curvature and conformational freedom control chemical reactivity of immobilized species, J. Am. Chem. Soc., 2014, 136, 2711–2714 CrossRef PubMed.
- Y. G. Kim, S. K. Oh and R. M. Crooks, Preparation and characterization of 1-2 nm dendrimer-encapsulated gold nanoparticles having very narrow size distributions, Chem. Mater., 2004, 16, 167–172 CrossRef CAS.
- R. Jin, Quantum sized, thiolate-protected gold nanoclusters, Nanoscale, 2010, 2, 343–362 RSC.
- T. Nishinaga, A. Wakamiya, D. Yamazaki and K. Komatsu, Crystal structures and spectroscopic characterization of radical cations and dications of oligothiophenes stabilized by annelation with bicyclo[2.2.2]octene units: sterically segregated cationic oligothiophenes, J. Am. Chem. Soc., 2004, 126, 3163–3174 CrossRef CAS PubMed.
- E. Pensa, E. Cortés, G. Corthey, P. Carro, C. Vericat, M. H. Fonticelli, G. Benítez, A. a. Rubert and R. C. Salvarezza, The chemistry of the sulfur-gold interface: in search of a unified model, Acc. Chem. Res., 2012, 45, 1183–1192 CrossRef CAS PubMed.
- P. D. Jadzinsky, G. Calero, C. J. Ackerson, D. a. Bushnell and R. D. Kornberg, Structure of a thiol monolayer-protected gold nanoparticle at 1.1 A resolution, Science, 2007, 318, 430–433 CrossRef CAS PubMed.
- W. P. Wuelfing, S. J. Green, J. J. Pietron, D. E. Cliffel and R. W. Murray, Electronic conductivity of solid-state, mixed-valent, monolayer-protected au clusters, J. Am. Chem. Soc., 2000, 122, 11465–11472 CrossRef CAS.
Footnote |
† Electronic supplementary information (ESI) available: General methods, synthesis, computational methods, 1H and 13C NMR spectra, Fig. S1–S4, and Cartesian coordinates of the optimized structures of all calculated molecules. See DOI: 10.1039/d1ma00493j |
|
This journal is © The Royal Society of Chemistry 2022 |
Click here to see how this site uses Cookies. View our privacy policy here.