DOI:
10.1039/D1MA00646K
(Paper)
Mater. Adv., 2022,
3, 1191-1199
H2O2-replenishable and GSH-depletive ROS ‘bomb’ for self-enhanced chemodynamic therapy†
Received
26th July 2021
, Accepted 21st November 2021
First published on 23rd November 2021
Abstract
Chemodynamic therapy (CDT) is an emerging strategy of tumor therapy that utilizes the Fenton reagent to kill tumor cells by disproportionation of H2O2 into hydroxyl radical (˙OH). However, insufficient endogenous H2O2 confines the antitumor efficacy of CDT. Additionally, the overexpressed glutathione (GSH) exhibits a potent scavenging effect on cytotoxic ˙OH, which further diminishes the efficacy of CDT. Though tremendous efforts have been done, engineering CDT agents with efficient and specific H2O2 self-supplying and GSH-depletion is promising but remains a great challenge. Herein, Fe3+–chelated CaO2 nanoparticles (CaO2–Fe NPs) are constructed as ROS ‘bomb’. In the tumor microenvironment, CaO2–Fe NPs can release Fe2+ by the reduction of GSH, and the remaining CaO2 reacts with H+ to selectively generate H2O2. The generated H2O2 can produce ˙OH under the catalysis of Fe2+ through the Fenton reaction, and re-oxidation from Fe2+ to Fe3+ endowing a long-lasting GSH-depletion, resulting in an improved CDT. These CaO2–Fe NPs supply H2O2 and exhaust GSH simultaneously to achieve a self-enhanced CDT, and paves an emerging strategy to enhance the therapeutic efficacy of CDT by combining H2O2-replenishable and GSH-depletive together and realizing a self-enhanced Fenton reaction cycle.
Introduction
Reactive oxygen species (ROS) based tumor therapy is an emerging therapeutic strategy to effectively induce tumor-cell apoptosis.1–3 Among various ROS, cytotoxic hydroxyl radical (˙OH), which could be generated by H2O2via the Fenton reaction in an acidic environment is the most destructive and commonly applied type, which is defined as chemodynamic therapy (CDT).4–7 Benefiting from the overproduction of H2O2 in tumors (100 μM–1 mM) than normal tissues, and the mild acidity of the tumor microenvironment (TME), CDT is regarded as a promising method for selective-tumor therapy with the help of catalysis by ferrous ions (Fe2+), manganese ions (Mn2+), or cuprous ions (Cu+).8–11 However, some works recently suggested that the endogenous H2O2 in the tumor site is still insufficient to support effective CDT, which restricts the clinical application.12–15 Therefore, the introduction of the H2O2-replenishable agents should be taken into consideration.
A few signs of progress have been made to increase the intratumoral H2O2 concentration by applying natural bio-enzymes such as glucose oxidase (GOx), nicotinamide adenine dinucleotide phosphate oxidase (NOX), and superoxide dismutase (SOD).16–18 Nevertheless, these natural enzyme-based H2O2 supplements suffer from some potential issues, such as instability of biological activity, high cost, and reliance on the exogenous H2O2-precursors such as glucose, superoxide anion (O2˙−) or O2.19,20 Encouragingly, it has been reported that inexpensive calcium peroxide (CaO2) can steadily liberate a substantial amount of H2O2 under acidic conditions due to the presence of peroxy bond (–O–O–), and the production of H2O2 based on CaO2 is independent of additional precursors.21–25 Thus, based on the acidic environment of the tumor, CaO2 is a practicable H2O2-replenishable ‘bomb’ for CDT to efficiently accumulate H2O2 with tumor specificity.
Conceivably, if ˙OH generated is eliminated by ROS scavengers such as glutathione (GSH), it is worth nothing. For this reason, efficient ROS generation in the tumor runs into the bottleneck due to elevated GSH level intratumorally (up to 10 mM) compared with that in normal tissue.14,26–28 This problem could be potentially solved by employing some transition metal ions with variable valences, such as ions of iron, copper, and manganese, as recyclable GSH consumers. The transformation from a high-valence ion to its low-valence form reduces the concentration of GSH, and the resulting low-valence ion is a good catalyst for the Fenton reaction.29–31 Noteworthy that during the Fenton reaction, the low-valence ion is re-oxidized to its high-valence form, giving a sustained depletion of GSH and release of ˙OH in GSH and H2O2-rich area.
Herein, a tumor-selective self-enhanced CDT ‘bomb’ is designed by using ferric ions (Fe3+)–chelated CaO2 nanoparticles (CaO2–Fe NPs). As elucidated in Fig. 1, under the tumor microenvironment, CaO2 NPs reacted with specific H+ to form H2O2in situ as ROS ‘bomb’, and the chelated Fe3+ is reduced to Fe2+ by high-leveled GSH as the trigger. Subsequently, the Fenton reaction is activated by the generated H2O2 and Fe2+. Finally, ˙OH is produced for tumor therapy. Furthermore, the consumption of GSH enhances the CDT efficiency, and the re-oxidation of Fe2+ to Fe3+ endowing a long-lasting GSH-depletion. As a result, CaO2–Fe NPs are able to selectively generate amounts of ROS to induce apoptosis of tumor cells with low systemic toxicity both in vitro and in vivo. These CaO2–Fe NPs are good candidates for constructing ROS ‘bomb’ with endogenous replenishment of H2O2 and depletion of GSH, providing a novel strategy for improving tumor-selective CDT.
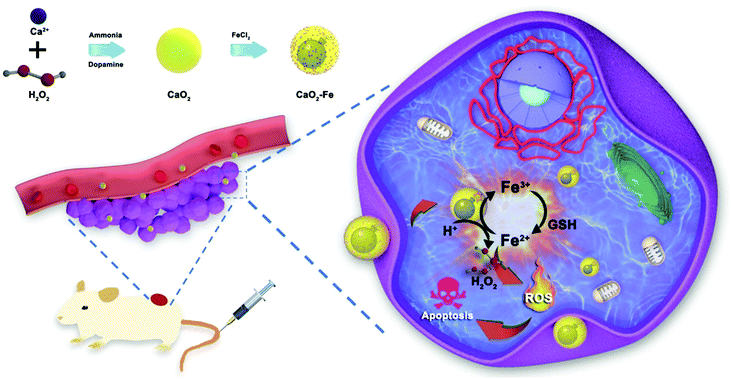 |
| Fig. 1 The schematic illustration of CaO2–Fe NPs as H2O2-replenishable and GSH-depletive ROS ‘bomb’ for self-enhanced CDT of the tumor. | |
Results and discussion
To obtain CaO2–Fe NPs, CaO2 NPs were first synthesized via a modified dopamine-assisted method, followed by mixing ferrous chloride to load iron ions.21,32,33 The chelated iron contents were adjusted by different feeding ratios between CaO2 and ferrous chloride (mass ratio). As shown in Table S1 (ESI†), contents of the chelated iron within CaO2-Fe NPs were gradually improved with the increase of the feeding ratio. However, CaO2–Fe NPs would not be formed when the feeding ratios were higher than 2
:
1. Thus, feeding ratios (4
:
1) were chosen to synthesize CaO2–Fe NPs. The morphology of CaO2–Fe NPs remained unchanged compared with the original CaO2 NPs, and their diameters increased from 91 nm to 122 nm (Fig. 2a and Fig. S1, S2, ESI†). Subsequently, as confirmed using X-ray diffraction (XRD), the introduction of iron ions could not influence the phase of CaO2 NPs (Fig. 2b). Strong and homogeneous iron signals were then observed from energy-dispersive X-ray spectroscopy (EDS) and EDS mapping, demonstrating the efficient binding of iron ions within CaO2 NPs (Fig. 2c and Fig. S3, ESI†). To explore the valence state of chelated iron ions, X-ray photoelectron spectrometry (XPS) was applied. The central peak at ∼710.0 eV (Fe 2p3/2) and the shakeup satellite peak at ∼724.0 eV (Fe 2p1/2) demonstrated that Fe2+ was transformed into Fe3+, which might be oxidized by CaO2 (Fig. 2d and e). The photoelectron peak at 532.5 eV of O 1s could be assigned to O–O, indicating the presence of peroxo groups (Fig. 2f).24
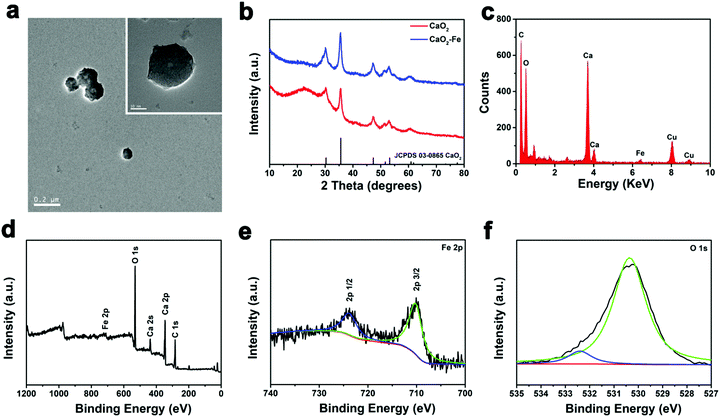 |
| Fig. 2 (a) TEM image of CaO2–Fe nanoparticles (inset: an image at a higher magnification of CaO2–Fe NPs). (b) XRD pattern of CaO2 and CaO2–Fe NPs. (c) EDS spectrum of CaO2–Fe NPs. (d) Survey XPS spectra of CaO2–Fe NPs. (e) High-resolution Fe 2p XPS spectra of CaO2–Fe NPs. (f) High-resolution O 1s XPS spectra of CaO2–Fe NPs. | |
Considering the significance of H2O2 for CDT, the H2O2 generation ability of CaO2–Fe NPs was investigated using potassium permanganate (KMnO4) as the indicator. As shown in Fig. 3a, the color of permanganate (MnO4−) disappeared after adding CaO2–Fe NPs to the acidic solution, suggesting the reduction of MnO4− to colorless Mn2+ by the generated H2O2. The dissociation of CaO2–Fe NPs in acidic solution further verified the acid-activated H2O2 generation (Fig. S4, ESI†). In comparison, less H2O2 was generated from CaO2–Fe NPs in a neutral environment (Fig. S5, ESI†). CaO2–Fe NPs could maintain long-term stability in a neutral environment (Fig. S6, ESI†). It indicated that CaO2–Fe NPs were good candidates for H2O2-replenishment in the acidic environment. These H2O2 suppliers could further release ˙OH induced by the Fenton reaction.
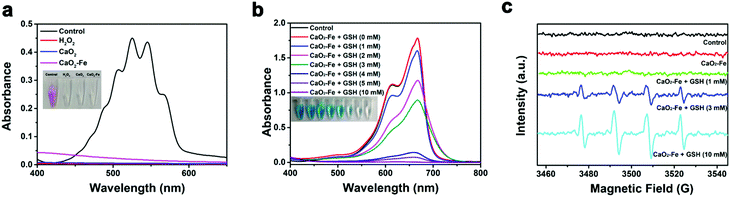 |
| Fig. 3 (a) UV-Vis absorption spectra and photo (inset) of KMnO4 after treating with H2O2, CaO2 NPs, and CaO2–Fe NPs in an acidic environment. (b) UV-vis absorption spectra and photo (inset) of MB after degradation by CaO2–Fe NPs treated with different amounts of GSH at pH 5.4. (c) ESR spectra of CaO2–Fe NPs treated with different amounts of GSH at pH 5.4 (5,5-dimethyl-1-pyrroline N-oxide (DMPO) as the spin trap). | |
To evaluate the ROS triggered by CaO2–Fe NPs, methylene blue (MB) was selected as the indicator. As can be seen in Fig. 3b and Fig. S7, S8 (ESI†), GSH is essential for ROS generation based on CaO2–Fe NPs, due to the generation of Fenton-catalytic Fe2+ by the reduction of GSH. It is noteworthy that a high level of GSH was adverse for ROS generation in most reported cases due to the strong scavenging effect of GSH on ROS.14,26 While CaO2–Fe NPs exhibited an excellent ROS releasing capacity even when the concentration of GSH was at 10 mM, with the MB degradation efficiency appeared to be 99%. This phenomenon could be ascribed to the continuous depletion of GSH under the Fenton reaction cycle based on CaO2–Fe NPs (Fig. S9, ESI†). During the GSH depletion and Fenton reaction cycle, Fe3+ was indispensable. In comparison with bare CaO2 NPs without Fe3+ chelated, CaO2–Fe NPs showed enhanced degradation of MB (Fig. S10, ESI†). Moreover, the ROS generation ability of CaO2–Fe NPs was increased with the improvement of the chelated iron content (Fig. S11, ESI†). In addition, CaO2–Fe NPs also showed a pH-dependent ROS due to the reliance on the generation of acidity of H2O2. These CaO2–Fe NPs caused an apparent color degradation of MB under acidic conditions (pH 5.4) with the the assistance of GSH, but no significant change was observed under neutral conditions (pH 7.4) (Fig. S12, ESI†). The type of ROS produced by CaO2–Fe NPs was further verified by the electron paramagnetic resonance (EPR) spin-trapping method. As shown in Fig. 3c, a characteristic 1
:
2
:
2
:
1 signal was obtained, indicating that the produced ROS by CaO2–Fe NPs was ˙OH. These results suggested that CaO2–Fe NPs were promising candidates for pH/GSH dual stimuli-activated CDT agents by H2O2 self-supplying and GSH-depletion.
Encouraged by the efficient production of ˙OH via CaO2–Fe NPs with the assistance of GSH and H+, in vitro ˙OH generation was investigated due to the higher intracellular GSH concentration and lower pH value in tumor cells. By employing 2,7-dichlorofluorescin diacetate (DCFH-DA) as the ˙OH indicator, fluorescence imaging was carried out on 4T1 cells, which showed that the fluorescence signal of CaO2–Fe NPs was dosage-dependent (Fig. S13, ESI†). Compared with CaO2 NPs and FeCl3 at the same dosage, CaO2–Fe NPs exhibited significantly stronger green fluorescence, indicating the self-enhanced ˙OH was generated from CaO2–Fe NPs in tumor cells (Fig. 4a and Fig. S14, ESI†). Considering the therapeutic effect of ˙OH, cell viability was then investigated by standard methyl thiazolyl tetrazolium (MTT) assay. As shown in Fig. 4b, CaO2–Fe NPs induced greater cell death by increasing concentrations, and the cytotoxic effect of the CaO2–Fe NPs treated group was greater than that of FeCl3 and CaO2 NPs at the same concentration. Results from live/dead cell staining assay further confirmed these results, which revealed that only a small number of 4T1 cells remained viable after treatment with CaO2–Fe NPs, while only a few cells were dead after treatment with FeCl3 and CaO2 NPs for 24 h (Fig. 4c and Fig. S15, ESI†).
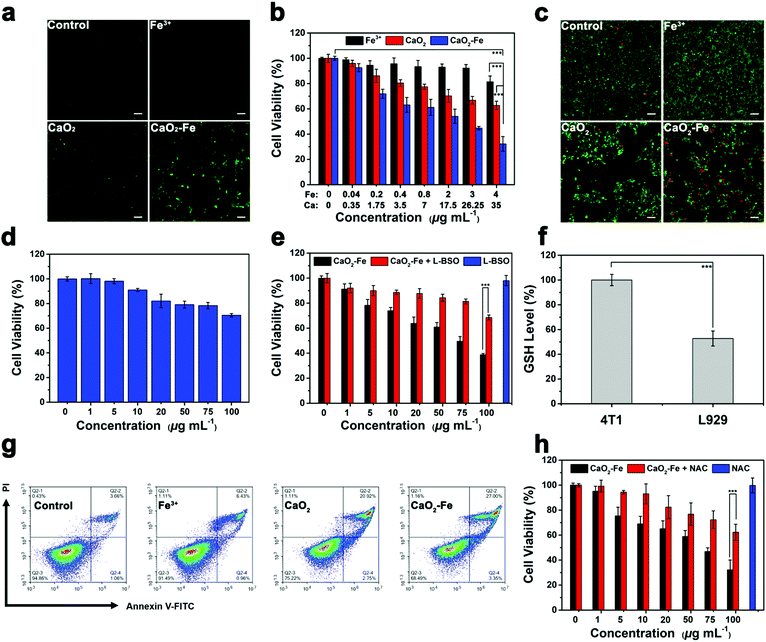 |
| Fig. 4 (a) Fluorescence images of DCFH-DA stained 4T1 cells after exposure to FeCl3, CaO2 NPs, and CaO2–Fe NPs for 4 h. The scale bar represents 100 μm. (b) Viability of 4T1 cells after 24 h of incubation with FeCl3, CaO2 NPs, and CaO2–Fe NPs. (n = 6, mean ± s.d., ***p < 0.001) (c) Fluorescence images of Calcein AM (green, live cells) and PI (red, dead cells) costained 4T1 cells after incubation with FeCl3, CaO2 NPs, and CaO2–Fe NPs for 24 h. The scale bar represents 100 μm. (d) Viability of L929 cells after 24 h of incubation with CaO2–Fe NPs. (n = 6, mean ± s.d.) (e) Viability of 4T1 cells after 24 h of incubation with CaO2–Fe NPs plus or without L-BSO. (n = 6, mean ± s.d., ***p < 0.001) (f) Intracellular GSH levels of 4T1 cells and L929 cells. (n = 3, mean ± s.d., ***p < 0.001) (g) Flow cytometry analysis of 4T1 cells treated with FeCl3, CaO2 NPs, and CaO2–Fe NPs for 24 h. (h) Viability of 4T1 cells after 24 h of incubation with CaO2–Fe NPs plus or without NAC. (n = 6, mean ± s.d., ***p < 0.001). | |
Interestingly, the cell inhibition effect from CaO2–Fe NPs was tumor cell-selective toxicity, which presented relatively low cytotoxicity toward normal cells (Fig. 4d and Fig. S16, ESI†). This phenomenon could be ascribed to the reliance of ˙OH generation on GSH concentration (Fig. 4e and Fig. S17, ESI†). After treating with CaO2–Fe NPs, the intracellular GSH level was decreased, and the cell viability was reversed by down-regulating GSH by using L-buthionine sulfoximine (L-BSO) as the GSH inhibitor. In addition, GSH concentration within normal cells (L929) was much lower than cancerous cells (4T1) (Fig. 4f). As a result, reduced GSH could hardly trigger the generation of enough ˙OH on normal cells (Fig. S18, ESI†), and finally suppressed the side effect.
Flow cytometry was further used to investigate the type of cell death using the annexin V-FITC/PI detection kit. As shown in Fig. 4g, cell death induced by all groups was apoptosis, and the CaO2–Fe NPs treated group had higher ratios of apoptotic cells (27%) than other groups. As ROS-mediated cell killing is regarded as the major pathway for apoptosis, the influence of N-acetyl-cysteine (NAC), a kind of ROS scavenger, on cell viability was then investigated. With the addition of NAC, cell apoptosis induced by CaO2–Fe NPs was obviously reversed, indicating the cell inhibition was originating from the production of ROS in tumor cells (Fig. 4h). All these results suggested that CaO2–Fe NPs was a GSH-enhanced CDT ‘bomb’ with self-supplied H2O2 to induce tumor cell apoptosis efficiently and selectively by ˙OH.
Tumor growth inhibition experiment was next performed by intravenous (i.v.) administration, inspired by outstanding treatment outcome of CaO2–Fe NPs in vitro. In vivo biodistribution of CaO2–Fe NPs was initially evaluated by labelling NIR dye (IR-783). As shown in Fig. S19 (ESI†), the CaO2–Fe NPs could be accumulated in tumor tissue via the EPR effect, indicating that CaO2–Fe NPs could serve as the ROS ‘bomb’ for self-enhanced chemodynamic therapy. The half-life of CaO2–Fe NPs was 1.17 ± 0.45 h (Fig. S20, ESI†). Mice treated with saline (as the control group), FeCl3, and CaO2 NPs showed rapid tumor growth, while the size of tumors in CaO2–Fe NPs injected mice was substantially inhibited (Fig. 5a and b). The therapeutic efficacy was also evidenced by hematoxylin and eosin (H&E) staining and terminal deoxynucleotidyl transferase-mediated dUTP nick-end labeling (TUNEL) staining, which revealed extensive tumor cell apoptosis after treatment with CaO2–Fe NPs (Fig. 5c). The effective generation of toxic ˙OH under TME with acidic pH and overexpressed GSH from CaO2–Fe NPs, leading to a remarkable tumor growth inhibition effect. Moreover, no apparent body weight changes were observed in mice injected with CaO2–Fe NPs during the whole period (Fig. 5d), and there was no obvious histological alteration in the major organs (Fig. S21, ESI†). No obvious physiological damages were observed in CaO2–Fe NPs treated group through blood biochemistry and hematology analysis (Fig. 5e and Fig. S22, ESI†). Therefore, it is feasible to use CaO2–Fe NPs as a smart CDT agent for effective tumor therapy with low toxicity.
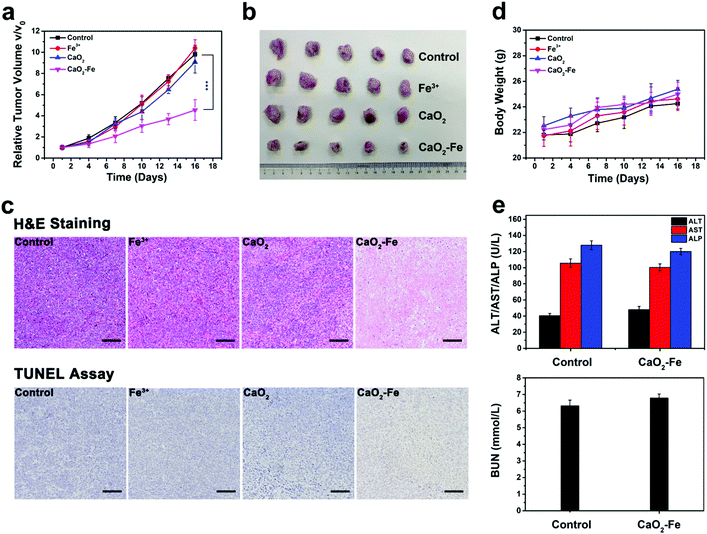 |
| Fig. 5 (a) Relative tumor growth curves of 4T1 tumor-bearing mice after treatment with saline (control group), FeCl3, CaO2 NPs, and CaO2–Fe NPs. (n = 5, mean ± s.d., ***p < 0.001) (b) A representative photo of dissected tumors from the different groups on day 17 after administration. (c) Images of H&E and TUNEL stained sections of tumors from the different groups on day 17 after administration. The scale bar represents 100 μm. (d) Time-dependent body-weight curves of mice in different groups. (n = 5, mean ± s.d.) (e) Blood biochemistry analysis of healthy mice after intravenously injected with saline or CaO2–Fe NPs for 17 days. (n = 3, mean ± s.d.). | |
Conclusions
In summary, an H2O2-replenishable and GSH-depletive ROS ‘bomb’ was successfully constructed for self-enhanced chemodynamic tumor therapy. After reaching tumor tissues, these ROS ‘bomb’, CaO2–Fe NPs, could be triggered due to the generation of Fe2+ ions by GSH. Meanwhile, amounts of H2O2 were generated by the reaction between CaO2 and H+. Eventually, with the accumulation of H2O2 as well as Fe2+ locally, a Fenton reaction cycle was achieved by continuously consuming GSH to output massive ROS, resulting in the improvement of the CDT efficacy by H2O2-supplementing and GSH-depletion. Both, in vitro and in vivo results demonstrated that CaO2–Fe NP presented an inspiring antitumor performance as well as low systemic toxicity. Therefore, these CaO2–Fe NPs could be regarded as a promising candidate for combining pH/GSH-responsive and GSH-depletion for CDT.
Experimental section
Materials
Calcium chloride (CaCl2, 96%), ammonium hydroxide (NH3·H2O, 28%), dopamine (99%), ferrous chloride (FeCl2, 98%), methylene blue (MB, 70%), N-acetyl cysteine (NAC), and glutathione (GSH, 99%) were obtained from J&K Scientific Ltd (Beijing, China). Ethanol (C2H6OH, 99.7%) and hydrogen peroxide (H2O2, 30%) were purchased from the Juhua Group Corporation.
Characterization
X-Ray diffraction patterns (XRD) was recorded using the X’Pert PRO X-ray diffractometer with Cu Kα (λ = 1.54 Å). Transmission electron microscopy (TEM) was performed using a FEI Tecnai G2 F30 microscope. X-Ray photoelectron spectroscopy (XPS) was performed using the Axis Ultra imaging photoelectron spectrometer (Kratos Analytical Ltd). Dynamic light scattering (DLS) measurements were conducted using the Zetasizer Nano ZS (Malvern). The concentrations of Fe and Ca were quantified using an inductively coupled plasma-atomic emission spectrometer (ICP-AES, NexION 350, PerkinElmer).
Synthesis of CaO2 NPs and CaO2–Fe NPs
CaCl2 (0.1 g) and dopamine (0.003 g) were first dissolved in ethanol (15 mL) with the help of ultrasound. Subsequently, NH4OH (1 mL) was added under magnetic stirring. Afterwards, H2O2 solution (0.2 mL) was injected slowly. The product (CaO2 NPs) was finally collected by centrifugation (8000 rpm), washed with ethanol three times, and redispersed in ethanol. CaO2 NPs were then reacted with FeCl2 to form CaO2–Fe NPs.
Colorimetric assay of peroxo groups
An aqueous solution containing KMnO4 (50 μg mL−1) and HCl (0.1 M) was first prepared. Subsequently, a certain amount of CaO2 NPs, CaO2–Fe NPs, or H2O2 was added into the mixture for 5 min. Finally, the mixture was measured by UV-vis spectra.
Chemodynamic activity of CaO2–Fe NPs
The degradation of methylene blue (MB) was used for quantitative analysis of ROS production based on CaO2–Fe NPs. In particular, the absorbance at λ = 644 nm of MB solution (25 mg L−1) in pH 7.4 or pH 5.4 with or without different concentrations of GSH (0, 1, 2, 3, 4, 5, and 10 mM) was measured before and after adding of 20 μg CaO2 NPs or CaO2–Fe NPs for 3 hours.
To confirm the ROS type from CaO2–Fe NPs, ESR spectroscopy was used. 5,5-Dimethyl-1-pyrroline N-oxide (DMPO) was utilized as the spin trap. DMPO solution (40 μL, 100 mmol L−1) was added into CaO2–Fe NP solution at different concentrations of GSH (0, 1, 3, and 10 mM) at pH 5.4. Subsequently, the above mixture (20 μL) was injected into a capillary, the results were recorded using a Bruker A300.
Cell culture
4T1 and L929 cell lines were obtained from Zhejiang Provincial People's Hospital. All biological reagents were purchased from Biological Industries. DMEM or 1640 with 10% FBS and 1% penicillin/streptomycin were treated as the cell culture medium. All cells were cultured in a cell incubator at 37 °C, 5% CO2 and 100% humidity.
Cytotoxicity assays
MTT assay was tested to evaluate the in vitro cytotoxicity. First, 5 × 103 per well 4T1 and L929 cells were seeded into 96-well plates and incubated overnight. Subsequently, various amounts of FeCl3, CaO2 NPs, and CaO2–Fe NPs at the same Ca or Fe concentrations were added. After further incubation for 24 h, a fresh cell culture medium with 5% 3-[4,5-dimethylthiazol-2-yl-]-2,5-diphenyltetrazolium bromide (MTT) was used to replace the culture medium with nanoparticles. Finally, dimethyl sulfoxide (DMSO, 100 μL) was used to replace the MTT solution and co-incubation for 4 h. Cell viability was measured using a Tecan m200. Furthermore, after 4T1 cells were treated after the above conditions, Calcein-AM and propidium iodide (PI) live/dead cell staining was used to further verify the cytotoxicity of CaO2–Fe NPs.
Intracellular ROS levels detection
5 × 104 per well 4T1 and L929 cells were plated into a 24-well plate and incubated overnight. Then, cells were incubated with FeCl3, CaO2 NPs, and CaO2–Fe NPs at the same Ca or Fe concentrations for 4 h. After washing with PBS, cells were stained with DCFH-DA (10 μM) for 30 min. Later, PBS was used to remove the free DCFH-DA. Finally, the fluorescence images were obtained using a Nikon ECLIPSE Ti.
Assessment of apoptosis
Annexin-V/PI assay kit (Sony) was used to determine the apoptosis of 4T1 cells treated with CaO2–Fe NPs using flow cytometry. In particular, 2 × 105 per well 4T1 cells were plated into a 6-well plate and incubated for 12 h. Afterwards, cells were treated with FeCl3, CaO2 NPs, and CaO2–Fe NPs at the same Ca or Fe concentrations for a further 24 h. After washing with PBS, cells were detached by trypsin. Finally, apoptosis was detected using flow cytometry (ACEA NovoCyte) using PI vs. Annexin V plots.
Animal modal
All animal experiments were performed abiding by the guidelines of the Institutional Animal Care and Use Committee (IACUC) of Zhejiang Provincial People's Hospital, Hangzhou, China. 6-Week female Balb/c mice were provided by Shanghai Sippe-Bk Lab Animal Co., Ltd, Shanghai, China. 1 × 106 4T1 cells solution (0.1 mL cells in PBS) was subcutaneously injected into the right axillary of all mice to construct the tumor model.
Tumor inhibition and in vivo toxicity assay
After tumor volume reached 100 mm3, 20 mice were randomly divided into 4 groups (n = 5): mice were intravenously injected with saline (as the control group), FeCl3, CaO2 NPs, and CaO2–Fe NPs at the same Ca or Fe concentrations. The whole experiment period was 15 days. All treatments were performed every three days and tumor volume and body weight were recorded 1-day after injection. Tumor volume was calculated using the formula as 0.5 × (length × width2).
On the 17th day, all mice were executed. Subsequently, tumors and major organs (heart, liver, spleen, lung, and kidney) were removed and stored in formalin. After the section of the tumors and major organs, hematoxylin and eosin (H&E) staining and terminal deoxynucleotidyl transferase-mediated dUTP nick-end labeling (TUNEL) staining were then detected. A Nikon ECLIPSE Ni-U was used to observe the slides.
Statistical analysis
The test data are shown as mean ± s.d. The student's two-tailed t-test was used to calculate the statistical comparisons. * means p < 0.05, ** means p < 0.01, *** means p < 0.001. p < 0.05 was regarded as statistically significant.
Author contributions
Fan Zhao: carried out all experiments and performed the statistical analysis, contributed to discussion, writing – original draft. Jiayu Yao: participated in animal studies and molecular biology experiments. Yu Tong: participated in animal studies and molecular biology experiments. Qing Xu: participated in molecular biology experiments. Dan Su: participated in molecular biology experiments. Juan Li: supervision. Yao Ying: supervision. Wangchang Li: supervision. Liang Qiao: supervision. Jingwu Zheng: supervision. Wei Cai: supervision. Xiaozhou Mou: conceptualization, supervision, writing – reviewing and editing. Shenglei Che: conceptualization, supervision, writing – reviewing and editing. Jing Yu: carried out all experiments and performed the statistical analysis, conceptualization, writing – review & editing. Yanglong Hou: conceptualization, supervision, writing – reviewing and editing.
Conflicts of interest
There are no conflicts to declare.
Acknowledgements
This work was supported in part by the National Natural Science Foundation of China (52073258 and 81672430), Natural Science Foundation of Zhejiang Province (LY20E020017 and LQ19H160016), Young Elite Scientist Sponsorship Program by CAST (2017QNRC001), the Fundamental Research Funds for the Provincial Universities of Zhejiang (RF-A2019004), and Foundation of Health Department of Zhejiang Province (2018KY239).
References
- C. Li, X. Zheng, W. Chen, S. Ji, Y. Yuan and X. Jiang, Tumor microenvironment-regulated and reported nanoparticles for overcoming the self-confinement of multiple photodynamic therapy, Nano Lett., 2020, 20, 6526–6534 CrossRef CAS PubMed.
- Y. Chen, Y. Huang, S. Zhou, M. Sun, L. Chen, J. Wang, M. Xu, S. Liu, K. Liang, Q. Zhang, T. Jiang, Q. Song, G. Jiang, X. Tang, X. Gao and J. Chen, Tailored chemodynamic nanomedicine improves pancreatic cancer treatment via controllable damaging neoplastic cells and reprogramming tumor microenvironment, Nano Lett., 2020, 20, 6780–6790 CrossRef CAS PubMed.
- V. G. Deepagan, D. G. You, W. Um, H. Ko, S. Kwon, K. Y. Choi, G.-R. Yi, J. Y. Lee, D. S. Lee, K. Kim, I. C. Kwon and J. H. Park, Long-circulating Au-TiO2 nanocomposite as a sonosensitizer for ROS-mediated eradication of cancer, Nano Lett., 2016, 16, 6257–6264 CrossRef CAS PubMed.
- H. Ranji-Burachaloo, P. A. Gurr, D. E. Dunstan and G. G. Qiao, Cancer treatment through nanoparticle-facilitated Fenton reaction, ACS Nano, 2018, 12, 11819–11837 CrossRef CAS PubMed.
- M. Wu, Y. Ding and L. Li, Recent progress in the augmentation of reactive species with nanoplatforms for cancer therapy, Nanoscale, 2019, 11, 19658–19683 RSC.
- Y. Wang, L. Shi, Z. Ye, K. Guan, L. Teng, J. Wu, X. Yin, G. Song and X. Zhang, Reactive oxygen correlated chemiluminescent imaging of a semiconducting polymer nanoplatform for monitoring chemodynamic therapy, Nano Lett., 2020, 20, 176–183 CrossRef CAS PubMed.
- C. Zhang, W. Bu, D. Ni, S. Zhang, Q. Li, Z. Yao, J. Zhang, H. Yao, Z. Wang and J. Shi, Synthesis of iron nanometallic glasses and their application in cancer therapy by a localized Fenton reaction, Angew. Chem., Int. Ed., 2016, 55, 2101–2106 CrossRef CAS PubMed.
- C. Wu, S. Wang, J. Zhao, Y. Liu, Y. Zheng, Y. Luo, C. Ye, M. Huang and H. Chen, Biodegradable Fe(III)@WS2-PVP nanocapsules for redox reaction and tme-enhanced nanocatalytic, photothermal, and chemotherapy, Adv. Funct. Mater., 2019, 29, 1901722 CrossRef.
- L. Zhang, S. Wan, C.-X. Li, L. Xu, H. Cheng and X. Zhang, An adenosine triphosphate-responsive autocatalytic Fenton nanoparticle for tumor ablation with self-supplied H2O2 and acceleration of Fe(III)/Fe(II) conversion, Nano Lett., 2018, 18, 7609–7618 CrossRef CAS PubMed.
- X. Chen, H. Zhang, M. Zhang, P. Zhao, R. Song, T. Gong, Y. Liu, X. He, K. Zhao and W. Bu, Amorphous Fe-based nanoagents for self-enhanced chemodynamic therapy by re-establishing tumor acidosis, Adv. Funct. Mater., 2020, 30, 1908365 CrossRef CAS.
- J. Yu, F. Zhao, W. Gao, X. Yang, Y. Ju, L. Zhao, W. Guo, J. Xie, X.-J. Liang, X. Tao, J. Li, Y. Ying, W. Li, J. Zheng, L. Qiao, S. Xiong, X. Mou, S. Che and Y. Hou, Magnetic reactive oxygen species nanoreactor for switchable magnetic resonance imaging guided cancer therapy based on pH-sensitive Fe5C2@Fe3O4 nanoparticles, ACS Nano, 2019, 13, 10002–10014 CrossRef CAS PubMed.
- X. Qian, J. Zhang, Z. Gu and Y. Chen, Nanocatalysts-augmented Fenton chemical reaction for nanocatalytic tumor therapy, Biomaterials, 2019, 211, 1–13 CrossRef CAS PubMed.
- M. Wu, Y. Ding and L. Li, Recent progress in the augmentation of reactive species with nanoplatforms for cancer therapy, Nanoscale, 2019, 11, 19658–19683 RSC.
- L.-S. Lin, J. Song, L. Song, K. Ke, Y. Liu, Z. Zhou, Z. Shen, J. Li, Z. Yang, W. Tang, G. Niu, H.-H. Yang and X. Chen, Simultaneous Fenton-like ion delivery and glutathione depletion by MnO2-based nanoagent to enhance chemodynamic therapy, Angew. Chem., Int. Ed., 2018, 57, 4902–4906 CrossRef CAS PubMed.
- X. Wang, F. Li, X. Yan, Y. Ma, Z.-H. Miao, L. Dong, H. Chen, Y. Lu and Z. Zha, Ambient aqueous synthesis of ultrasmall Ni0.85Se nanoparticles for noninvasive photoacoustic imaging and combined photothermal-chemotherapy of cancer, ACS Appl. Mater. Interfaces, 2017, 9, 41782–41793 CrossRef CAS PubMed.
- P. a. Ma, H. Xiao, C. Yu, J. Liu, Z. Cheng, H. Song, X. Zhang, C. Li, J. Wang, Z. Gu and J. Lin, Enhanced cisplatin chemotherapy by iron oxide nanocarrier-mediated generation of highly toxic reactive oxygen species, Nano Lett., 2017, 17, 928–937 CrossRef CAS PubMed.
- M. Huo, L. Wang, Y. Chen and J. Shi, Tumor-selective catalytic nanomedicine by nanocatalyst delivery, Nat. Commun., 2017, 8, 357 CrossRef PubMed.
- L.-H. Fu, C. Qi, Y.-R. Hu, J. Lin and P. Huang, Glucose oxidase-instructed multimodal synergistic cancer therapy, Adv. Mater., 2019, 31, 1808325 CrossRef.
- L.-H. Fu, C. Qi, J. Lin and P. Huang, Catalytic chemistry of glucose oxidase in cancer diagnosis and treatment, Chem. Soc. Rev., 2018, 47, 6454–6472 RSC.
- Y. Dai, Z. Yang, S. Cheng, Z. Wang, R. Zhang, G. Zhu, Z. Wang, B. C. Yung, R. Tian, O. Jacobson, C. Xu, Q. Ni, J. Song, X. Sun, G. Niu and X. Chen, Toxic reactive oxygen species enhanced synergistic combination therapy by self-assembled metal-phenolic network nanoparticles, Adv. Mater., 2018, 30, 1704877 CrossRef PubMed.
- S. Shen, M. Mamat, S. Zhang, J. Cao, Z. D. Hood, L. Figueroa-Cosme and Y. Xia, Synthesis of CaO2 nanocrystals and their spherical aggregates with uniform sizes for use as a biodegradable bacteriostatic agent, Small, 2019, 15, 1902118 CrossRef PubMed.
- M. Zhang, R. Song, Y. Liu, Z. Yi, X. Meng, J. Zhang, Z. Tang, Z. Yao, Y. Liu, X. Liu and W. Bu, Calcium-overload-mediated tumor therapy by calcium peroxide nanoparticles, Chem, 2019, 5, 2171–2182 CAS.
- S. Gao, Y. Jin, K. Ge, Z. Li, H. Liu, X. Dai, Y. Zhang, S. Chen, X. Liang and J. Zhang, Self-supply of O2 and H2O2 by a nanocatalytic medicine to enhance combined chemo/chemodynamic therapy, Adv. Sci., 2019, 6, 1902137 CrossRef CAS PubMed.
- L.-S. Lin, T. Huang, J. Song, X.-Y. Ou, Z. Wang, H. Deng, R. Tian, Y. Liu, J.-F. Wang, Y. Liu, G. Yu, Z. Zhou, S. Wang, G. Niu, H.-H. Yang and X. Chen, Synthesis of copper peroxide
nanodots for H2O2 self-supplying chemodynamic therapy, J. Am. Chem. Soc., 2019, 141, 9937–9945 CrossRef CAS PubMed.
- Z. Tang, Y. Liu, D. Ni, J. Zhou, M. Zhang, P. Zhao, B. Lv, H. Wang, D. Jin and W. Bu, Biodegradable nanoprodrugs: “Delivering” ROS to cancer cells for molecular dynamic therapy, Adv. Mater., 2020, 32, 1904011 CrossRef CAS PubMed.
- C. Liu, D. Wang, S. Zhang, Y. Cheng, F. Yang, Y. Xing, T. Xu, H. Dong and X. Zhang, Biodegradable biomimic copper/manganese silicate nanospheres for chemodynamic/photodynamic synergistic therapy with simultaneous glutathione depletion and hypoxia relief, ACS Nano, 2019, 13, 4267–4277 CrossRef CAS PubMed.
- F. Gong, L. Cheng, N. Yang, O. Betzer, L. Feng, Q. Zhou, Y. Li, R. Chen, R. Popovtzer and Z. Liu, Ultrasmall oxygen-deficient bimetallic oxide MnWOX nanoparticles for depletion of endogenous gsh and enhanced sonodynamic cancer therapy, Adv. Mater., 2019, 31, 1900730 CrossRef PubMed.
- G. Chen, Y. Yang, Q. Xu, M. Ling, H. Lin, W. Ma, R. Sun, Y. Xu, X. Liu, N. Li, Z. Yu and M. Yu, Self-amplification of tumor oxidative stress with degradable metallic complexes for synergistic cascade tumor therapy, Nano Lett., 2020, 20, 8141–8150 CrossRef CAS PubMed.
- Y. Liu, W. Zhen, L. Jin, S. Zhang, G. Sun, T. Zhang, X. Xu, S. Song, Y. Wang, J. Liu and H. Zhang, All-in-one theranostic nanoagent with enhanced reactive oxygen species generation and modulating tumor microenvironment ability for effective tumor eradication, ACS Nano, 2018, 12, 4886–4893 CrossRef CAS PubMed.
- B. Ma, S. Wang, F. Liu, S. Zhang, J. Duan, Z. Li, Y. Kong, Y. Sang, H. Liu, W. Bu and L. Li, Self-assembled copper-amino acid nanoparticles for in situ glutathione “AND” H2O2 sequentially triggered chemodynamic therapy, J. Am. Chem. Soc., 2019, 141, 849–857 CrossRef CAS PubMed.
- Z. Dong, L. Feng, Y. Chao, Y. Hao, M. Chen, F. Gong, X. Han, R. Zhang, L. Cheng and Z. Liu, Amplification of tumor oxidative stresses with liposomal Fenton catalyst and glutathione inhibitor for enhanced cancer chemotherapy and radiotherapy, Nano Lett., 2019, 19, 805–815 CrossRef CAS PubMed.
- Z. Dong, L. Feng, Y. Hao, M. Chen, M. Gao, Y. Chao, H. Zhao, W. Zhu, J. Liu, C. Liang, Q. Zhang and Z. Liu, Synthesis of hollow biomineralized CaCO3-polydopamine nanoparticles for multimodal imaging-guided cancer photodynamic therapy with reduced skin photosensitivity, J. Am. Chem. Soc., 2018, 140, 2165–2178 CrossRef CAS.
- L. Chen, Z. Lin, L. Liu, X. Zhang, W. Shi, D. Ge and Y. Sun, Fe2+/Fe3+ ions chelated with ultrasmall polydopamine nanoparticles induce ferroptosis for cancer therapy, ACS Biomater. Sci. Eng., 2019, 5, 4861–4869 CrossRef CAS PubMed.
Footnote |
† Electronic supplementary information (ESI) available: Supplementary figures and discussions. See DOI: 10.1039/d1ma00646k |
|
This journal is © The Royal Society of Chemistry 2022 |
Click here to see how this site uses Cookies. View our privacy policy here.