DOI:
10.1039/D2MA00309K
(Paper)
Mater. Adv., 2022,
3, 4649-4658
Smartphone-based digitized recognition of As3+ along with its effectual mitigation in water using a benzothiazole-functionalized molecular scaffold†
Received
17th March 2022
, Accepted 20th April 2022
First published on 6th May 2022
Abstract
In this work, an innovative azomethine-functionalized chromogenic and electrochemical chemosensor ABH [2-((acridin-9-yl)methylene)-1-(benzo[d]thiazol-2-yl)hydrazine] was explored, which was synthesized via a one-pot synthetic route with the aim of prolific and sequential aqueous-phase detection of As3+ and F−. The chemosensor exhibited distinct colorimetric transformation from yellow to burgundy upon addition of As3+, which upon subsequent addition of F− rejuvenated, suggesting a strategic application for designing three-input logic gate imitative electronic circuits derived from Boolean algebra. Several sophisticated wet chemical experiments, for instance, UV-Vis spectroscopy, ESI-MS, cyclic voltammetry, 1H-NMR spectroscopy, and FTIR spectroscopy along with theoretical computations substantiated the sensing phenomena. Furthermore, the development of an inexpensive portable arsenic sensor kit (ASK) in addition to a RGB-based smartphone-coupled diagnostic sensor station for rapid on-field detection of As3+ made the chemosensor an exclusive one as a value-added product in our society. Furthermore, the complexation ability of the sensor with toxic As3+ introduced it as a smart material for the aqueous-phase elimination of As3+.
1. Introduction
Arsenic (As3+), one of the most abundant minerals in the earth's crust, causes worldwide intimidation to the ecosystem and human health due to its severe toxicological effects. Drinking water with an elevated concentration of As3+ causes adverse health effects including arsenicosis, black foot disease, hyperkeratosis, fatigue, genotoxic and mutagenic effects and even cancer. As stated by the World Health Organization (WHO) and the US Environmental Protection Agency (USEPA), the acceptable level for As3+ in drinking water is 10 ppb.1 According to the reports, 20 countries worldwide suffer from exceeding As3+ concentration in drinking water.2 As a consequence, low-level efficacious recognition of As3+ in water is of considerable importance in recent times. The conventional As3+ detection techniques include atomic absorption spectroscopy (AAS), inductively coupled plasma-mass spectrometry (ICP-MS), atomic fluorescence spectrometry (AFS), inductively coupled plasma-atomic emission spectrometry (ICP-AES), graphite furnace-atomic absorption spectrometry (GF-AAS), and hydride generation-atomic absorption spectrometry (HG-AAS), which are highly expensive, time-consuming and require trained person.3,4 Moreover, the conventional colorimetric methods for As3+ recognition, based on the Gutzeit reaction, suffer from fewer optimistic response and lethal by-products such as AsH3 gas and mercury compounds.5a Therefore, introducing proficient chemical sensors,5b–d capable of detecting As3+ in water is of immense importance to the scientific community. In this perspective, metal nanoparticles have drawn considerable attention over conventional probes, which also suffer from various limitations such as lack of matrix interference, poor stability, high cost and the requirement of special agents to discriminatively detect As3+.6 Conversely, premeditated benzothiazole-functionalized azomethine-based derivatives are acquiring noteworthy consideration owing to their interesting nonlinear optical properties for naked eye detection of As3+ below the WHO permissible limit.7
Therefore, tailor-made synthesis of an inimitable azomethine-functionalized chemosensor, 2-((acridin-9-yl)methylene)-1-(benzo[d]thiazol-2-yl)hydrazine [ABH], was explored in this work, which showed unprecedented selectivity and sensitivity towards As3+ in a water medium. This benzothiazole-functionalized Schiff-base chemosensor was synthesized via a one-step condensation reaction by a simple wet chemical method and characterized by several analytical techniques such as ESI-MS, FTIR spectroscopy, SCXRD, and 1H-NMR spectroscopy. The presence of aqueous-phase As3+ can be selectively detected by the rapid colorimetric alteration of the chemosensor from yellow to burgundy even in the presence of other cations, owing to its bis-coordination to the N,N-chelate of the benzothiazole derivative, which was further confirmed by 1H-NMR titration studies along with density functional theory calculations.8 Interestingly, the ABH–As3+ adduct was found to be highly efficient to regenerate ABH selectively in the presence of F−, one of the most perilous anions due to its various fatal effects such as dental and skeletal fluorosis, and kidney failure in humans,9 which can further mimic an explicit progression of numerous logical functions, competent of performing molecular-level arithmetic computations.10 The pH of the ground water As3+ ranges from pH 5 to pH 6. Consequently, there exists a tendency of interference between the sensing response of As3+ and sensor solution in acidic pH. After synthesizing the sensor molecule, the halochromic behaviour was investigated. The ABH was found to exhibit colorimetric alteration at an extremely lower pH (pH 2), which signified that the sensing response of As3+ will not interfere with the working pH. Furthermore, to examine any interference caused by the solvatochromic alteration of ABH and sensing response of ABH with As3+, different solvatochromic responses (nonpolar to polar) have been considered and a subtle red-shift in the UV-Vis spectra with the increase in solvent polarity was found. However, in MeOH, like a low-polar protic solvent, compared to highly polar DMSO, such type of chromogenic alteration was not observed. Moreover, MeOH has been selected as sensing media for the high solubility of the sensor. Overall, these solvatochromic and halochromic properties of the sensor strengthen the recognition aptitude of ABH towards As3+. To the best of our knowledge, this type of sensory probe capable of consecutively detecting both As3+ and F− is yet to be explored in the literature.
However, updated strategies are required in clinical, food, and environmental realms for rapid analysis using sophisticated instrumentation. In this context, both Analytical Chemistry and Data Management are disciplines of paramount importance. Small, low-cost and portable analytical devices that can be used by inexperienced personnel to acquire accurate and precise data are of significant importance for the betterment of the society.11 This analytical device should be compatible with resource-limitations such as power supply, laboratory setup and skilled operators. At present, smartphones can be an ideal platform for monitoring contamination in ground water in large areas and the most suitable standpoint for providing device portability, analysis power, data processing and excellent communication abilities. Therefore, in this work, a portable smartphone-based archetype was fabricated in order to have a clear perception about the concentration of As3+ in groundwater based on the colorimetric alterations of ABH in the presence of different concentrations of As3+. This Bluetooth-operated smartphone-based prototype will also be effective to provide contactless data to minimize the risk of direct contact with the contaminants. As per our knowledge, a mobile platform for arsenic detection from ground water with systematized digital response is still less explored in the pertinent research territory.
Moreover, complex-based aqueous-phase mitigation of As3+ along with its effectual recognition using ABH is another advantageous key feature of this probe. Thus, in this work, our prime proposition is based on the exploration of a new economically viable and quick responsive colorimetric chemosensor (ABH) for the detection of As3+ in groundwater with high sensitivity and exceptional selectivity along with a smartphone-based digital response, which was further employed for the sequential recognition of F− and complexation-based mitigation of As3+.
2. Experimental
2.1. Materials
The requisite precursors and other solvents were used without further modification. All the cationic and anionic salts were purchased from Sigma Aldrich and Alfa Aesar Company. Solvents such as acetonitrile, dimethyl sulfoxide, dimethyl formamide, tetrahydrofuran, dichloromethane, hexane, methanol, acetone and water of spectroscopic grade were procured from Merck (India) Pvt. Ltd.
2.2. Synthesis and characterization
ABH was synthesized via a straightforward condensation reaction of 2-hydrazinobenzothiazole and acridine-9-carboxaldehyde in MeOH as a solvent medium along with the catalytic amount of acetic acid (Fig. S1, ESI†). The reaction takes place via a condensation reaction of aldehyde with a primary amine. After 12 hours of constant stirring, a yellow colored compound was obtained as the sole and end product of the reaction. The product was then collected by solvent evaporation. Yellow colored crystals of ABH were obtained by sluggish evaporation of a THF–HXN (1
:
1) solution. ABH was thereafter characterized by sophisticated analytical techniques such as CHNS analysis, ESI-MS, HR-MS, FT-IR spectroscopy, 1H-NMR spectroscopy (1D and 2D-COSY),13C-NMR spectroscopy and UV-Vis spectroscopy to confirm the structure and purity, (m/z) calculated 354 for C21H14N4S; found, 354.8 (ABH + H+) (Fig. S2, ESI†). CHN anal. calcd for C21H14N4S: C: 71.16%; H: 3.98%; N: 15.81%; S: 9.05%; found. C: 71.05%; H: 3.13%; N: 15.6%; S: 9%.1H-NMR (400 MHz, solvent: DMSO d6): δ = 9.4(s, 1H), δ = 8.8 (d, 2H, J = 8.87 Hz), δ = 8.2 (d,2H, J = 8.21 Hz), δ = 7.9(t, H, J = 7.92 Hz), δ = 7.7(t, 3H, J = 7.73), δ = 7.5 (d, 2H, J = 8 Hz), δ = 7.35 (t, 1H, J = 7.36), δ = 7.15 (t, 1H, J = 7.4), δ = 6.95 (s, 1H) (Fig. S3a, ESI†), 2D COSY 1H-NMR (Fig. S3b and Table S9, ESI†) and 13CNMR (Fig. S3c, ESI†).
2.3. Instrumentation
FT-IR spectroscopy (Spectrum 65, PerkinElmer) was used to confirm the functional groups present within the newly synthesized ABH moiety. The mass of ABH was corroborated using a mass spectrometer (Advion Make; Serial no. 3013-0140). A CARY60 spectrophotometer was used to perform the absorbance spectroscopic studies. X-Ray crystallography (Bruker D8 Venture (APEX-III) with photon detector) and 1H-NMR spectroscopy (Bruker 400 MHz NMR spectrometer) were performed to identify the exact structure and purity of ABH. Cyclic voltammetry was carried out using a CH instrument to monitor the electrochemical activity of ABH. Atomic absorption spectroscopy (iCE 3500 AAS atomic absorption spectrometer) was accomplished in acquaintance with complex-based As3+ removal.
2.4. X-Ray crystallographic discussion
The crystals of ABH (CCDC No. 2107203) were acquired owing to the sluggish evaporation of the THF–HXN solvent (1
:
1) mixture (Fig. 1 and Tables S1–S4, ESI†). The probe was revealed to be asymmetric in nature. In the thiazole part, the endo-cyclic –C
N, C–S bond distance and <CNC bond angle were found to be 1.29 Å, 1.75 Å and 109.5° respectively, which are almost similar to the conventional benzothiazole moiety with regard to endo-cyclic –C
N (1.31 Å), C–S (1.733 Å) bond length and <CNC (110.62°) bond angle.12 Moreover, exo-cylic –C–N, –N–N, –C
N and C–C bond distances were found to be 1.362 Å, 1.345 Å, 1.29 Å and 1.466 Å respectively. In the acrydine part, the endo-cyclic –C
N bond distance and the <CNC bond angle were found to be 1.35 Å and 118° respectively. Additionally, two ABH molecules were interconnected via extensive H-bonding interactions between endo-cyclic N (–C
N) and exo-cyclic –NH groups, which formed a closely packed rectangular dimeric assembly.
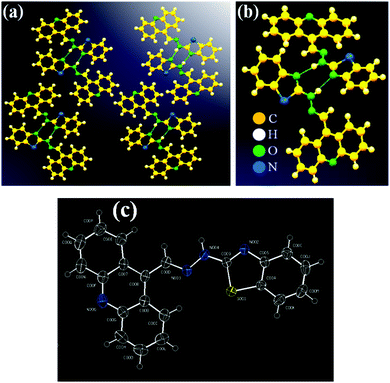 |
| Fig. 1 (a) H-bonding interaction within ABH between N of –C N (ring) and H of –NH; (b) closely packed rectangular shaped structure of ABH; and (c) ORTEP image of ABH (50% probability). | |
3. Results and discussions
3.1. Solvatochromism and halochromism exhibited by ABH
Interesting solvatochromic behavior was noticed for ABH in different solvents, wherein bathochromic shifts were observed with the increment in solvent polarity. To confirm these findings, the UV-Vis absorption spectra of ABH in both nonpolar and polar solvents (DCM, THF, ACN, MeOH, DMF and DMSO) were investigated [Fig. 2(a)]. The studies revealed that with the increment in solvent polarity from DCM to DMSO, the absorption spectra of ABH showed substantial red-shifting from 410 to 430 nm. Basically, enhancement of solvent polarity can stabilize the excited state of molecules and, therefore, diminish the energy gap among ground and excited states, facilitating the charge transfer from the ground state to the excited state at a higher wavelength (i.e. lower energy) [Fig. 2(c)].13,14 In addition, this newly synthesized azomethine-based chemosensor, ABH, was professed to specify a particular pH region, establishing the superiority of this chemosensor over previously reported sensory probes. The nature has preserved certain pH-dependent environments to maintain proficient functionalism. Each cell of our body works like a tiny molecular machine and has its own pH-dependent working environment. Alkalinity keeps these tiny machines healthy, whereas an acidic environment spawns an alarm indicating disease.15–20 In this relevance, the halochromic nature of ABH can play a feasible role. The initial base peak of ABH appearing at 430 nm remained constant at a biological pH of 6 to 8. However, at pH 2, the peak at 430 nm diminished through the generation of a new peak at 525 nm, indicated by a sharp color change from yellow (color of at biological pH) to violet red, while at pH 4, the peak broadened and ABH turned into firebrick color, which could be attributed to the acid-mediated charge transfer within the molecular scaffold. Therefore, these types of pH-dependent colorimetric alterations can further be a footprint towards the development of small molecule–based effectual colorimetric pH sensors [Fig. 2(b and d)].
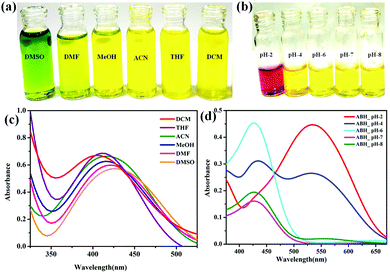 |
| Fig. 2 Pictorial representation of (a) solvatochromism and (b) halochromism exhibited by (1 × 10−4 M) ABH. UV-Vis spectral evidence of (c) solvatochromism and (d) halochromism produced by ABH. | |
3.2. Absorbance spectral outcomes of ABH with cations
The chromogenic response of ABH in the presence of several analytes is shown in Fig. 3(a), where it can be revealed that the sensor color changed from yellow to burgundy owing to the addition of As3+, while it remained unaltered in the presence of other metal ions. The optical response of the probe with As3+ was validated by UV-Vis spectroscopy. The free sensor showed a peak at 425 nm, which diminished in intensity with the generation of a new peak at 540 nm by the gradual addition of As3+, suggesting the charge transfer within the sensor and As3+ [Fig. 3(b)].21,22
 |
| Fig. 3 (a) Visual colorimetric responses of ABH (1 × 10−4 M) in MeOH towards different metal ions (1 × 10−4 M) in water and (b) UV-Vis absorption spectra of ABH (10−4 M, MeOH) upon sequential addition of As3+ (10−4 M, water). | |
The plausible stoichiometric interaction between ABH and As3+ was initially acquainted from Job's plot [Fig. S4, ESI†], which revealed the probable binding mode of ABH and As3+ to be 1
:
1. Thenceforward, this possibility of interaction has been well supported by upshot from ESI-MS of ABH–As3+ adducts [Fig. S5, ESI†]. The binding constant was also calculated using the Benesi–Hildebrand equation [Table 1 and Fig. S6(a), ESI†], which revealed that the binding constant was 0.47 × 103 M−1, indicating the effective interaction of the targeted analyte with the sensor moiety. The LOD (limit of detection) was also found to be 5.4 ppb, i.e. 72.1 nM [Fig. S6(b), ESI†]. Moreover, to get an insight into the requisite time of interaction of ABH with As3+, the kinetic study was performed, which showed that the mechanistic interaction pathway of ABH with As3+ followed a zero-order reaction kinetics with a rate constant of 2.6 × 10−3 mol L−1 s−1, signifying the independence of rate constant on the concentration of both of the sensor and the analyte [Table 1 and Fig. S7, ESI†].23
Table 1 Interaction of ABH with As3+ in terms of LOD, binding constant and reaction kinetics
Interaction between sensor and incoming analyte |
Limit of detection (LOD in ppb) |
Binding constant (K in M−1) |
Order and rate of the reaction (mol L−1 s−1) |
ABH–As3+ |
5.4 ppb |
0.47 × 103 |
2.6 × 10−3 |
3.3. Interference studies of ABH with different cationic analytes
The selectivity of ABH towards As3+ was affirmed from the interference studies, which were conducted by introducing As3+ into the ABH solution containing other competitive cations such as Zn2+,Cd2+, Pb2+, Hg2+, Mn2+, Mg2+, Ni2+, Co2+, Cu2+, Fe3+, La3+, Nd3+, Gd3+, Sb3+, Sm3+, Al3+, Cr3+, Au3+, +Bi3+, and Ca2+.24 The UV-Vis spectral responses of the ABH solution upon addition of As3+ in the presence of various other cations showed that As3+ induced an absorbance enhancement at 540 nm, while the other metal ions did not produce any significant variation even if the concentration of interfering ions was much higher than that of As3+, indicating high selectivity of ABH towards As3+ [Fig. S8(a–c), ESI†]. This excellent selectivity of ABH towards As3+ along with its anti-interference potentiality provides a prospective application to make a scrutiny of As3+ in groundwater.
3.4. Real-world application of ABH in determining As3+ in water
The present work was envisaged to detect As3+ from contaminated ground water samples. Therefore, aliquots of 300 μL of As3+ of different concentrations (10 ppb, 20 ppb, 50 ppb, 100 ppb and 1 ppm) were added to 500 μL of ABH solutions. Immediately, the yellow-colored sensor solution turned into pale yellow, yellowish orange, orange, reddish orange and burgundy as per the incremental concentration of As3+ [Fig. 4 and Fig. S9(a), ESI†]. Hence, ABH was perceived to be an efficient chemosensor towards detection of As3+ in water at both its nontoxic and toxic levels by varying the colorimetric responses, which is of utmost importance in recent times to defeat the worldwide As3+ contamination.
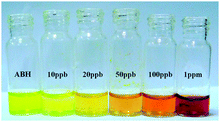 |
| Fig. 4 Pictorial view of the quantitative detection of As3+ in terms of RGB values. | |
3.5. Development of an As3+ Sensor Kit (ASK) and a Smartphone-coupled diagnostic sensor station for As3+ quantification in water
The concentration-dependent chromogenic sub-micro molar prompt response of ABH in the presence of As3+ instigated us to develop an As3+ sensor kit (ASK) [Fig. 5(a and b)] and a smartphone-coupled, RGB-based point-of-care testing (POCT) device [Fig. 6(a, b) and Fig. S9(b), ESI†] in order to acquire a coherent acuity regarding the concentration of As3+ in water samples [Fig. S10(a–f), ESI†]. Herein, the chemosensor solution (0.5 mL 10−4 M) was taken in a vial followed by the addition of 0.3 mL contaminated ground water sample. Instantaneous concentration–dependent chromogenic change has been observed upon shaking the vial for 3–5 seconds. The concentration-specific colors of the sensor-analyte solutions were thereafter employed to create a color chart, so that common people can tally the chromogenic change of the sensor after the addition of As3+ in order to be acquainted with the concentration of As3+. Accordingly, a sachet pack of arsenic sensor kit (ASK) was developed containing vial 1 (containing a chemosensor solution), vial 2, color chart, working methodology, tissue and soap paper. First, 0.3 mL contaminated water sample will have to be taken within the vial 2 up to the mark. Thereafter, the sensor solution will have to be added from vial 1 to vial 2 to mix the sensor-analyte solution and the observed color change will have to be tallied with the provided color chart. Therefore, rural people can have a preliminary idea about the concentration of As3+ in water.
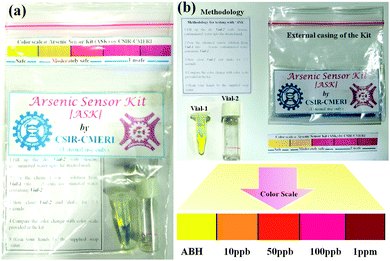 |
| Fig. 5 (a) Arsenic sensor kit (ASK) and (b) different components of ASK. | |
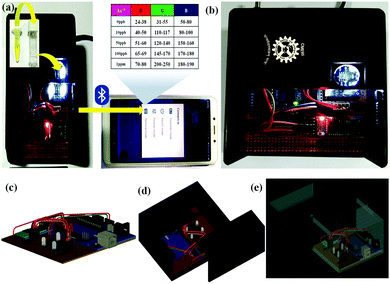 |
| Fig. 6 (a) Smartphone-coupled diagnostic sensor station for As3+ detection; (b) physical image of the fabricated device for the quantitative detection of As3+; (c) 3D CAD model of the circuit of the fabricated device; (d) 3D CAD model of the fabricated device; and (e) X-ray view of the fabricated device. | |
The ASK has further been employed towards the development of a Bluetooth-operated potable smartphone-based POCT device depending upon the RGB color code for rapid and on-field recognition of As3+. Unambiguously, the fabricated archetype comprises various unique features such as (i) straight measurement of photophysical parameters using integrated sensors, (ii) wireless connectivity with additional devices and (iii) easy operation. The entire prototype is allied with a TCS sensor and a HC-05 Bluetooth SPP module mounted on a designed circuitry board entrenched with a microcontroller along with the boot loader [Fig. S9(b), ESI† and Fig. 6]. The electronic circuitry was positioned in an acrylic-made black box [Fig. 6(b)] to inhibit the infiltration of the surrounding light towards the color sensor. In this device, the concomitant colorimetric responses of ABH in the presence of diverse concentrations of As3+ are recognized by the TCS color sensor and transpired via the integrated circuitry and the final output is observed in terms of RGB values on the smartphone via Arduino Bluetooth Controller by Giumig Apps. The different RGB values corresponding to different concentrations of As3+ clearly illustrate the concentration of As3+ within a specific water solution [Table 2]. Moreover, this Bluetooth-operated module will further take a cutting-edge outline towards the contactless analysis of As3+-like toxic analytes for the sustainability of our society. Therefore, this RGB-based Bluetooth-operated smartphone-coupled POCT device can be deployed along with the sachet kit and the table containing the experimentally observed RGB values of different concentration of As3+ in order to batch scale investigation of As3+ water samples.
Table 2 Obtained RGB values in the Smartphone-coupled device after consecutive addition of As3+ in the sensor
3.6. Proposition of electronic circuit fabrication
The attention-grabbing reversible outcomes of ABH upon sequential addition of As3+ and F− [Fig. S12(a and b), ESI†] were employed to design a specific logical circuitry, which imitates a particular sequence of molecular-level arithmetic calculations. Moleculators can perform logical operations based on one or more chemical inputs and a single output. In this case, numerous spectroscopic upshots of the chemical inputs produce a molecular-level complex logic gate circuitry, which can efficiently resolve assorted computational intricacies. The spectroscopic upshots of ABH with inward guests, As3+ and F−, are tabulated in Table 3. Several logic functions such as NAND, NOT, and NOR can be obtained by solving the truth table. A permutation of these logic functions formulates fascinating multifaceted circuits [Fig. 7 and Fig. S11, ESI†]. It was evidently observed from the UV-Vis spectra that by the concurrence of F− to the ABH–As3+ solution, the peak at 525 nm was omitted with the arrival of the sensor's initial peak at 425 nm, while further addition of As3+ again produced the response at 525 nm. Therefore, the inputs are as follows: In1: ABH; In2: As3+; and In3: F−. In brief, output Y2 will be ON if both inputs 1 (i.e.; sensor) and 2 (i.e.; As3+) are present. Thus, in the presence of successful input 3 (i.e.; F−) as the inhibitor, it will inhibit the interaction between the sensor and As3+ by forming AsF3: achievement of the inverter, i.e., NOT gate. Truth Table 2 successfully mimicked the AND–NOT–OR for ABH. Hence, the replication of the truth table input along with the output on the suggested logic circuit was accomplished productively.25,26
Table 3 Truth table for ABH with As3+ and F−
Input |
Output |
In1 (ABH) |
In2 As3+ |
In3 (F−) |
Output Y1 (425 nm) |
Output Y2 (525 nm) |
0 |
0 |
0 |
0 |
0 |
1 |
0 |
0 |
1 |
0 |
0 |
1 |
0 |
0 |
0 |
0 |
0 |
1 |
0 |
0 |
1 |
1 |
0 |
0 |
1 |
1 |
1 |
1 |
1 |
0 |
0 |
1 |
1 |
0 |
0 |
1 |
0 |
1 |
1 |
0 |
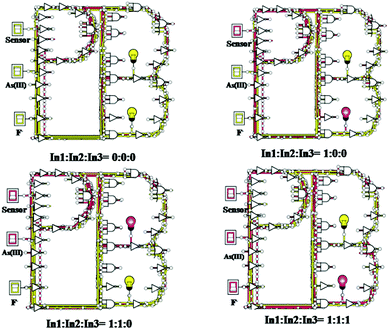 |
| Fig. 7 Fabrication of the three-input logic gate mimic ensemble based on NAND–NOT–NOR logic functions for ABH with As3+ and F−. | |
3.7. Cyclic voltammetric titration of ABH with As3+
The electrochemical experimentation of ABH with As3+ was accomplished in MeOH in an ambience with TBAPF6 as the supporting electrolyte, which showed noticeable alteration of the chemosensor's inherent oxidation-reduction potential upon addition of As3+. In the absence of As3+, the sensor showed quasi-reversibility, while upon addition of As3+ (120 μL), the anodic potential at 0.85 V (current height 0.013 mA) and 0.35 V (current height 0.0072 mA) was diminished with the generation of a new peak at 0.52 V (current height 0.0165 mA), indicating the oxidation of the probe upon addition of As3+. Additionally, the cathodic potential at 0.7 V (current height 0.0031 mA) and 0.35 V was obtained, signifying the feasibility of the corresponding reduction of the system in the cathode after binding As3+ [Fig. S13 and Table S5, ESI†].
3.7. Infrared spectroscopic experimentation of ABH with As3+
The infrared spectroscopy of ABH was performed in order to have an inclination regarding the host–guest interaction mode. The sensor exhibited a peak at 3408 cm−1, owing to the N–H stretching vibration of secondary amines and the appearance of a sharp peak at 3074 cm−1 may be attributed to the Ar–CH stretching vibration,27 while the peaks at 1627 cm−1, 1557 cm−1 and 750 cm−1 were attributed to the stretching vibration of –C
C, –C
N and C–S bonds respectively [Fig. S14, ESI†]. With the addition of As3+ to ABH, the characteristic peaks in the aromatic region (1450–1650 cm−1) were diminished due to interaction with As3+via N and S atoms of the probe. Moreover, the reduction of peak intensity in the specific region (1700 cm−1–1000 cm−1) signified the electronic allocation in the host–guest system with the addition of As3+ [Fig. S15, ESI†].8,28,29
3.8.
1H-NMR titration of ABH with AsI3
The 1H-NMR experiments of ABH with As3+ were performed in MeOD and D2O solvents with 10−4 M of the sensor and 10−4 M targeted analyte solutions in order to affirm the plausible mode of interaction involving ABH and As3+. The outcome of this study remains in a close-knit with all the spectroscopic and electrochemical experiments [Fig. 8]. With the addition of As3+, benzothiazole-functionalized aromatic ring protons (δ 8.85 ppm and δ 9.35 ppm) started shifting towards the downfield region, signifying the movement of charge density from ABH towards As3+, while the decrease in the –NH proton peak signified the interaction between As3+ with the nearby imine N and benzothiazole ring N centres.30,31
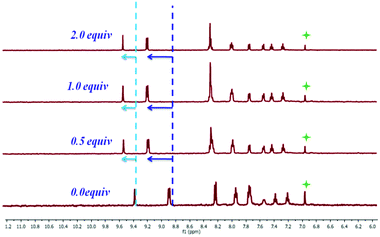 |
| Fig. 8
1H-NMR titration of ABH with As3+ (molar ratio of L to As3+; 5 : 3) in MeOD-D2O. | |
3.9. Theoretical substantiations
DFT computation was accomplished in the Turbomole (v7.0) in order to expand an obvious perception concerning the plausible mechanistic interaction among ABH and As3+, which were completely in proportion to the experimental findings. In the case of 1
:
1 ABH: As3+ adducts, the energies and the HOMO–LUMO gap of the host–guest moiety (1.579 eV) seemed to be inferior than those of the sensor molecule (3.012 eV), suggesting a strong host–guest interaction [Fig. 9]. The interaction energy was evaluated using the following equation:
ΔEint = E (A, B) − [E(A) + E(B)] |
where E(A, B) is the energy of the ABH: As3+ adduct, E(A) is the energy of ABH and E(B) is the energy of AsI3. At first, the geometry of ABH was optimized having an energy of −2258.23 kJ mol−1 prior to interaction with As3+ and the optimized energy of AsI3 was found to be −2270.07 kJ mol−1. However, after interaction of ABH with As3+, the interaction energy (ΔEint) was found to be −1412.62 kJ mol−1, implying a spontaneous chemical interaction [ESI† and Table 4]. Moreover, the interaction of ABH with As3+ and other interfering lethal trivalent cations was executed. ABH:As3+ adduct formation was obtained as energetically the most promising among other trivalent cationic adducts with ABH [Fig. S16, ESI†], which indicated the selectivity of As3+ among other trivalent congeners. The Lowdin population analysis in the DFT study reflected that addition of 1 equivalent As3+ resulted in diminution in electron density on nitrogen atoms of exo-cyclic and endo-cyclic (benzothiazole part) –C
N units, and the electron density on S atoms of the benzothiazole unit remained slightly rehabilitated, reflecting the feasibility of bis-coordinated N,N-chelate formation of the benzothiazole derivative with As3+. Furthermore, electron density on the nitrogen atom of acrydine part slightly altered due to the drifting of the electron cloud towards arseno-chelate [Table S8, ESI†].
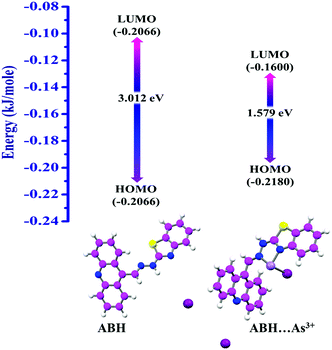 |
| Fig. 9 Geometrically optimized structures of ABH with As3+. | |
Table 4 Theoretical upshot of ABH: As3+ interaction
|
Total energy (KJ mol−1) |
HOMO−LUMO gap (eV) |
ΔEint = E (A, B) – [E(A) + E(B)] (KJ mol−1) |
ABH
|
−2837.8307 |
3.012 |
— |
ABH:As3+ |
−3694.4551 |
1.579 |
−1412.62 |
3.10. Riddance of As3+ in adulterated water
The complexation capability of ABH with As3+ instigated us to check its mitigation potential of As3+ in contaminated water. Initially, ABH resulted in a colorimetric change from yellow to burgundy upon addition of As3+ in water, while after a few hours nucleation followed by precipitation was observed at the vial, keeping the upper surface of the solution colorless. The UV-Vis and ESI-MS spectroscopic studies of the complex confirmed the ABH–As3+ complexation. Therefore, As3+ ions can be removed by filtering the As3+ complex from the solution. Furthermore, atomic absorption spectroscopy of the filtrate confirmed that 90% subsidence of As3+ occurred after separating the ABH: As3+ adduct [Fig. 10].
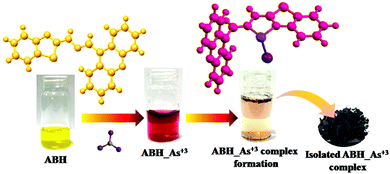 |
| Fig. 10 Removal of As3+ in water by ABH. | |
3.11. Comparative literature survey
In order to explore the advantage of this chemosensor, a thorough literature study was carried out. From the hitherto explored reports, it is observed that most of the probes showed selectivity towards As5+ and remained silent towards As3+, while As3+ is more toxic than As5+. Moreover, some of the synthesized probes showed equal selectivity towards trivalent cations, not specific towards As3+. Though plethora of As3+ detecting probes have been synthesized till date, aqueous-phase detection of As3+ still can be regarded as a challenge. Ground water contains AsO2−, AsO4−3, As3+ and As5+. Previously, some probes were designed in order to detect AsO2− (anionic form), which failed to detect As3+ (cationic form). Somewhere, expensive precursors such as gold salt and environmentally toxic materials were used in order to develop target-specific chemosensors. The newly synthesized chemosensor ABH (i) can detect As3+ from the aqueous phase using a smartphone-coupled device, (ii) can act as an efficient pH sensor by showing halo-chromism and (iii) can detect the solvent purity due to the solvatochromic behavior. Moreover, the newly synthesized chemosensor is nontoxic, inexpensive and shows almost 5 cycles of reversibility with F− [Table S9, ESI†].31–39
Conclusion
Briefly, the newly synthesized inexpensive chemosensor ABH can detect highly toxic As3+ in water with a detection limit (LOD) of 5.4 ppb. The solvatochromic behavior of the newly synthesized chemosensor makes it more efficient to verify the solvent purity, while the halochromism of the probe permits us to treat it as a pH sensor for lower pH values. Furthermore, a logic gate imitating electronic circuitry has been formulated by exploiting the successive reversibility of ABH with As3+ and F−, which further attributes the effective detection efficiency of the probe towards venomous As3+ and F− reversibly. In addition, the fabricated arsenic sensor kit (ASK) and smartphone-coupled diagnostic sensor station introduced the chemosensor as a value-added product in our society, which is explicitly unique of its kind. Moreover, this inimitable probe possesses the integral efficiency towards simultaneous detection and removal of toxic As3+ as a result of its complexation ability with As3+, which made it a smart and efficient material for aqueous-phase elimination of As3+.
Conflicts of interest
There are no conflicts to declare.
Acknowledgements
The authors sincerely acknowledge DST-DAAD funded project GAP-228512. Authors are thankful to Mr Abhijit Hazra, Research scholar, CSIR-CMERI, for his scientific inputs in designing the sensor molecule. AM is thankful to UGC for her UGC sponsored NFSC fellowship.
Notes and references
-
(a) N. Moghimi, M. Mohapatra and K. T. Leung, Bimetallic Nanoparticles for Arsenic Detection, Anal. Chem., 2015, 87(11), 5546–5552 CrossRef CAS PubMed;
(b) A. S. Saleemi, M. Hafeez, A. Munawar, N. Akhtar, W. Abbas, M. E. Mazhar, Z. Shafiq, A. P. Davis and S. L. Lee, Synthesis and Sensing Efficiency of CN Wrapped ZnFe2O4 Microspheres-Ionic Liquid Composite Towards Ultra-High Sensitivity Arsenic(III) Monitoring of Ground Drinking Water, J. Mater. Chem. C, 2020, 8, 12984–12992 RSC;
(c) X. Tian, L. Chen, Y. Li,a, C. Yang, Y. Nie, C. Zhoua and Y. Wang, Design and synthesis of molecule with aggregation-induced emission effect and its application in detection of arsenite in groundwater, J. Mater. Chem. C, 2017, 5, 3669–3672 RSC.
- C. Gao, X. Y. Yu, S. Q. Xiong, J. H. Liu and X. J. Huang, Electrochemical Detection of Arsenic(III) Completely Free from Noble Metal: Fe3O4 Microspheres-Room Temperature Ionic Liquid Composite Showing Better Performance than Gold, Anal. Chem., 2013, 85(5), 2673–2680 CrossRef CAS PubMed.
- L. Zhang, X. R. Chen, S. H. Wen, R. P. Liang and J. D. Qiu, Optical sensors for inorganic arsenic detection, TrAC, Trends Anal. Chem., 2019, 118, 869–879 CrossRef CAS.
- Y. Zhou, X. Huang, C. Liu, R. Zhang, X. Gu, G. Guan, C. Jiang, L. Zhang, S. Du, B. Liu, M. Y. Han and Z. Zhang, Color-Multiplexing-Based Fluorescent Test Paper: Dosage-Sensitive Visualization of Arsenic(III) with Discernable Scale as Low as 5 ppb, Anal. Chem., 2016, 88(12), 6105–6109 CrossRef CAS PubMed.
-
(a) L. Zeng, D. Zhou, J. Gong, C. Liu and J. Chen, Highly Sensitive Aptasensor for Trace Arsenic(III) Detection Using DNAzyme as the Biocatalytic Amplifier, J. Anal. Chem., 2019, 91(3), 1724–1727 CrossRef CAS PubMed;
(b) H. Sharma, N. Kaur, A. Singh, A. Kuwar and N. Singh, Optical Chemosensors for Water Sample Analysis, J. Mater. Chem. C, 2016, 4, 5154–5194 RSC;
(c) P. Kaur and K. Singh, Recent Advances in Application of BODIPY in Bioimaging and Chemosensing, J. Mater. Chem. C, 2019, 7, 11361–11405 RSC;
(d) X. N. Qi, L. R. Dang, W. J. Qu, Y. M. Zhang, H. Yao, Q. Lin and T. B. Wei, Based on the phenazine derivatives for optical sensing: a review, J. Mater. Chem. C, 2020, 8, 11308–11339 RSC.
- C. I. Weng, J. S. Cang, J. Y. Chang, T. M. Hsiung, B. Unnikrishnan, Y. L. Hung, Y. T. Tseng, Y. J. Li, Y. W. Shen and C. C. Huang, Detection of Arsenic(III) through Pulsed Laser-Induced Desorption/Ionization of Gold Nanoparticles on Cellulose Membranes, Anal. Chem., 2014, 86(6), 3167–3173 CrossRef CAS PubMed.
-
(a) S. Lohar, S. Pal, B. Sen, M. Mukherjee, S. Banerjee and P. Chattopadhyay, Selective and Sensitive Turn-on Chemosensor for Arsenite Ion at the ppb Level in Aqueous Media Applicable in Cell Staining, Anal. Chem., 2014, 86(22), 11357–11361 CrossRef CAS PubMed;
(b) J. F. Mike, J. J. Inteman, A. Ellern and M. Jeffries-EL, Facile Synthesis of 2,6-Disubstituted Benzobisthiazoles: Functional Monomers for the Design of Organic Semiconductors, J. Org. Chem., 2010, 75, 495–497 CrossRef CAS PubMed;
(c) M. Godfroy, J. Liotier, V. M. Mwalukuku, D. Joly, Q. Huaulme, L. Cabau, C. Aumaitre, Y. Kervella, S. Narbey, F. Ostwald, E. Palomares, C. A.-G. Flores, G. Oskam and R. Demadrille, Benzothiadiazole-based photosensitizers for efficient and stable dye-sensitized solar cells and 8.7% efficiency semi-transparent mini-modules, Sustainable Energy Fuels, 2021, 5, 144–153 RSC.
- K. Chauhan, P. Singh, B. Kumari and R. K. Singhal, Synthesis of new benzothiazole Schiff base as selective and sensitive colorimetric sensor for arsenic on-site detection at ppb level, Anal. Methods, 2017, 9(11), 1779–1785 RSC.
- X. Fan, D. J. Parker and M. D. Smith, Adsorption kinetics of fluoride on low cost materials, Water Res., 2003, 37(20), 4929–4937 CrossRef CAS PubMed.
- A. Mondal, A. Roy Chowdhury, S. Bhuyan, S. K. Mukhopadhyay and P. Banerjee, A simple urea-based multianalyte and multichannel chemosensor for the selective detection of F−, Hg2+ and Cu2+ in solution and cells and the extraction of Hg2+ and Cu2+ from real water sources: a logic gate mimic ensemble, Dalton Trans., 2019, 48, 4375–4386 RSC.
-
(a) A. Mukherjee, M. K. Sengupta, M. A. Hossain, S. Ahamed, B. Das, B. Nayak, D. Lodh, M. M. Rahman and D. Chakraborti, Arsenic Contamination in Groundwater: A Global Perspective with Emphasis on the Asian Scenario, J. Heal. Popul. Nutr., 2006, 24(2), 142–163 Search PubMed;
(b) Y. Zhao, M. Gobbi, L. E. Hueso and P. Samori, Molecular Approach to Engineer Two-Dimensional Devices for CMOS and beyond-CMOS Applications, Chem. Rev., 2022, 122(1), 50–131 CrossRef CAS PubMed;
(c) S. Franco, J. Garín, N. M.-D. Baroja, R. P. Tejada, J. Orduna, Y. Yu and M. L. Cantu, New D-π-A-Conjugated Organic Sensitizers Based on 4H-Pyran-4-ylidene Donors for Highly Efficient Dye-Sensitized Solar Cells, Org. Lett., 2012, 14(3), 752–755 CrossRef CAS PubMed;
(d) S. Schumann, R. Da Campo, B. Illy, A. C. Cruickshank, M. A. McLachlan, M. P. Ryan, D. J. Riley, D. W. Mc Comb and T. S. Jones, Inverted organic photovoltaic devices with high efficiency and stability based on metal oxide charge extraction layers, J. Mater. Chem., 2011, 21, 2381–2386 RSC;
(e) F. Rosei, M. Schunack, Y. Naitoh, P. Jiang, A. Gourdon, E. Laegsgaard, I. Stensgaard, C. Joachim and F. Besenbacher, Properties of large organic molecules on metal surfaces, Prog. Surf. Sci., 2003, 95–146 CrossRef CAS;
(f) M. Chen, L. Lu, H. Yu, C. Li and Ni Zhao, Integration of Colloidal Quantum Dots with Photonic Structures for Optoelectronic and Optical Devices, Adv. Sci., 2021, 8(18), 2101560 CrossRef CAS PubMed.
- H. G. Raubenheimer, E. K. Marais, S. Cronje, C. Esterhuysen and G. J. Kruger, J. Chem. Soc., Dalton Trans., 2000, 3016–3021 RSC.
- S. Nigam and S. Rutan, Principles and Applications of Solvatochromism, Appl. Spectrosc., 2001, 55, 362A–370A CrossRef CAS.
- A. Marini, A. Muñoz-Losa, A. Biancardi and B. Mennucci, What is solvatochromism?, J. Phys. Chem. B, 2010, 114(51), 17128–17135 CrossRef CAS PubMed.
- S. Halder, S. Dey and P. Roy, A quinoline based Schiff-base compound as pH sensor, RSC Adv., 2015, 5, 54873–54881 RSC.
- S. Halder, A. Bhattacharjee, A. Roy, S. Chatterjee and P. Roy, Chromogenic and fluorescence sensing of pH with a Schiff-base molecule, RSC Adv., 2016, 6, 39118–39124 RSC.
- C. Hazneci, K. Ertekin, B. Yenigul and E. Cetinkaya, Optical pH sensor based on spectral response of newly synthesized Schiff bases, Dyes Pigm., 2004, 62(1), 35–41 CrossRef CAS.
- K. Jiang, Y. C. Wu, H. Q. Wu, S. L. Li, S.
H. Luo and Z. Y. Wang, A highly selective, pH-tolerable and fast-response fluorescent probe for Fe3+ based on star-shape benzothiazole derivative, J. Photochem. Photobiol., A, 2018, 350, 52–58 CrossRef CAS.
- D. Maity, S. Halder and P. Roy, High pH Sensing Properties of a New Schiff-base Compound, ChemistrySelect, 2018, 3(2), 440–445 CrossRef CAS.
- V. Kachwal, I. S. Vamsi Krishna, L. Fageria, J. Chaudhary, R. R. Kinkar, R. Chowdhury and I. R. Laskar, Exploring the hidden potential of a benzothiazole-based Schiff-base exhibiting AIE and ESIPT and its activity in pH sensing, intracellular imaging and ultrasensitive & selective detection of aluminium (Al3+), Analyst, 2018, 143(15), 3741–3748 RSC.
- V. K. Gupta, A. K. Singh and L. K. Kumawat, Thiazole Schiff base turn-on fluorescent chemosensor for Al3+ ion, Sens. Actuators, B, 2014, 195, 98–108 CrossRef CAS.
-
(a) A. R. Chowdhury, P. Ghosh, S. Paul, S. Bhuyan, J. C.-K. Bose, S. Mukhopadhyay and P. Banerjee, A novel ditopic chemosensor for cadmium and fluoride and its possible application as a pH sensor, Anal. Methods, 2017, 9, 124–133 RSC;
(b) K. P. Goetz, D. Vermeulen, M. E. Payne, C. Kloc, L. E. McNeilb and O. D. Jurchescu, Charge-transfer complexes: new perspectives on an old class of compounds, J. Mater. Chem. C, 2014, 2, 3065–3076 RSC;
(c) T. Allen, S. Benis, N. Munera, J. Zhang, S. Dai, T. Li, B. Jia, W. Wang, S. Barlow, D. J. Hagan, E. W. Van Stryland, X. Zhan, J. W. Perry and S. R. Marder, Highly Conjugated, Fused-Ring, Quadrupolar Organic Chromophores with Large Two-Photon Absorption Cross-Sections in the Near-Infrared, J. Phys. Chem. A, 2020, 124(22), 4367–4378 CrossRef CAS PubMed.
- T. R. Knutson, C. M. Knutson, A. R. Mozzetti, A. R. Campos, C. L. Haynes and R. L. Penn, Quantifying Gold Nanoparticle Concentration in a Dietary Supplement Using Smartphone Colorimetry and Google Applications, J. Chem. Educ., 2015, 93(2), 318–321 Search PubMed.
- A. Mondal, A. Hazra, J. Chakrabarty, K. J.-C. Bose and P. Banerjee, Tandem Detection of Sub-Nano Molar Level CN– and Hg2+ in Aqueous Medium by a Suitable Molecular Sensor: A Viable Solution for Detection of CN– and Development of the RGB-Based Sensory Device, ACS Omega, 2020, 5(12), 6576–6580 CrossRef CAS PubMed.
- D. Tzeli and I. D. Petsalakis, Physical Insights into Molecular Sensors, Molecular Logic Gates, and Photosensitizers in Photodynamic Therapy, J. Chem., 2019, 6793490 Search PubMed.
-
(a) A. R. Chowdhury, P. Ghosh, B. G. Roy, S. K. Mukhopadhyay, P. Mitra and P. Banerjee, A simple and dual responsive efficient new Schiff base chemoreceptor for selective sensing of F− and Hg2+: application to bioimaging in living cells and mimicking of molecular logic gates, RSC Adv., 2015, 5, 62017–62023 RSC;
(b) K. M. Poulsen and T. Bjørnholm, Molecular electronics with single molecules in solid-state devices, Nat. Nanotechnol., 2009, 4, 551–556 CrossRef.
- M. Sobkowiak and P. Painter, Determination of the aliphatic and aromatic CH contents of coals by FT-ir: studies of coal extracts, Fuel, 1992, 71(10), 1105–1125 CrossRef CAS.
- S. Erdemir and O. Kocyigit, A new perylene bisimide-armed calix [4]-aza-crown as “turn on” fluorescent sensor for Hg2+ ion and its application to living cells, Sens. Actuators, B, 2015, 220, 381–388 CrossRef CAS.
- S. Daravath, N. Vamsikrishna, N. Ganji, K. Venkateswarlu and Shivaraj, Facile synthesis, structural characterization, DNA binding, incision evaluation, antioxidant and antimicrobial activity studies of Cobalt(II), Nickle(II) and Copper(II) complexes of 3-amino-5-(4-fluorophenyl)isoxazole derivatives, Chem. Data Collect., 2018, 17–18, 159–168 CrossRef.
- A. Rambabu, M. Pradeep Kumar, S. Tejaswi, N. Vamsikrishna and Shivaraj, DNA
interaction, antimicrobial studies of newly synthesized copper(II) complexes with 2-amino-6-(trifluoromethoxy) benzothiazole Schiff base ligands, J. Photochem. Photobiol., B, 2016, 165, 147–156 CrossRef CAS PubMed.
- A. R. Sadrolhosseini, M. Naseri and H. M. Kamari, Surface plasmon resonance sensor for detecting of arsenic in aqueous solution using polypyrrole-chitosan-cobalt ferrite nanoparticles composite layer, Opt. Commun., 2017, 383, 132–137 CrossRef CAS.
- M. Lo Presti, S. El Sayed, R. Martínez-Máñez, A. M. Costero, S. Gil, M. Parra and F. Sancenón, Selective chromo-fluorogenic detection of trivalent cations in aqueous environments using a dehydration reaction, New J. Chem., 2016, 40, 9042–9045 RSC.
- G. Bhanjana, N. Mehta, G. R. Chaudhary, N. Dilbaghi, K. H. Kim and S. Kumar, Emerging nanobiotechnology in agriculture for the management of pesticide residues, J. Mol. Liq., 2018, 264, 198–204 CrossRef CAS.
- A. Bonyár, P. Nagy, V. Mayer, A. Vitéz, A. Gerecs, H. Sántha and G. Harsányi, A colorimetry based, semi-automated portable sensor device for the detection of arsenic in drinking water, Sens. Actuators, B, 2017, 251, 1042–1049 CrossRef.
- K. Chauhan, P. Singh, B. Kumari and R. K. Singhal, Synthesis of new benzothiazole Schiff base as selective and sensitive colorimetric sensor for arsenic on-site detection at ppb level, Anal. Methods, 2017, 9, 1779–1785 RSC.
- V. C. Ezeh and T. C. Harrop, A sensitive and selective fluorescence sensor for the detection of arsenic(III) in organic media, Inorg. Chem., 2012, 51(3), 1213–1215 CrossRef CAS PubMed.
- J. R. Kalluri, T. Arbneshi, S. A. Khan, A. Neely, P. Candice, B. Varisli, M. Washington, S. McAfee, B. Robinson, S. Banerjee, A. K. Singh, D. Senapati and P. C. Ray, Use of gold nanoparticles in a simple colorimetric and ultrasensitive dynamic light scattering assay: selective detection of arsenic in groundwater, Angew. Chem., Int. Ed., 2009, 48(51), 9668–9671 CrossRef CAS PubMed.
- J. Li, L. Chen, T. Lou and Y. Wang, Highly Sensitive SERS Detection of As3+ Ions in Aqueous Media using Glutathione Functionalized Silver Nanoparticles, ACS Appl. Mater. Interfaces, 2011, 3(10), 3936–3941 CrossRef CAS PubMed.
- Y. Wu, S. Zhan, F. Wang, L. He, W. Zhi and P. Zhou, Cationic polymers and aptamers mediated aggregation of gold nanoparticles for the colorimetric detection of arsenic(III) in aqueous solution, Chem. Commun., 2012, 48, 4459–4461 RSC.
|
This journal is © The Royal Society of Chemistry 2022 |
Click here to see how this site uses Cookies. View our privacy policy here.