DOI:
10.1039/D2SC02363F
(Edge Article)
Chem. Sci., 2022,
13, 8583-8589
Excited-state palladium-catalysed reductive alkylation of imines: scope and mechanism†
Received
26th April 2022
, Accepted 1st July 2022
First published on 7th July 2022
Abstract
Palladium catalysis induced by visible-light irradiation is a promising tool for promoting unusual chemical transformations. We describe the development of excited-state palladium-catalyzed reductive alkylation of imines with alkyl bromides. The new methodology shows broad functional group tolerance and can additionally be applied in the direct three-component reaction of aldehydes, anilines, and alkyl bromides to give the alkyl amines under mild reaction conditions. Time-resolved photoluminescence experiments allowed the determination of the excited-state reaction kinetics and indicate that the reaction is proceeding via the inner-sphere electron transfer mechanism.
Introduction
Alkyl amines are important functional moieties that are presented in a wide range of drugs and natural products.1 The development of sustainable and environmentally benign strategies for their synthesis has been an enduring area of research. Among the different synthetic approaches available for the synthesis of alkyl amines, the addition of alkyl organometallics to imines is considered one of the most straightforward techniques.1a,2 Due to the moisture-sensitive nature of organometallic reagents and the instability of imines, most of the alkyl addition reactions have to be carried out under anhydrous conditions, which may limit their application in organic synthesis. Recently, the visible light-mediated photoredox catalysed alkylation of imines has been realized under mild reaction conditions,3 which has been noticed to be a new and elegant complementary method to the existing classical radical-based strategies [Fig. 1a; classical methods for the alkyl radical generation use alkyl iodides along with Et3B/O2 or metal reductants (tin reagents or Zn)].4 However, these photoredox methods are mainly applicable to more reactive alkyl-substituted radical precursors such as trifluoroborates, silicates, trimethylsilyl, redox-active esters, and substituted 1,4-dihydropyridines (Fig. 1b).5 In addition, the direct reduction of imines with photoredox catalysis to furnish a persistent radical anion intermediate that can undergo radical–radical cross-coupling to give hydroalkylation products has also been reported. However, these systems are limited to C–H activation of substrates with heteroatoms alpha to the site of functionalization.6 Moreover, these C–H activation methods do not provide a general solution for the versatile alkylation of imines. Furthermore, more expensive metal or non-metal alkyl radical precursors or selective heteroatom substituted alkyl derivatives and ruthenium or iridium polypyridyl complexes were often used, thus limiting the scope of the alkyl coupling partner to oxidizable functional groups. As a consequence, the development of new radical-mediated reductive alkylation reactions of imines using commercially available unactivated alkyl reagents (alkyl bromides) under mild visible light irradiation conditions would be desirable as it remained unexplored.
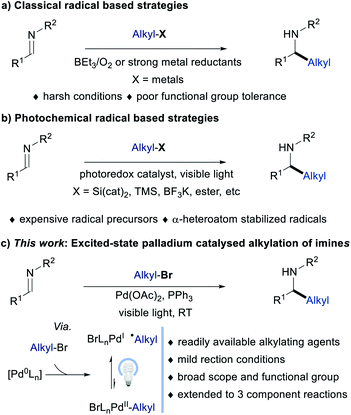 |
| Fig. 1 Strategies for radical-mediated alkylation of imines (a) Classical approach. (b) Photochemical approach. (c) This-work: excited-state Pd-catalyzed alkylation of imines. | |
Advances in visible light-mediated excited-state palladium catalysis have identified competent and versatile manifolds for the selective construction as well as functionalization of structurally diverse organic compounds.7 Particularly, the generation of Pd(I)/alkyl radical hybrid species and their reactivity in organic synthesis has been highlighted as a significant process that enables noticeable alkylations under mild reaction conditions, which are hard to realize via conventional thermal processes. For instance, excited-state palladium catalysed one-electron reduction of alkyl halides successfully overcomes various long-standing difficulties encountered in two-electron processes involving palladium, such as reluctant oxidative addition to tertiary alkyl halides, fast protonation of alkyl palladium intermediates and the undesired β-H elimination steps. Using this strategy, Gevorgyan,8 Shang and Fu,9 Glorius,10 Rueping,11 and others12 independently reported alkyl radical addition cross-coupling reactions, including the Mizoroki–Heck reactions, difunctionalizations, alkylations, and carbonylations. In most of the cases, a facile addition of Pd(I)/alkyl radical hybrid species to C
C bonds and its application in organic reactions were studied. However, the addition of Pd(I)/alkyl radical hybrid species to polar C
N bond for reductive alkylation of imines using alkyl halides remained elusive (Fig. 1c).13 In this regard Gevorgyan’s group recently reported the alkyl-Heck reaction of oximes using palladium and indium in the presence of visible light14a as well as a Pd-catalyzed Heck-type alkylation of ester hydrazones.14b Interestingly, the radical addition to oximes/hydrazones was followed by hydrogen atom transfer by Pd or radical recombination along with beta-hydride elimination to give the unsaturated product, while reduced amine was not formed.
Results and discussions
Given the overall interest in the development of imine alkylations with readily available unreactive alkyl bromides we started to investigate the reductive alkylation reaction by reacting imine 1a and bromocyclohexane 2f in the presence of Pd(PPh3)4 (10 mol%) and Cs2CO3 (2 equiv.) in DMSO under irradiation with 34 W blue LEDs which resulted in the desired alkylated amine 3f in 53% yield (Table 1, entry 1).
Table 1 Optimization of the reaction conditionsa
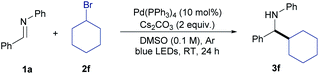
|
Entrya |
Change in the reaction conditions |
Yieldb (%) |
Reaction conditions: 1 (0.1 mmol), bromocyclohexane 2f (0.2 mmol), 34 W blue LEDs, RT, Ar, 24 h. RT = 32 ± 2 °C.
Yield of product determined by GC-FID using trimethoxybenzene as internal standard. Yield in bracket is the isolated one.
|
1 |
None |
53 |
2 |
MeOH or 1,4-dioxane as solvent |
40/28 |
3 |
CsOAc, K3PO4 or TEA as base |
43/43/26 |
4 |
5 mol% of Pd(PPh3)4 |
30 |
5 |
15 mol% of Pd(PPh3)4 |
81 |
6 |
Use of 10–40 mol% PPh3 as extra ligand |
68–89 |
7 |
10 mol% PdCl2 and 40 mol% PPh3 as catalyst |
48 |
8 |
10 mol% Pd(PPh3)2Cl2 and 40 mol% PPh3 as catalyst |
67 |
9 |
10 mol% Pd(PPh3)2Cl2 and 20 mol% Xantphos as catalyst |
28 |
10 |
10 mol% Pd(OAc)2 and 60 mol% PPh3 as catalyst |
70 |
11 |
10 mol% Pd(OAc)2 and 100 mol% PPh3 as catalyst |
93 (85) |
12 |
5 mol% Pd(OAc)2 and 100 mol% PPh3 as catalyst |
88 |
13 |
10 : 40 mol% Pd(OAc)2: PPh3, TEA (4 eq), Na2CO3 (1 eq.) |
46 |
14 |
10 : 40 mol% Pd(OAc)2: PPh3, Zn (3 eq.) |
34 |
Various protic and aprotic solvents were tested which however did not result in improved yield (Table 1, entry 2, also see ESI†). The use of other acetate, phosphate, and organic bases also did not have a considerable impact on the reaction yield (Table 1, entry 3). However increasing the loading of Pd(PPh3)4 from 5 to 15 mol% substantially increased the yield (entry 4, 5). This result along with other control experiments revealed that PPh3 is playing a dual role both as a ligand and as a reductant (Entry 7–9). To support this observation an extra 10–40 mol% of PPh3 was added which increased the yield to 89% (entry 6). The use of Pd(OAc)2 and PPh3 instead of Pd(PPh3)4 is equally effective which gave the product in 85% isolated yield (entry 11). To avoid the excess PPh3 being used, amines as reducing agents were employed in the reaction which is known from nickel catalysis (entry 13).15 Furthermore, Zn-powder was also used to reduce the amount of PPh3. However, the yield of the reductive alkylation product was not improved (entry 14, also see ESI†). Interestingly, apart from the reductive alkylation product, we did not observe alkyl-Heck products, i.e. imines, in any of the reactions.14
With the optimized conditions the scope of the reductive alkylation reaction was first evaluated by reacting N-benzylideneaniline 1a with varying alkyl bromides 2 (Table 2). A variety of primary, secondary, and tertiary alkyl bromides were found reactive under the standard reaction conditions. The reactivity of tertiary and secondary alkyl bromides (3a–n) was found to be superior compared to primary alkyl bromides (3o–r). Cyclic tertiary alkyl bromides that can undergo β-hydride elimination with Pd were also tolerated to give the alkylation product (3a and 3b). However, under the standard conditions employed for the coupling of imine 1a and tert-butyl bromide (non-cyclic), the reaction was not efficient (<20 yield). In this case, the β-hydride elimination seems faster if compared to the radical formation and addition to imine. The reactivity in the case of secondary alkyl bromides was found to increase with the increase in ring size 3c–g. Heteroatom containing alkyl bromides also underwent the reaction smoothly giving the alkylation product 3d and 3h–k in 70–93% yield. Bicyclic and primary alkyl bromides with various functional groups including chloro, ester, and phenyl substituents also gave the alkylated product 3m–r in good to moderate yields. The selective alkylation of the C–Br bond in the case of 1-bromo-6-chlorohexane provides an opportunity for further functionalization of the reaction product (3p).
Table 2 Scope of the reaction by varying alkyl bromides and iminesa
Reaction conditions: 1 (0.2 mmol), 2 (0.4 mmol), 34 W blue LEDs, Ar, 24 h; RT = 32 ± 2 °C; Yields of isolated products.
Reaction time 72 h.
d.r. between 1 : 1 to 1.2 : 1.
|
|
Next, we started to examine the scope of the reductive alkylation protocol concerning imines under the standard reaction conditions (Table 2). In the first instance, the benzylidene component of the imine was varied which gave the expected product 4a-j in good to moderate yields. Benzylidene moieties with electron-withdrawing groups at ortho- and para-position gave a better yield compared to electron-donating groups (4a–c and 4e–g). Likewise, an electron-donating group at meta-position (4d) gave a better yield compared to ortho- and para-substituents. Aryl boronate which is prone to undergo Suzuki coupling under palladium-catalysed conditions was also well tolerated (4h).16 Heterocyclic substrates also gave the expected product however in moderate yields which are due to the poisoning of the Pd by the strongly coordinating nitrogen and sulfur heteroatoms (4i and 4j).17 Next, the aniline component of the imine was varied to explore the effect of various substituents on the alkylation reaction (4k–o). Similarly, to the benzylidene component variation, electron-withdrawing groups at the para-position (4m–o) gave better yields compared to the electron-donating groups (4k and 4l). Additionally, different substituents on the arene ring were tolerated (4p–x). Further, the direct three-component reactions of benzaldehydes, anilines, and bromocyclohexane provided the expected reductive alkylation product 3f, 4g, and 4o in 59–78% yield under the standard conditions which highlights the feasibility of the protocol and avoiding the pre-synthesis step of imines (Fig. 2a).
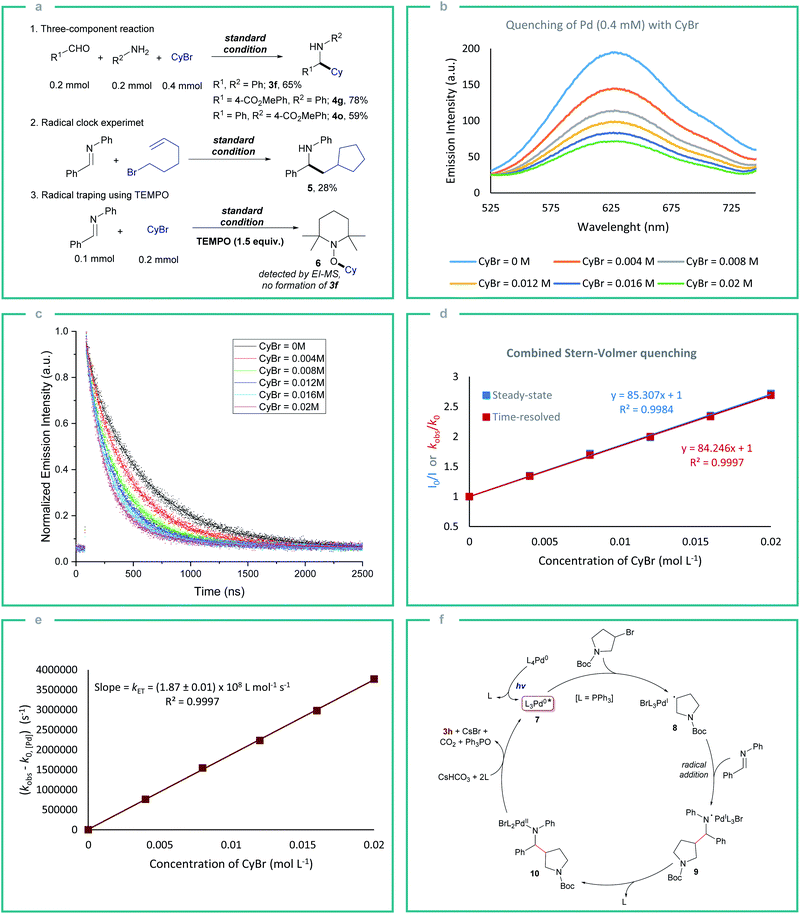 |
| Fig. 2 (a) Mechanistic experiments and the three-component reaction; (b) steady-state quenching of Pd(PPh3)4 (0.4 mM) with different concentrations of quencher (bromocyclohexane); (c) decrease in phosphorescence lifetimes of excited-state Pd(PPh3)4 (0.4 mM) upon increasing the concentration of bromocyclohexane; (d) steady-state and time-resolved Stern–Volmer quenching of Pd(PPh3)4 (0.4 mM) with different concentrations of bromocyclohexane; (e) plot of the observed rate constant (kobs) of *Pd(PPh3)4 corrected by its intrinsic ground state recovery rate (k0) vs. different concentrations of bromocyclohexane to determine the electron transfer rate constant (kET) between Pd and bromocyclohexane; (f) proposed mechanistic pathway for reductive alkylation of imines. | |
Subsequently, radical clock experiments were performed to understand if the light-mediated single electron oxidative addition of alkyl bromide to the Pd is operative (Fig. 2a). The reaction of 6-bromohex-1-ene with N-benzylideneaniline gave product 5 supporting the radical mechanism (Fig. 2a). Further, a radical trapping experiment using TEMPO under standard reaction conditions completely suppressed the product formation which also supports the single electron transfer (SET) mechanism. To gain insight into the nature of photoinduced SET between *Pd and alkyl bromide, steady-state (SSPL) and time-resolved photoluminescence (TRPL) quenching experiments were performed. The excited-state *Pd(PPh3)4 exhibits a long-lived triplet state with a lifetime of τ0 = 494.85 ± 1.62 ns, which may induce an inner-sphere SET with alkyl bromides. The steady-state quenching studies showed that the emission intensity of *Pd(PPh3)4 decreases with the increase of bromocyclohexane concentration (Fig. 2b). A similar result is also observed in TRPL quenching studies where a decrease in phosphorescence lifetimes of *Pd(PPh3)4 with increasing bromocyclohexane concentration was observed (Fig. 2c). Both the SSPL and TRPL Stern–Volmer plots displayed a perfect linear correlation which suggests that there is no static quenching between the Pd and alkyl bromide and that the quenching is dynamic (Fig. 2d). The quenching constant calculated from the Stern–Volmer slopes and the lifetime of *Pd was found to be kq = 1.7 × 108 M−1 s−1.18 Further, to determine the electron transfer rate constant (kET), the rate constant plot was plotted by using TRPL measurements (Fig. 2e). The plot of the observed rate (kobs) of *Pd(PPh3)4 corrected by its intrinsic ground state recovery rate without quencher (k0) versus different concentrations of bromocyclohexane gave a slope of (1.87 ± 0.01) × 108 L mol−1 s−1 which correlates with the electron transfer rate constant (kET) (Fig. 2e).6c,18
Based on these observations, a plausible reaction mechanism proceeding via an inner-sphere ET pathway is proposed (Fig. 2f). The in situ generated Pd(PPh3)4 complex undergoes excitation in the presence of visible light resulting in the triplet state *Pd0L3 (7) that provides an open coordination site for the alkyl bromide association. This results in the inner sphere electron transfer furnishing a hybrid alkyl radical PdI intermediate.11a The alkyl radical then adds to the imine resulting in an N-centered radical intermediate 9 which undergoes radical recombination with PdI to form a PdII intermediate 10 with the release of PPh3. To realize the product formation and the regeneration of Pd0 from intermediate 10, hydrogen and oxygen are required. Therefore, we initially tested the reaction using DMSO-d6 as a solvent which did not result in any deuterium incorporated alkylation product 3f which confirmed that the DMSO is not acting as a source of hydrogen. Besides, the formation of triphenylphosphine oxide is observed even in solvents without oxygen (CH3CN, toluene), leading to the conclusion that DMSO is neither hydrogen nor oxygen source. Thus, CsHCO3 generated in the reaction can be the source of hydrogen and oxygen, which facilitates the release of the hydroalkylation product 3 and Pd0 from intermediate 10 along with the generation of triphenylphosphine oxide which is observed in comparable yields to the amine 3 formed (Fig. 2f, also see ESI Fig. S8 and S9†). Other plausible pathways for PdII reduction are also discussed in the ESI (Fig. S8)†.
Conclusions
In summary, we developed a new method for the reductive alkylation of imines with readily available bromoalkanes by the photoexcitation of Pd in the presence of visible light. Imines with a wide range of functional groups are tolerated in this mild process. The reactivity and utility of this protocol are also demonstrated by the direct three-component reaction of aldehyde, amine, and alkyl bromide. Time-resolved photoluminescence experiments were performed for the first time to determine the excited-state kinetics and to support the formation of the reactive intermediates in photoexcited state Pd catalysis.
Data availability
The data for this work, including optimization tables, experimental procedures, and characterization data for allcompounds are provided in the ESI.†
Author contributions
M. R., R. K., and K. M. conceived and designed the project. R. K., and K. M. performed and analysed the experiments. R. K., and K. M. contributed equally to this work. M. R., R. K., and K. M. wrote the manuscript. M. R. directed the whole research.
Conflicts of interest
There are no conflicts to declare.
Acknowledgements
This publication is based upon work supported by the King Abdullah University of Science and Technology (KAUST), Office of Sponsored Research (OSR) under URF/1/4025.
References
-
(a) D. Ferraris, Tetrahedron, 2007, 39, 9581–9597 CrossRef;
(b) J. R. Liddell, Nat. Prod. Rep., 2002, 19, 773–781 RSC;
(c) T. C. Nugent and M. El-Shazly, Adv. Synth. Catal., 2010, 352, 753–819 CrossRef CAS;
(d) A. P. Taylor, R. P. Robinson, Y. M. Fobian, D. C. Blakemore, L. H. Jones and O. Fadeyi, Org. Biomol. Chem., 2016, 14, 6611–6637 RSC.
-
(a) S. Kobayashi and H. Ishitani, Chem. Rev., 1999, 99, 1069–1094 CrossRef CAS PubMed;
(b) M. Hatano, S. Suzuki and K. Ishihara, J. Am. Chem. Soc., 2006, 128, 9998–9999 CrossRef CAS PubMed;
(c) K.-i. Yamada and K. Tomioka, Chem. Rev., 2008, 108, 2874–2886 CrossRef CAS;
(d) M. Yus, J. C. Gonzalez-Gomez and F. Foubelo, Chem. Rev., 2011, 111, 7774–7854 CrossRef CAS;
(e) M. Hatano, K. Yamashita, M. Mizuno, O. Ito and K. Ishihara, Angew. Chem., Int. Ed., 2015, 54, 2707–2711 CrossRef CAS.
-
(a) A. Shatskiy, A. Axelsson, E. V. Stepanova, J.-Q. Liu, A. Z. Temerdashev, B. P. Kore, B. Blomkvist, J. M. Gardner, P. Dinér and M. D. Kärkäs, Chem. Sci., 2021, 12, 5430–5437 RSC;
(b) J. Wang, Z. Shao, K. Tan, R. Tang, Q. Zhou, M. Xu, Y.-M. Li and Y. Shen, J. Org. Chem., 2020, 85, 9944–9954 CrossRef CAS PubMed;
(c) D. P. Plasko, C. J. Jordan, B. E. Ciesa, M. A. Merrill and J. M. Hanna, Photochem. Photobiol. Sci., 2018, 17, 534–538 CrossRef CAS PubMed;
(d) H.-H. Zhang and S. Yu, J. Org. Chem., 2017, 82, 9995–10006 CrossRef CAS;
(e) S.-Y. Hsieh and J. W. Bode, Org. Lett., 2016, 18, 2098–2101 CrossRef CAS;
(f) N. R. Patel, C. B. Kelly, A. P. Siegenfeld and G. A. Molander, ACS Catal., 2017, 7, 1766–1770 CrossRef CAS PubMed;
(g) J. Jia, Q. Lefebvre and M. Rueping, Org. Chem. Front., 2020, 7, 602–608 RSC;
(h) S. T. Cullen and G. K. Friestad, Org. Lett., 2019, 21, 8290–8294 CrossRef CAS PubMed;
(i) A. F. Garrido-Castro, H. Choubane, M. Daaou, M. C. Maestro and J. Alemán, Chem. Commun., 2017, 53, 7764–7767 RSC;
(j) L. R. Pantaine, J. A. Milligan, J. K. Matsui, C. B. Kelly and G. A. Molander, Org. Lett., 2019, 21, 2317–2321 CrossRef CAS PubMed;
(k) J. Yi, S. O. Badir, R. Alam and G. A. Molander, Org. Lett., 2019, 21, 4853–4858 CrossRef CAS;
(l) J. Guo, Q.-L. Wu, Y. Xie, J. Weng and G. Lu, J. Org. Chem., 2018, 83, 12559–12567 CrossRef CAS PubMed;
(m) P. Ji, Y. Zhang, Y. Wei, H. Huang, W. Hu, P. A. Mariano and W. Wang, Org. Lett., 2019, 21, 3086–3092 CrossRef CAS.
-
(a) G. K. Friestad, Tetrahedron, 2001, 57, 5461–5496 CrossRef CAS;
(b) J. A. Fernandez-Salas, M. C. Maestro, M. M. Rodríguez-Fernández, J. L. Garcia-Ruano and I. Alonso, Org. Lett., 2013, 15, 1658–1661 CrossRef CAS PubMed;
(c) J. A. Fernández-Salas, M. M. Rodríguez-Fernández, M. C. Maestro and J. L. García-Ruano, Eur. J. Org. Chem., 2014, 2014, 5265–5272 CrossRef;
(d) S. Fujii, T. Konishi, Y. Matsumoto, Y. Yamaoka, K. Takasu and K.-I. Yamada, J. Org. Chem., 2014, 79, 8128–8133 CrossRef CAS PubMed;
(e) K.-I. Yamada, T. Konishi, M. Nakano, S. Fujii, R. Cadou, Y. Yamamoto and K. Tomioka, J. Org. Chem., 2012, 77, 1547–1553 CrossRef CAS;
(f) S. Ni, A. F. Garrido-Castro, R. R. Merchant, J. N. de Gruyter, D. C. Schmitt, J. J. Mousseau, G. M. Gallego, S. Yang, M. R. Collins and J. X. Qiao, Angew. Chem., Int. Ed., 2018, 57, 14560–14565 CrossRef CAS;
(g) M. Ueda, H. Miyabe, O. Miyata and T. Naito, Tetrahedron, 2009, 65, 1321–1326 CrossRef CAS;
(h) H. Miyabe, M. Ueda and T. Naito, Chem. Commun., 2000, 2059–2060 RSC;
(i) H. Miyabe, M. Ueda and T. Naito, Synlett, 2004, 2004, 1140–1157 Search PubMed.
- J. A. Leitch, T. Rossolini, T. Rogova, J. A. P. Maitland and D. J. Dixon, ACS Catal., 2020, 10, 2009–2025 CrossRef CAS.
-
(a) D. Hager and D. W. MacMillan, J. Am. Chem. Soc., 2014, 136, 16986–16989 CrossRef CAS;
(b) E. Fava, A. Millet, M. Nakajima, S. Loescher and M. Rueping, Angew. Chem., Int. Ed., 2016, 55, 6776–6779 CrossRef CAS;
(c) J. Jia, R. Kancherla, M. Rueping and L. Huang, Chem. Sci., 2020, 11, 4954–4959 RSC;
(d) D. Uraguchi, N. Kinoshita, T. Kizu and T. Ooi, J. Am. Chem. Soc., 2015, 137, 13768–13771 CrossRef CAS;
(e) M. Fernández and R. Alonso, Org. Lett., 2003, 5, 2461–2464 CrossRef;
(f) S. Yang, S. Zhu, D. Lu and Y. Gong, Org. Lett., 2019, 21, 2019–2024 CrossRef CAS;
(g) G. Deng and C.-J. Li, Tetrahedron Lett., 2008, 49, 5601–5604 CrossRef CAS;
(h) B. T. Matsuo, J. T. M. Correia and M. W. Paixao, Org. Lett., 2020, 22, 7891–7896 CrossRef CAS PubMed.
-
(a) P. Chuentragool, D. Kurandina and V. Gevorgyan, Angew. Chem., Int. Ed., 2019, 58, 11586–11598 CrossRef CAS;
(b) R. Kancherla, K. Muralirajan, A. Sagadevan and M. Rueping, Trends Chem., 2019, 1, 510–523 CrossRef CAS;
(c) W.-J. Zhou, G.-M. Cao, Z.-P. Zhang and D.-G. Yu, Chem. Lett., 2019, 48, 181–191 CrossRef CAS;
(d) W.-M. Cheng and R. Shang, ACS Catal., 2020, 10, 9170–9196 CrossRef CAS;
(e) K. P. S. Cheung, S. Sarkar and V. Gevorgyan, Chem. Rev., 2022, 122, 1543–1625 CrossRef;
(f) V. M. Chernyshev and V. P. Ananikov, ACS Catal., 2022, 12, 1180–1200 CrossRef CAS.
-
(a) D. Kurandina, M. Parasram and V. Gevorgyan, Angew. Chem., Int. Ed., 2017, 56, 14212–14216 CrossRef CAS PubMed;
(b) D. Kurandina, M. Rivas, M. Radzhabov and V. Gevorgyan, Org. Lett., 2018, 20, 357–360 CrossRef CAS;
(c) K. P. S. Cheung, D. Kurandina, T. Yata and V. Gevorgyan, J. Am. Chem. Soc., 2020, 142, 9932–9937 CrossRef;
(d) X. Jia, Z. Zhang and V. Gevorgyan, ACS Catal., 2021, 11, 13217–13222 CrossRef CAS PubMed.
-
(a) G.-Z. Wang, R. Shang, W.-M. Cheng and Y. Fu, J. Am. Chem. Soc., 2017, 139, 18307–18312 CrossRef CAS PubMed;
(b) G.-Z. Wang, R. Shang and Y. Fu, Org. Lett., 2018, 20, 888–891 CrossRef CAS PubMed;
(c) B. Zhao, R. Shang, G.-Z. Wang, S. Wang, H. Chen and Y. Fu, ACS Catal., 2019, 10, 1334–1343 CrossRef;
(d) G.-Z. Wang, R. Shang and Y. Fu, Synthesis, 2018, 50, 2908–2914 CrossRef CAS;
(e) W.-L. Xing, R. Shang, G.-Z. Wang and Y. Fu, Chem. Commun., 2019, 55, 14291–14294 RSC;
(f) W.-M. Cheng, R. Shang and Y. Fu, Nat. Commun., 2018, 9, 5215 CrossRef PubMed.
-
(a) M. Koy, F. Sandfort, A. Tlahuext-Aca, L. Quach, C. G. Daniliuc and F. Glorius, Chem.– Eur. J., 2018, 24, 4552–4555 CrossRef CAS PubMed;
(b) M. Koy, P. Bellotti, F. Katzenburg, C. G. Daniliuc and F. Glorius, Angew. Chem., Int. Ed., 2020, 59, 2375–2379 CrossRef CAS PubMed;
(c) H.-M. Huang, M. Koy, E. Serrano, P. M. Pflüger, J. L. Schwarz and F. Glorius, Nat. Catal., 2020, 3, 393–400 CrossRef CAS;
(d) H.-M. Huang, P. Bellotti, P. M. Pflüger, J. L. Schwarz, B. Heidrich and F. Glorius, J. Am. Chem. Soc., 2020, 142, 10173–10183 CrossRef CAS PubMed.
-
(a) R. Kancherla, K. Muralirajan, B. Maity, C. Zhu, P. Krach, L. Cavallo and M. Rueping, Angew. Chem., Int. Ed., 2019, 58, 3412–3416 CrossRef CAS;
(b) K. Muralirajan, R. Kancherla, A. Gimnkhan and M. Rueping, Org. Lett., 2021, 23, 6905–6910 CrossRef CAS PubMed.
-
(a) W. J. Zhou, G. M. Cao, G. Shen, X. Y. Zhu, Y. Y. Gui, J. H. Ye, L. Sun, L. L. Liao, J. Li and D. G. Yu, Angew. Chem., Int. Ed., 2017, 56, 15683–15687 CrossRef CAS PubMed;
(b) S. Sun, C. Zhou, J.-T. Yu and J. Cheng, Org. Lett., 2019, 21, 6579–6583 CrossRef CAS PubMed;
(c) Z. Jiao, L. H. Lim, H. Hirao and J. S. Zhou, Angew. Chem., Int. Ed., 2018, 57, 6294–6298 CrossRef CAS PubMed;
(d) G. M. Torres, Y. Liu and B. A. Arndtsen, Science, 2020, 368, 318–323 CrossRef CAS PubMed;
(e) J.-W. Ma, X. Chen, Z.-Z. Zhou and Y.-M. Liang, J. Org. Chem., 2020, 85, 9301–9312 CrossRef CAS PubMed;
(f) D. Kim, G. S. Lee, D. Kim and S. H. Hong, Nat. Commun., 2020, 11, 5266 CrossRef CAS;
(g) Z. Yang and R. M. Koenigs, Chem.– Eur. J., 2021, 27, 3694–3699 CrossRef CAS PubMed;
(h) L. Sun, J.-H. Ye, W.-J. Zhou, X. Zeng and D.-G. Yu, Org. Lett., 2018, 20, 3049–3052 CrossRef CAS PubMed;
(i) G. S. Lee, D. Kim and S. H. Hong, Nat. Commun., 2021, 12, 991 CrossRef CAS PubMed;
(j) W. Yao, G. Zhao, Y. Wu, L. Zhou, U. Mukherjee, P. Liu and M.-Y. Ngai, J. Am. Chem. Soc., 2022, 144, 3353–3359 CrossRef CAS PubMed.
- G. K. Friestad and A. K. Mathies, Tetrahedron, 2007, 63, 2541–2569 CrossRef CAS.
-
(a) N. Kvasovs, V. Iziumchenko, V. Palchykov and V. Gevorgyan, ACS Catal., 2021, 11, 3749–3754 CrossRef CAS PubMed;
(b) N. Kvasovs and V. Gevorgyan, Org. Lett., 2022, 24, 4176–4181 CrossRef CAS PubMed.
- A. Garcia-Dominguez, Z. Li and C. Nevado, J. Am. Chem. Soc., 2017, 139, 6835–6838 CrossRef CAS PubMed.
- M. R. Netherton, C. Dai, K. Neuschütz and G. C. Fu, J. Am. Chem. Soc., 2001, 123, 10099–10100 CrossRef CAS PubMed.
- Y.-J. Liu, H. Xu, W.-J. Kong, M. Shang, H.-X. Dai and J.-Q. Yu, Nature, 2014, 515, 389–393 CrossRef CAS PubMed.
-
(a) R. Kancherla, K. Muralirajan, B. Maity, S. Karuthedath, G. S. Kumar, F. Laquai, L. Cavallo and M. Rueping, Nat. Commun., 2022, 13, 2737 CrossRef CAS;
(b) S. Mukherjee, T. Patra and F. Glorius, ACS Catal., 2018, 8, 5842–5846 CrossRef CAS;
(c) M. Teders, C. Henkel, L. Anhäuser, F. Strieth-Kalthoff, A. Gómez-Suárez, R. Kleinmans, A. Kahnt, A. Rentmeister, D. Guldi and F. Glorius, Nat. Chem., 2018, 10, 981–988 CrossRef CAS;
(d) H. Yue, C. Zhu, R. Kancherla, F. Liu and M. Rueping, Angew. Chem., Int. Ed., 2020, 59, 5738–5746 CrossRef CAS PubMed;
(e) L. Huang, C. Zhu, L. Yi, H. Yue, R. Kancherla and M. Rueping, Angew. Chem., Int. Ed., 2020, 59, 457–464 CrossRef CAS PubMed.
|
This journal is © The Royal Society of Chemistry 2022 |
Click here to see how this site uses Cookies. View our privacy policy here.