DOI:
10.1039/D2LC00888B
(Paper)
Lab Chip, 2023,
23, 125-135
Versatile, facile and low-cost single-cell isolation, culture and sequencing by optical tweezer-assisted pool-screening†
Received
24th September 2022
, Accepted 29th November 2022
First published on 29th November 2022
Abstract
Real-time image-based sorting of target cells in a precisely indexed manner is desirable for sequencing or cultivating individual human or microbial cells directly from clinical or environmental samples; however, the versatility of existing methods is limited as they are usually not broadly applicable to all cell sizes. Here, an optical tweezer-assisted pool-screening and single-cell isolation (OPSI) system is established for precise, indexed isolation of individual bacterial, yeast or human-cancer cells. A controllable static flow field that acts as a cell pool is achieved in a microfluidics chip, to enable precise and ready screening of cells of 1 to 40 μm in size by bright-field, fluorescence, or Raman imaging. The target cell is then captured by a 1064 nm optical tweezer and deposited as one-cell-harboring nanoliter microdroplets in a one-cell-one-tube manner. For bacterial, yeast and human cells, OPSI achieves a >99.7% target-cell sorting purity and a 10-fold elevated speed of 10–20 cells per min. Moreover, OPSI-based one-cell RNA-seq of human cancer cells yields high quality and reproducible single-cell transcriptome profiles. The versatility, facileness, flexibility, modularized design, and low cost of OPSI suggest its broad applications for image-based sorting of target cells.
1. Introduction
Single-cell multi-omics have been transforming life sciences.1,2 Prior to the profiling of genomes, transcriptomes, proteomes or metabolomes for individual cells, a prerequisite is frequently the identification and acquisition of those single cells of target phenotypes. An image, of either bright field, fluorescence or Raman microspectroscopy, harbors rich phenotypic information to guide the selection of target cells for downstream omics profiling. Therefore, a real-time image-based device that sorts cells in a precisely indexed, “what you see is what you obtain” manner is a highly desirable and broadly applicable part of most single-cell analysis workflows. Moreover, desirable features of the device include: (i) versatility in cell size (i.e., from bacterial cells of 1 μm in size to human cells of several dozen μm in diameter), (ii) maintenance of cell vitality during operation, (iii) facileness and efficiency in operation, (iv) modularized design that allows extendibility to commercial microscopes or microspectrometers, and (v) relatively low cost in system and consumables.
Methods for real-time image-based cell-sorting have been under rapid development. Flow cytometry, which is based on either bright-field imaging, scattered light, or fluorescence, offers very high throughput.3–8 However, traditional flow cytometry can only handle low-resolution data for real-time data processing and cell sorting (e.g., 20 light scattering and fluorescence signals without spatial information), which greatly limited the sorting accuracy. For this, an intelligent image-activated cell sorter (IACS) is presented which employs a radically different data-management infrastructure and realizes real-time image-based intelligent cell interrogation and sorting at an unprecedented accuracy and rate.9,10 Apart from the sorting throughput, accurate and damage-free single cell isolation which involves considerations for sample preparation is also important especially for single cell genomics analysis. Flow cytometry usually has an uncertainty in precise single cell isolation, especially for those cells with a size of around 1 μm, and high flow shearing may affect cell viability during sorting. Dielectrophoresis array (DEP-array) integrates optical systems and dielectrophoresis, so as to realize real-time assessment of single-cell phenotypes in an array of cell pools and then reliable, at-will image-based single-cell isolation.11–14 As no shearing stream is induced, the integrity of sorted and isolated cells can be well preserved. However, DEP-arrays have been unable to sort those tiny cells of 1–5 μm in diameter (i.e., bacteria). Laser induced cell ejection (LICE) can sort and isolate individual bacterial cells in a label-free manner via a microscopic or microspectrospic image, but the large optothermal damage induced by the laser during cell ejection have either caused challenges in sorting large cells such as those from humans, or hindered the downstream single-cell culture or sequencing (in the case of Raman-based sorting).15,16
Optical tweezer-assisted single-cell sorting can potentially address these challenges. We have introduced Raman-activated gravity-driven encapsulation (RAGE) which combines optical tweezers and microfluidics to screen individual live human or bacterial cells via single-cell Raman spectroscopy (or bright-field or fluorescence imaging), package the target single-cell in a microdroplet, and then export it in a precisely indexed one-cell-one tube manner.17–19 The integration of droplet encapsulation and exportation allows a miniaturized amplification reaction volume (to the nanoliter range), which greatly reduces the amplification bias and improves the evenness of genome coverage for each single-cell. However, it is tedious to maintain a static aqueous phase in the microchannel which is important for the high accuracy of single-cell capture and encapsulation. Moreover, the relatively low throughput (1–2 cells per min) and tediousness of operation can limit its broader application.
To address these limitations, here we introduce an optical tweezer-assisted pool-screening and single-cell isolation (OPSI) device which combines a “pool-screening” design of a microfluidic chip and a 1064 nm optical tweezer for the trapping, manipulation, encapsulation, and export of individual cells with a wide size-range of 1 to 40 μm in diameter. By confining a pool of cells in a static chamber and imaging them in real-time by high-resolution fluorescence, bright field microscopy or Raman mapping, OPSI enables the sorting of cells in a precisely indexed, “what you see is what you obtain” manner. Tests on bacterial, yeast and human cells (1 μm to 40 μm in diameter) suggest a >99.7% target-cell sorting purity and a 10-fold improved sorting throughput of 10–20 cells per min. Moreover, OPSI-based one-cell RNA-seq of human MCF-7 cells reports high quality and reproducibility of single-cell transcriptome profiles. The versatility, facileness, flexibility and low cost of OPSI suggest its broad applications for image-based sorting of target cells.
2. Experimental procedures
2.1 Configuration of the optical tweezer system
The EasySort system (Qingdao Single-cell Biotechnology Ltd, China) was designed for single cell imaging, manipulation, and sorting. A 1064 nm continuous-wave diode-pumped laser (Changchun New Industries Optoelectronics Technology Co., Ltd, China) was employed as a light source of the optical tweezer for single cell manipulation (Fig. 1). The near-infrared wavelength of the selected laser can minimize the photodamage on trapped cells. The laser beam was expanded with a beam expander (3×, L1 and L2), and then focused with a 60× objective lens (Olympus 60×, NA 0.7) after a dichroic mirror (DM1) for single-cell trapping. To move and manipulate the trapped cells precisely, the microscope stage was mounted on an electric three-axis motorized translation stage with a travel range of 75 mm × 50 mm, resolution of 0.01 μm (smallest step size), and repeatability of <1 μm (bidirectional). An LED was used as a fluorescent light source, which went through a filter set (excitation filter EF and dichroic mirror DM2) before entering of the objective lens. Here, two filter sets were employed for the yellow and red fluorescence imaging of the sample using a CCD camera. A touch screen (10 inch) was integrated on the system for the real-time visualization of cell imaging and manipulation. To miniaturize the whole system, an integrated processing unit was built for the control of the CCD, optical tweezer, and microscope stage movement. The compact design can enable an arrangement in a biosafety cabinet to avoid contamination during sorting.
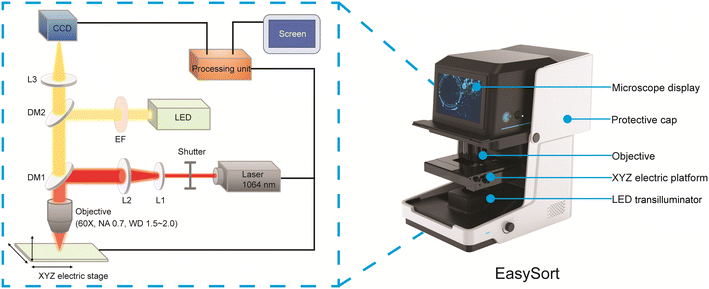 |
| Fig. 1 The optical path of EasySort. L1: plano-concave lens; L2: plano-convex lens; L3: lens; DM1, DM2: dichroic mirror; EF: excitation filter. | |
2.2 Fabrication of the OPSI microfluidic chip
An OPSI chip consists of a polydimethylsiloxane (PDMS) layer and a glass layer. Fabrication of the PDMS layer followed a soft photolithography protocol. Specifically, a ∼30 μm thick microstructure mold was photopatterned using a negative photoresist (MicroChem SU-83250) on a polished silicon wafer. A PDMS prepolymer with a curing agent mixed (10
:
1 ratio, Dow Corning SYLGARD 184) was poured onto the mold and baked at 65 °C for 2 h to ensure complete curing. The PDMS was peeled off from the mold, and into the PDMS, a through-hole of 1.0 mm in diameter was punched at the main-channel tip for inlet tube connection. For fluorescence sorting, the PDMS layer was bonded with a 1 mm thick glass slide by oxygen plasma. For Raman sorting, the PDMS layer was bonded with 0.15 mm thick high borosilicate glass.
2.3 Sample preparation and on-chip loading
E. coli cells (GFP labeled DH5a and ATCC25922) were cultured on Columbia blood agar plates at 37 °C with shaking overnight. Yeast cells were cultured on a YPD (yeast peptone dextrose) agar plate at 30 °C with shaking for 24 h. A single colony was inoculated into PBS solution and diluted to a concentration range of 106–108 cells per mL. MCF-7 cells (a human breast cancer cell line) were cultured in a Gibco RPMI 1640 medium (with 10 wt% FBS) and passaged every 2–3 d. The cells were collected and diluted to the concentration of 105–106 cells per mL with RNase-free PBS. A total of 0.2 wt% Tween-20 was added into suspensions to avoid cell adhesion in the chip. The inlet of the chip was sealed with adhesive tape, then the chip was placed in a glass desiccator with a vacuum pump connected (Agilent IDP-3) for 10 min vacuum treatment. Then, a drop of the cell suspension (0.5–10 μL) was added to the channel outlet in the oil well immediately after the chip was moved out from the desiccator. A pure water phase was connected to the inlet of the chip to wash the redundant cells in the channel out of the chip. Finally, the loaded chip was placed on the microscopic stage of EasySort. The chip inlet was connected to a syringe filled with pure water and hanged on a height adjustable sample holder. Mineral oil with 2% wt EM90 was added to the oil well for droplet generation.
2.4 Single-cell Raman imaging
A 60× objective lens (Olympus 60×, NA 0.7) was used for Raman signal acquisition and optical tweezers. A 532 nm laser was used for single cell Raman imaging due to its higher signal-to-noise ratio. Once trapped by the 532 nm laser (50 mW, pinhole 125 μm), the cell was typically exposed for 0.5 s for Raman signal acquisition. The Raman spectrum of a cell-free position inside the chip was also collected in triplicate, for background noise subtraction. All spectra underwent background noise subtraction, baseline correction and normalization processing using the Q-specs software (Qingdao Single-cell Biotech, China).
2.5 Single-cell sorting using OPSI
By adjusting the height of the sample holder, a balance between the oil phase and the water phase (driven by the capillary force and the liquid-level difference respectively) was readily established. The water–oil interface was kept at the tip of the channel near the oil well. Then, a 60× objective lens was used and the power of a 1064 nm laser was set to 150 mW (emergent power, before the shutter and lens) to capture the target single cell in the chamber and move it to the main channel. Then, the sample holder was elevated and then lowered to its original height, to generate several (>8) microdroplets in the oil well. The target individual cell was encapsulated among the droplets generated. Due to the lower-than-water density of mineral oil, the microdroplet could stay static at the bottom of the oil well, which can be readily transferred by inherent capillary force to the pipette tip within 1–2 seconds (low retention, maximum volume: 20 μL). Finally, the target cells transferred to tube in an indexed one-cell-one-tube style.
2.6 Single-cell RNA-seq library preparation and data analysis
After isolating single MCF-7 cells into each PCR tube, single-cell RNA-seq libraries were made using the MATQ-seq method following the published protocol, including cell lysis, reverse transcription, second-strand synthesis, cDNA amplification and purification, and stranded sequencing library preparation.20 The resulting libraries were sequenced using an Illumina NovaSeq 6000. For data analysis, raw reads were first trimmed by fastp version 0.12.4.21 MAQT-seq specific adapters and UMIs were cut by Cutadapt version 3.5.22 Clean reads were then mapped to the human reference genome assembly (“hg19”) using HISAT2 version 2.2.1.23 To eliminate the bias caused by PCR amplification, a customized script was used to filter the mapped reads based on UMI. Readcounts for each gene were determined using featureCounts version 2.0.3 with the default parameter.24
3. Results and discussion
3.1 Design principles of the OPSI microfluidic chip
To functionally sort and then isolate individual cells in a precisely indexed fashion, the OPSI platform includes an OPSI microfluidic chip for single-cell stream and an optical tweezer (via the EasySort system; Methods) for controlled trap-and-release of cells. For the OPSI microfluidic chip, the PDMS layer is plasma sealed with a smooth glass on the top (Fig. 2A). The top layer glass includes a round hole with a diameter of ∼6 mm: after being bound with PDMS, the hole is bottom sealed, and turned into an open well for oil storage which is directly connected to the microchannel on PDMS (Fig. 2B). As EasySort is an upright metallurgical microscopic system, the bottom set PDMS design can avoid the defocus caused by the uneven wall of the microchannel (Fig. S1†). A round cell chamber with ∼500 μm in diameter is set up, which is connected to the main channel with a side channel. For cell storage and imaging in chip, the cell chamber is surrounded with 7 side chambers that assist the vacuum-driven cell suspension filling. As the side chambers are filled with air after bonding with the glass substrate, after putting the chip in a vacuum desiccator for degassing with the inlet sealed, the surrounding side chambers would form a negative pressure for the porosity of PDMS, and then drive the cell suspensions to fully fill the cell chamber. (Fig. 2C and D). Then, a pure water phase is injected into the main channel, flowed linearly towards the oil well to wash out the residual cells (Fig. 2E). The suspension of washed-out cells left in the oil well is removed using a pipette. Then, the oil well is also washed with pure water several times to eliminate the residual cells completely to prevent contamination of the following sorting. After that, oil is added in the open well for single-cell droplet encapsulation (Fig. 2F).
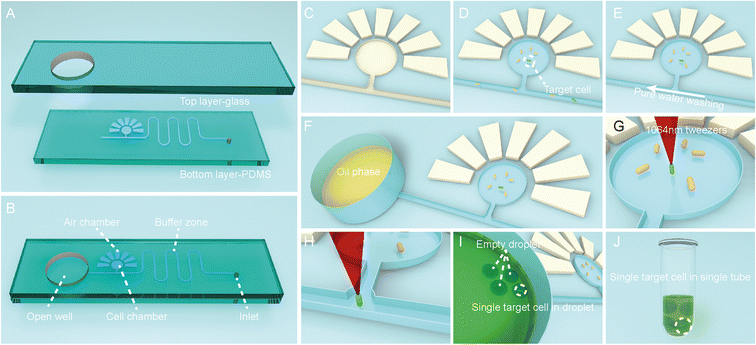 |
| Fig. 2 The scheme of the OPSI procedures. (A) the chip consists of an upper glass slide with a through-hole and a PDMS layer with the channel. (B) The structural sketch of the prepared chip. (C) Empty cell well. (D) Cell well is loaded with the cell suspension after vacuuming treatment. (E) Microchannel is washed with pure water after cell loading. (F) Adding oil in the open well. (G) Target single-cell screening and capture. (H) Target single-cell is moved to the microchannel using a 1064 nm optical tweezer. (I) Target single-cell is encapsulated in a droplet. (J) The droplets are transferred to a tube using a pipette tip. Notably, after its removal from vacuum, the degassed PDMS and air chambers (i.e., the seven ‘rays’ eradiated from the ‘sun’) would absorb gas from the environment, which creates a vacuum (in the cell chambers and channels) for sample loading. | |
For single-cell sorting and isolation, the target cells are screened in the hydrostatic cell chamber, then captured and moved to the microchannel by the optical tweezer individually (Fig. 2G and H). A 1064 nm laser is chosen here for its negligible photodamage on cells.25 Thus, the target single cell is flowed into the open well and encapsulated into a droplet (Fig. 2I). Due to the lower-than-water density of oil, the microdroplet stays static at the bottom of the open well near the channel, which can then be readily transferred by the inherent capillary force to the pipette tip and exported to a tube or well, in an indexed, one-cell-one-tube style.
Instead of the cytometry sorting strategy which detects individual cells one by one in a flowing stream, here the cells are screened whole staying together in a hydrostatic cell chamber, i.e., in a “pool-screening” strategy. As a result, the screening throughput is mainly dependent on the size of the microscopic view field. In addition, cytometry requires a strong fluorescent light source (usually a laser system) as the cells move rapidly in stream which result in a limited exposure time of typically a microsecond level for single cell imaging.26 The single-cell capture and manipulation precision can reach to a sub-micrometer level using an optical tweezer.27–29 Previously reported optical tweezer single-cell sorting strategies typically need to move a cell over a distance of several millimeters to centimeters for isolation. For example, in our previous RAGE system, a target cell needs to be moved ∼1 mm, from the cell chamber to the capillary channel tip, which usually takes ∼15 s. Here, the cell moving distance in OPSI is reduced to ∼140 μm (from the cell chamber to the channel); as a result, the shortened duration (shortened to 2–4 s) of laser capture improves the isolation throughput and minimizes the laser impact to cell vitality too. More importantly, the sorting procedures are also greatly simplified here. In the RAGE system, as the cell chamber and oil well are directly connected through the capillary channel, to ensure target cell purity, it is needed to sweep other unwanted cells out of the capillary channel one by one using the optical tweezer before target cell sorting. Frustrated, after every single-cell isolation, the unwanted cells will flow into the capillary channel again. In OPSI, as all the cells can be confined in the cell chamber, the above step can be omitted for which also improves the throughput.
3.2 Verification of the OPSI chip: sample loading
A complete filling of the microchamber on the OPSI chip with cells is required for subsequent single-cell sorting. The sample loading on chip is based on a self-loading mechanism that uses a degas driven flow to draw the cell suspension into the cell chambers as mentioned above. The vacuum inside the chip drives the cell suspension to fully fill the cell chamber within 2 min (Fig. 3A). The curving channel next to the cell chamber can prevent the cell suspension from entering the inlet (Fig. S2†). As the cell chamber is directly connected with the main channel, a diffusion-based exchange of solution takes place. Considering the distance (L) between the channel and farthest boundary of the chamber and the diffusion time scale t = L2/D (D stands for the diffusion constant), thorough exchange of solution from the chamber to the channel can be completed rapidly (within minutes). To prove this, amaranth red solution is injected into the main channel at 0.02 m s−1. Then, the amaranth solution fills the cell chambers through diffusion from the main channel in 10 min, indicating a quick interchange of solution between the cell chamber and main channel. Thus, the cell chamber can be regarded as a continuous culture vessel for on chip incubation. Compared to the cytometry strategy, which usually requires a minimal sample volume of tens of milliliters, 0.5 μL is sufficient to fill the cell chamber on the OPSI chip for subsequent sorting, representing a significant improvement.
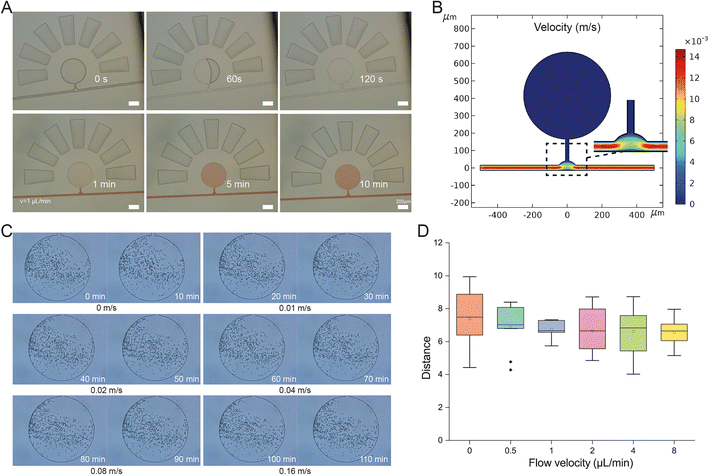 |
| Fig. 3 Vacuum-assisted sample loading and hydromechanics analysis of the chip. (A) The sample loading and diffusion between the cell chamber and main channel. (B) COMSOL analysis of flow velocity in the cell chamber and main channel. (C) PS bead movements at different flow rates of the main channel. (D) Distance moved by the beads in the cell chamber at different flow rates. | |
3.3 Verification of the OPSI chip: hydrodynamics
For tiny cells such as bacteria, one factor that confounds reliable indexing of cell capture is the disturbance of fluids by the flow in the main channel. To ensure all cells in the chambers can stay static in the chambers for sorting, we start with a COMSOL-based hydrodynamics simulation (Fig. 3B), which reveals a distinct difference in flow velocity between the main channel and cell chamber, i.e., cell suspensions in the cell chamber can maintain a stationary state when water flows quickly in the main channel. To experimentally validate the phenomenon, trajectory statistics of beads (∼5 μm in diameter) loaded in the cell chamber at various flow rates in the main channel is conducted. The movements of the particles in the chamber are time-lapse recorded every 10 min (Fig. 3C). Slight position changes of the particles are observed, mainly due to an inherent Brownian motion effect; however, no differences in moving distances are observed among the various main-channel flow rates, even at the fastest speed of 0.16 m s−1 (Fig. 3D). Moreover, no beads escaped from the cell chamber, indicating a high stability of the quiescent field in the chamber. To further validate the stability of the quiescent field in the chamber, the movements of live E. coli cells in the chambers are also recorded (Video S1†). At an inlet flow rate of 0.02 m s−1, no E. coli cells escaped from the chamber to the main channel for a duration of 10 min. Thus, the pooled-cell chamber can be regarded as a quiescent field well isolated from external disturbance, which ensures an optimal condition for target cell screening and manipulation.
3.4 Verification of the OPSI chip: droplet generation for single-cell encapsulation
Reliable, precise and indexed isolation of individual cells (particularly bacterial cells) relies on highly controlled and accurate encapsulation into one-cell microdroplets. In OPSI, the droplet encapsulation strategy ensures nanoliter-level single-cell isolation and easy chip-to-tube export of the one-cell droplet for downstream analysis (such as single-cell multiple displacement amplification (MDA)17,18), with a design that reduces bias and minimizes contamination.30–33 The droplet generation design is based on the Rayleigh–Plateau-type instability effect, which the droplets can be formed when a liquid issues from an orifice into another immiscible fluid.34,35 The driving force of pure water in the channel comes from a height difference between the liquid in chip and the hanged syringe (Fig. 4A). The syringe height is adjusted through a connected rocker. Thus, the number and formation-speed of droplets can be flexibly and readily controlled by adjusting the height of the syringe. When screening target cells in the cell chamber, the height of the syringe is adjusted to keep a balanced interference between the water and oil phases near the oil well (Fig. 4B). No droplets are generated until a target cell is found. For reliable single-cell encapsulation and export, droplets with uniform sizes are desirable. Assessment of the uniformity of droplet sizes through 100 continuously generated droplets reveals an average size of ∼82.4 μm in diameter, with a 5.9% coefficient of variance (C. V.; Fig. S3†), which ensures uniform encapsulation of single cells at the nanoliter level.
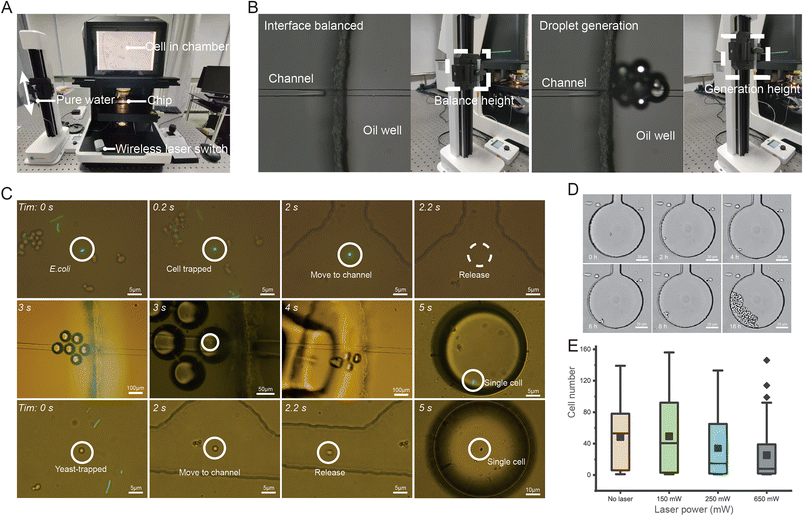 |
| Fig. 4 Platform and single-cell sorting. (A) EasySort platform ready for single-cell sorting. (B) The droplet generation can be precisely controlled by the gravity-driven sample injection system through tuning the height of the sample holder. (C) The single-cell sorting of artificial mock samples which contained E. coli and yeast. (D) 16 h culture of an individual yeast cell in the chamber. (E) Comparison of the number of cells in each chamber after 24 h on chip incubation under various laser power. | |
3.5 Application demonstration of the OPSI chip: fluorescence-activated sorting and export of E. coli and yeast cells
To test the performance of OPSI in sorting small cells (1–5 μm in diameter), an artificial mixture of E. coli and yeast was tested (Fig. 4C). Specifically, three types of cells including GFP-E. coli, non-GFP-E. coli and yeast cells are mixed in a ∼1
:
1
:
1 ratio and loaded on chip. GFP-E. coli and yeast single-cells are sorted and isolated through fluorescence and bright field microscopy. To prove that the OPSI also enables a precise isolation of rare cells from heterogeneous samples, an artificial mixed sample with only a 0.1% ratio of GFP-E. coli cells is also sorted here (Video S2†). The target cell with green fluorescence is quickly found in the cell chamber, and then captured and isolated. To ensure that the target cell is encapsulated, several droplets are generated as there is a distance between the cell chamber and oil well. The droplet generation number needed can be calculated by dividing the whole volume of the liquid from the cell chamber junction to the main-channel outlet by the mean volume of each droplet. Here, >8 droplets can ensure a 100% rate of target cell encapsulation.
The optical tweezer assisted accurate single-cell manipulation and droplet encapsulation strategy can ensure a 100% target cell collection. However, some unwanted cells can also sneak into the main channel from the cell chamber through self-swimming or Brownian movement and get collected with target cells together. A plate culture is conducted here to count the sneaked cell ratio for the sorting accuracy verification. Firstly, 100 μL droplets generated by water flowing out from the main channel (without sorting operations) are spread onto an agar culture plate for incubation. After 24 h, the number of visible colonies N0 are shown in Table 1. The ratio of unwanted cells, regarded as the sorting error R can be calculated through the following equations:
V1 is the volume of water spread on the agar culture plate (100 μL).
V2 is the combined volume of droplets required for only one target cell sorting and isolation. As the average diameter of droplets is ∼80 μm, ∼10 droplets are collected for one cell isolation, and thus,
V2 is 2.68 × 10
−3 μL, and
N is ∼37
![[thin space (1/6-em)]](https://www.rsc.org/images/entities/char_2009.gif)
300. Here,
N can be regarded as the sorting frequency when 100 μL droplets are collected. Thus, the ratio of unwanted cells in each sorting and isolation (
i.e., sorting error rate
R) can be obtained by dividing
N0 by
N. And the target-cell purity can be obtained by 100% minus error rate
R. A sorting purity of >99.7% (100% −
R) is achieved for the
E. coli and yeast samples of different concentrations (
Table 1). Theoretically, cells with higher mobility will induce a higher sorting error because it can get into the main channel more easily. As shown in
Table 1, the sorting of
E. coli cells has a higher sorting error than that of yeast cells, which is due to the
E. coli cells having a better swimming ability because of their inherent flagellar structures. In addition, a higher cell density in the chamber also leads to a higher chance of non-target cell collection.
Table 1 Plate culture-based verification of single-cell sorting accuracy over a wide range of cell concentrations. “Error rate”: the experimentally measured proportion of untargeted cells in an OPSI-based operation that sorts one target cell
Cell type |
Concentration in the chamber (cfu mL−1) |
Outlet concentration (cfu mL−1) |
Error rate R (  ) |
Mean |
SD |
E. coli
|
106 |
<10 |
— |
<0.268 |
107 |
13 |
10 |
0.349 |
108 |
107 |
28 |
2.87 |
109 |
992 |
80 |
26.6 |
Yeast |
107 |
<10 |
— |
<0.268 |
108 |
<10 |
— |
<0.268 |
3.6 Application demostration of the OPSI chip: single-cell culture for the cells sorted via bright-field imaging
To evaluate the optical tweezer effects on cell viability during sorting, on-chip single-cell culture of 1064 nm laser exposed yeast cells is conducted. An OPSI chip with multiple culture chambers of smaller sizes beside the main channel is fabricated (Fig. S4†). Individual cells can be confined in the culture chamber during incubation. When multiple cells get into one chamber, only one cell is retained for single-cell culture while others are moved out by the optical tweezer. The main channel is injected with continuously flowing fresh culture media (0.02 m s−1 flow rate, YPD), and thus, the nutrients in the cell chambers can be replenished via diffusion. To evaluate the photothermal effects of the optical tweezer on the viability of cells, the cells in each chamber are captured for 30 s under different laser power separately. The number of cells in each chamber is recorded after 24 h of incubation (Fig. 4D). As a 150 mW laser is already enough for the capture of cells with sizes of 1–30 μm in diameter, three groups (each contains 50 individual cells) irradiated under 150 mW to 650 mW power are obtained here. A control group without laser irradiation is also obtained for comparison. As shown in Fig. 4E, the cell numbers in the chambers after 24 h incubation exhibit an obvious variation, i.e., varying from 0 to 150. This may be due to the inherent heterogeneity among cells.36 Some cells show a remarkably higher proliferation rate than the others, while some cells show no proliferation even in the control group. By intergroup comparison, the control group has the highest median compared to the other laser exposed groups, indicating that the laser used here can induce an inhibition on cell growth, even though the 1064 nm wavelength is in the infrared region which is far from DNA absorption peaks in the UV region. However, the control group and 150 mW group have similar average cell numbers (54 in the control group; 40 in the 150 mW group), indicating that the photothermal damage from the laser below 150 mW here can be ignored for single-cell culture.
3.7 Application demonstration of the OPSI chip: Raman-activated single-cell sorting and culture
Single-cell Raman spectroscopy can provide an intrinsic chemical fingerprint of cells, making it possible to directly interrogate cellular compositions and identify species and physiological states in a label-free manner.37 Raman activated single-cell sorting (RACS) has become a new promising technology in functional screening and strain development of live microbial, plant and animal cell factories.38–41 However, the spontaneous Raman signal from one cell is inherently weak. A longer interrogation time (hundreds of microseconds to several seconds) is usually needed to obtain an acceptable spectrum. OPSI is an ideal platform for Raman activated single-cell sorting as it has no limitation on interrogation time. For the validation of RACS ability, carotenoid producing yeast cells are sorted from an artificial mixture (carotenoid producing and non-carotenoid producing S. cerevisiae cells are mixed in a ratio of ∼1
:
100). The carotenoids act as antioxidant agents inducing three obvious peaks near 996, 1143, and 1502 cm−1 (Fig. 5A). The spectra obtained from the original mixture are shown in Fig. 5B. Two kinds of yeasts can be easily distinguished by these characteristic peaks from the label-free single cell Raman spectra (SCRS). One target carotenoid yeast cell with characteristic SCRS is found in the mixture, and then sorted to a tube which contains YPD media for single-cell culture (Fig. 5C). After 24 h of incubation at 30 °C, an obvious orange suspension is found in the tube (Fig. 5D). To prove the sorting reliability, the SCRS of the cells cultured from the target cell are obtained and compared with that of the original mixture. Most of the SCRS exhibit carotenoid characteristic peaks (Fig. 5B), indicating a successful single-cell sorting and cultivation of the target carotenoid yeast cell. Compared with flow cytometry-based RACS,42–44 OPSI-RACS features a lower throughput but enables complete and sufficient Raman imaging without limitation on interrogation time, achieving a nearly 100% sorting accuracy.
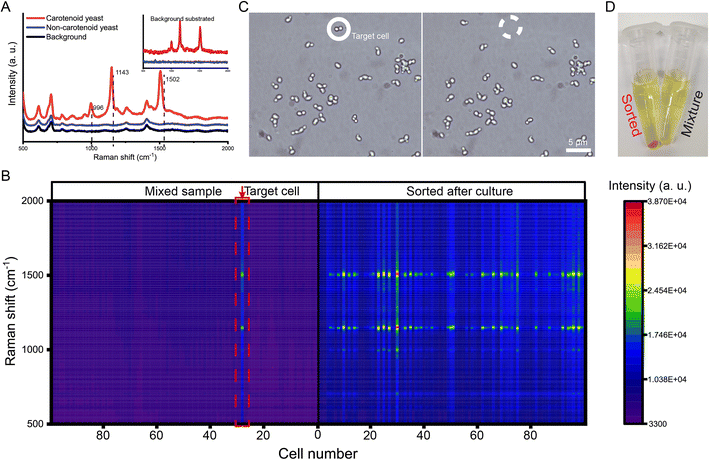 |
| Fig. 5 Raman-activated cell sorting of yeast via OPSI. (A) Single-cell Raman spectra obtained in chip from carotenoid yeast and non-carotenoid yeast. (B) Raman spectra comparison between the original mixed sample and cultured cells from sorted carotenoid yeast single-cells. (C) Target carotenoid yeast cell sorting. (D) Centrifuged products from the original artificial mixed sample and sorted carotenoid yeast cells after culture. | |
3.8 Application demonstration of the OPSI chip: single-cell RNA-seq of human MCF-7 cells sorted based on bright-field imaging
Besides microbes, the OPSI platform is also readily applicable to larger cells such as mammalian cells. Compared to existing strategies such as FACS and DEP-array (Table S1†), nanoliter-droplet encapsulation can be achieved here, which is favorable to downstream single-cell sequencing as it can reduce bias and avoid contamination.33,45–47 To further validate the viability and integrity of isolated cells and to extend the ability of the proposed system to the single-cell transcriptome level, we developed a novel OPSI-seq workflow, which combines our OPSI platform with single-cell RNA-seq library preparation (Fig. 6A). Single-cell RNA-seq methods such as 10× Genomics and SMART-based sequencing technologies have been widely adopted; however, these methods are not suitable for selectively sequencing target single-cells based on morphology. It is well known that cell morphology is critical in cell type identification and cell activity regulation.48 In OPSI-seq, each cell can be readily viewed and isolated based on their morphology on the image, with the quality ready for downstream single-cell RNA-seq library preparation (Fig. 6B). As a test, two single cells were isolated from the human MCF-7 cancer cell line (the s1 and s2 groups), and the total RNA was sequenced. One positive control sample that consists of three individual cells is included (the d1 group). Approximately 1.5–2 million reads were sequenced and mapped to the coding region for each sample (Fig. 6C). In single-cell RNA-seq analysis, the proportion of mitochondrial reads is one of the major criteria of sample quality control. Examination of our data reveals that the vast majority (about 90%) of the reads are mapped to non-mitochondrial genic regions, indicating the high quality of the scRNA-seq profiles. More than 15
000 genes are detected from each sample, with approximately 9000 of them being high quality genes (N mapped reads >10, Fig. 6D). The number of genes detected with the proposed workflow is much higher than that reported from the 10× Genomics,49 and comparable with the Smart-seq2/3 sequencing methods.50 A total of 6921 (∼70%) high quality genes are shared among all the sequencing samples, indicating the high reproducibility of the proposed workflow (Fig. 6E). In addition, the number of UMIs detected per gene is similar among all three samples (Fig. 6F), which further confirms the quality and reproducibility of the single-cell transcriptome profiles generated by the OPSI platform.
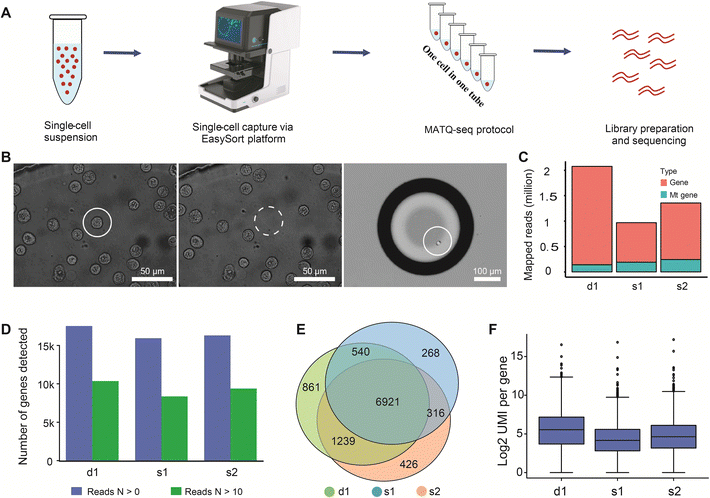 |
| Fig. 6 Single-cell RNA-seq of isolated cells from OPSI. (A) scRNA-seq workflow. (B) MCF-7 single cell isolation with the OPSI strategy. (C) Number of reads mapped to mitochondrial genes (green) and normal genes (red) in each sample. (D) Number of genes detected in each sample. (E) Number of shared genes among the 3 samples. (F) Distribution of UMI per gene (read coverage) in each sample. | |
4. Conclusion
In this work, an OPSI platform is established for bright-field imaging, fluorescence or Raman activated sorting of microbes, and offers several advantages over existing systems (Table S1†). Cells with a size range of 1–30 μm can be precisely isolated from groups and deposited as one-cell-harboring microdroplets in a one-cell-one-tube manner. Moreover, a loading volume as low as 0.5 μL allows the analysis of small-volume or rare samples. Furthermore, evaluation of optothermal damage on cells through single-cell cultures of yeast in chip suggests that a 1064 nm laser with a power of 150 mW would not induce a noticeable effect on cell viability. Briefly, the design of a static chamber for holding the cell pool, coupled with optical tweezer-assisted high-resolution bright field microscopy and Raman mapping, enables a real-time and accurate collection of single target cells at will. Specifically, GFP-labeled E. coli and carotenoid-yeast cells with ratios of 1/1000 and 1/100 are isolated with a >99.7% accuracy. Notably, the picoliter to nanoliter encapsulation and isolation ensures the high quality of single-cell RNA-seq: the number of genes detected with the OPSI-seq workflow is much higher than that typically reported using the 10× Genomics workflow,49 and is comparable with the Smart-seq2/3 sequencing methods.50 Taking advantage of the wide-field imaging rather than detecting single cells one by one in flowing stream, the recognition of target cells can be very fast; however, the isolation steps greatly limit the sorting throughput due to tedious manual single-cell capture and droplet collection. For this, efforts are made in the automation of cell recognition and manipulation to simplify the procedures. Nevertheless, OPSI, due to its versatility, facileness, flexibility, modularized design, and low cost, provides a generally applicable platform for phenotype-based single-cell sorting, sequencing and cultivation.
Author contributions
T. X. and Y. L. contributed equally to this work. T. X. designed the microfluidics system of OPSI and performed single-cell sorting. Y. L. designed the optical tweezer system EasySort. X. H. performed single-cell sorting and culture. L. K. optimized the optical path of EasySort. J. R. and L. S. conducted single-cell RNA-seq library preparation and data analysis. Z. D. conducted sample preparation. Y. J. and P. Z. integrated the EasySort system. J. X. and B. M. conceived the study. T. X., Y. L., L. S., B. M. and J. X. wrote the manuscript.
Conflicts of interest
There are no conflicts to declare.
Acknowledgements
This work was supported by the National Key R&D Program of China (2018YFA0902904), the Natural Science Foundation of Shandong Province, China (ZR2019PB022, ZR2020MD090, and 2021ZDSYS29), the National Center of Technology Innovation for Synthetic Biology (TSBICIP-PTJS-003-05), and the National Natural Science Foundation of China (32170103).
References
- P. C. Blainey and S. R. Quake, Nat. Methods, 2014, 11, 19–21 CrossRef CAS PubMed.
- R. Sandberg, Nat. Methods, 2014, 11, 22–24 CrossRef CAS PubMed.
- R. Stepanauskas and M. E. Sieracki, Proc. Natl. Acad. Sci. U. S. A., 2007, 104, 9052–9057 CrossRef CAS PubMed.
- N. Navin, J. Kendall, J. Troge, P. Andrews, L. Rodgers, J. McIndoo, K. Cook, A. Stepansky, D. Levy, D. Esposito, L. Muthuswamy, A. Krasnitz, W. R. McCombie, J. Hicks and M. Wigler, Nature, 2011, 472, 90–94 CrossRef CAS PubMed.
- M. Sesen and G. Whyte, Sci. Rep., 2020, 10, 8736–8749 CrossRef CAS PubMed.
- J. C. Baret, O. J. Miller, V. Taly, M. Ryckelynck, A. El-Harrak, L. Frenz, C. Rick, M. L. Samuels, J. B. Hutchison, J. J. Agresti, D. R. Link, D. A. Weitz and A. D. Griffiths, Lab Chip, 2009, 9, 1850–1858 RSC.
- D. Schraivogel, T. M. Kuhn, B. Rauscher, M. Rodriguez-Martinez, M. Paulsen, K. Owsley, A. Middlebrook, C. Tischer, B. Ramasz, D. Ordonez-Rueda, M. Dees, S. Cuylen-Haering, E. Diebold and L. M. Steinmetz, Science, 2022, 375, 315–320 CrossRef CAS PubMed.
- H. Cong, F. C. Loo, J. Chen, Y. Wang, S. K. Kong and H. P. Ho, Biosens. Bioelectron., 2019, 133, 236–242 CrossRef CAS PubMed.
- N. Nitta, T. Sugimura, A. Isozaki, H. Mikami, K. Hiraki, S. Sakuma, T. Iino, F. Arai, T. Endo, Y. Fujiwaki, H. Fukuzawa, M. Hase, T. Hayakawa, K. Hiramatsu, Y. Hoshino, M. Inaba, T. Ito, H. Karakawa, Y. Kasai, K. Koizumi, S. Lee, C. Lei, M. Li, T. Maeno, S. Matsusaka, D. Murakami, A. Nakagawa, Y. Oguchi, M. Oikawa, T. Ota, K. Shiba, H. Shintaku, Y. Shirasaki, K. Suga, Y. Suzuki, N. Suzuki, Y. Tanaka, H. Tezuka, C. Toyokawa, Y. Yalikun, M. Yamada, M. Yamagishi, T. Yamano, A. Yasumoto, Y. Yatomi, M. Yazawa, D. Di Carlo, Y. Hosokawa, S. Uemura, Y. Ozeki and K. Goda, Cell, 2018, 175, 266–276 CrossRef CAS PubMed.
- A. Isozaki, H. Mikami, H. Tezuka, H. Matsumura, K. Huang, M. Akamine, K. Hiramatsu, T. Iino, T. Ito, H. Karakawa, Y. Kasai, Y. Li, Y. Nakagawa, S. Ohnuki, T. Ota, Y. Qian, S. Sakuma, T. Sekiya, Y. Shirasaki, N. Suzuki, E. Tayyabi, T. Wakamiya, M. Xu, M. Yamagishi, H. Yan, Q. Yu, S. Yan, D. Yuan, W. Zhang, Y. Zhao, F. Arai, R. E. Campbell, C. Danelon, D. Di Carlo, K. Hiraki, Y. Hoshino, Y. Hosokawa, M. Inaba, A. Nakagawa, Y. Ohya, M. Oikawa, S. Uemura, Y. Ozeki, T. Sugimura, N. Nitta and K. Goda, Lab Chip, 2020, 20, 2263–2273 RSC.
- M. Werner-Klein, A. Grujovic, C. Irlbeck, M. Obradovic, M. Hoffmann, H. Koerkel-Qu, X. Lu, S. Treitschke, C. Kostler, C. Botteron, K. Weidele, C. Werno, B. Polzer, S. Kirsch, M. Guzvic, J. Warfsmann, K. Honarnejad, Z. Czyz, G. Feliciello, I. Blochberger, S. Grunewald, E. Schneider, G. Haunschild, N. Patwary, S. Guetter, S. Huber, B. Rack, N. Harbeck, S. Buchholz, P. Rummele, N. Heine, S. Rose-John and C. A. Klein, Nat. Commun., 2020, 11, 4977 CrossRef CAS PubMed.
- F. Chemi, D. G. Rothwell, N. McGranahan, S. Gulati, C. Abbosh, S. P. Pearce, C. Zhou, G. A. Wilson, M. Jamal-Hanjani, N. Birkbak, J. Pierce, C. S. Kim, S. Ferdous, D. J. Burt, D. Slane-Tan, F. Gomes, D. Moore, R. Shah, M. Al Bakir, C. Hiley, S. Veeriah, Y. Summers, P. Crosbie, S. Ward, B. Mesquita, M. Dynowski, D. Biswas, J. Tugwood, F. Blackhall, C. Miller, A. Hackshaw, G. Brady, C. Swanton, C. Dive and T. R. Consortium, Nat. Med., 2019, 25, 1534–1539 CrossRef CAS PubMed.
- M. Abonnenc, N. Manaresi, M. Borgatti, G. Medoro, E. Fabbri, A. Romani, L. Altomare, M. Tartagni, R. Rizzo, O. Baricordi, E. Tremante, E. Lo Monaco, P. Giacomini, R. Guerrieri and R. Gambari, Anal. Chem., 2013, 85, 8219–8224 CrossRef CAS PubMed.
- M. Hata, M. Suzuki and T. Yasukawa, Biosens. Bioelectron., 2022, 209, 114250 CrossRef CAS PubMed.
- X. Su, Y. Gong, H. Gou, X. Jing, T. Xu, X.-S. Zheng, R. Chen, B. Ma and J. Xu, Anal. Chem., 2020, 92, 8081–8089 CrossRef CAS PubMed.
- B. Hopp, Opt. Eng., 2012, 51, 014302 CrossRef.
- T. Xu, Y. Gong, X. Su, P. Zhu, J. Dai, J. Xu and B. Ma, Small, 2020, 16, e2001172 CrossRef.
- X. Jing, Y. Gong, T. Xu, Y. Meng and J. Xu, mSystems, 2021, e0018121 CrossRef.
- L. Min, Z. Pengfei, Z. Lei, G. Yanhai, W. Chen, S. Lu, W. Lili, C. Rongze, M. Yuli and F. Xiaoting, Clin. Chem., 2022, 68, 1064–1074 CrossRef PubMed.
- K. Sheng, W. Cao, Y. Niu, Q. Deng and C. Zong, Nat. Methods, 2017, 14, 267–270 CrossRef CAS PubMed.
- S. Chen, Y. Zhou, Y. Chen and G. Jia, Bioinformatics, 2018, 34, 884–890 CrossRef PubMed.
- M. Martin, EMBnet J., 2011, 17, 10–12 CrossRef.
- D. Kim, J. M. Paggi, C. Park, C. Bennett and S. L. Salzberg, Nat. Biotechnol., 2019, 37, 907–915 CrossRef CAS PubMed.
- L. Yang, G. K. Smyth and S. Wei, Bioinformatics, 2014, 30, 923–930 CrossRef PubMed.
- Z. Pilat, J. Jezek, M. Sery, M. Trtilek, L. Nedbal and P. Zemanek, J. Photochem. Photobiol., B, 2013, 121, 27–31 CrossRef CAS.
- H. Mikami, M. Kawaguchi, C. J. Huang, H. Matsumura, T. Sugimura, K. Huang, C. Lei, S. Ueno, T. Miura, T. Ito, K. Nagasawa, T. Maeno, H. Watarai, M. Yamagishi, S. Uemura, S. Ohnuki, Y. Ohya, H. Kurokawa, S. Matsusaka, C. W. Sun, Y. Ozeki and K. Goda, Nat. Commun., 2020, 11, 1162 CrossRef CAS PubMed.
- A. Blazquez-Castro, Micromachines, 2019, 10, 507 CrossRef PubMed.
- B. Landenberger, H. Hofemann, S. Wadle and A. Rohrbach, Lab Chip, 2012, 12, 3177–3183 RSC.
- H. Zhang and K. K. Liu, J. R. Soc., Interface, 2008, 5, 671–690 CrossRef CAS PubMed.
- J. Wang, H. C. Fan, B. Behr and S. R. Quake, Cell, 2012, 150, 402–412 CrossRef CAS PubMed.
- M. Yann, I. Thomas, R. S. Lasken, T. B. Stockwell, B. P. Walenz, A. L. Halpern, K. Y. Beeson, S. M. D. Goldberg and S. R. Quake, PLoS Genet., 2007, 3, 1702–1708 Search PubMed.
- J. Gole, A. Gore, A. Richards, Y. J. Chiu, H. L. Fung, D. Bushman, H. I. Chiang, J. Chun, Y. H. Lo and K. Zhang, Nat. Biotechnol., 2013, 31, 1126 CrossRef CAS PubMed.
- K. Leung, A. Klaus, B. K. Lin, E. Laks, J. Biele, D. Lai, A. Bashashati, Y. F. Huang, R. Aniba and M. Moksa, Proc. Natl. Acad. Sci. U. S. A., 2016, 113, 8484–8489 CrossRef CAS PubMed.
- H. Teng, C. M. Kinoshita and S. M. Masutani, Int. J. Multiphase Flow, 1995, 21, 129–136 CrossRef CAS.
- M. L. Eggersdorfer, H. Seybold, A. Ofner, D. A. Weitz and A. R. Studart, Proc. Natl. Acad. Sci. U. S. A., 2018, 115, 9479–9484 CrossRef CAS PubMed.
- Z. Guan, S. Jia, Z. Zhu, M. Zhang and C. J. Yang, Anal. Chem., 2014, 86, 2789–2797 CrossRef CAS PubMed.
- M. Li, J. Xu, M. Romero-Gonzalez, S. A. Banwart and W. E. Huang, Curr. Opin. Biotechnol., 2012, 23, 56–63 CrossRef CAS PubMed.
- S. Yan, J. Qiu, L. Guo, D. Li, D. Xu and Q. Liu, Appl. Microbiol. Biotechnol., 2021, 105, 1315–1331 CrossRef CAS PubMed.
- N. Nitta, T. Iino, A. Isozaki, M. Yamagishi, Y. Kitahama, S. Sakuma, Y. Suzuki, H. Tezuka, M. Oikawa, F. Arai, T. Asai, D. Deng, H. Fukuzawa, M. Hase, T. Hasunuma, T. Hayakawa, K. Hiraki, K. Hiramatsu, Y. Hoshino, M. Inaba, Y. Inoue, T. Ito, M. Kajikawa, H. Karakawa, Y. Kasai, Y. Kato, H. Kobayashi, C. Lei, S. Matsusaka, H. Mikami, A. Nakagawa, K. Numata, T. Ota, T. Sekiya, K. Shiba, Y. Shirasaki, N. Suzuki, S. Tanaka, S. Ueno, H. Watarai, T. Yamano, M. Yazawa, Y. Yonamine, D. Di Carlo, Y. Hosokawa, S. Uemura, T. Sugimura, Y. Ozeki and K. Goda, Nat. Commun., 2020, 11, 3452 CrossRef CAS PubMed.
- Y. Lyu, X. Yuan, A. Glidle, Y. Fu, H. Furusho, T. Yang and H. Yin, Lab Chip, 2020, 20, 4235–4245 RSC.
- K. S. Lee, F. C. Pereira, M. Palatinszky, L. Behrendt, U. Alcolombri, D. Berry, M. Wagner and R. Stocker, Nat. Protoc., 2020, 16, 634–676 CrossRef PubMed.
- K. S. Lee, M. Palatinszky, F. C. Pereira, J. Nguyen, V. I. Fernandez, A. J. Mueller, F. Menolascina, H. Daims, D. Berry, M. Wagner and R. Stocker, Nat. Microbiol., 2019, 4, 1035–1048 CrossRef CAS.
- X. Wang, Y. Xin, L. Ren, Z. Sun and B. Ma, Sci. Adv., 2020, 6, eabb3521 CrossRef CAS PubMed.
- P. Zhang, L. Ren, X. Zhang, Y. Shan, Y. Wang, Y. Ji, H. Yin, W. E. Huang, J. Xu and B. Ma, Anal. Chem., 2015, 87, 2282–2289 CrossRef CAS PubMed.
- Y. Marcy, T. Ishoey, R. S. Lasken, T. B. Stockwell, B. P. Walenz, A. L. Halpern, K. Y. Beeson, S. M. Goldberg and S. R. Quake, PLoS Genet., 2007, 3, 1702–1708 CAS.
- J. Gole, A. Gore, A. Richards, Y. J. Chiu, H. L. Fung, D. Bushman, H. I. Chiang, J. Chun, Y. H. Lo and K. Zhang, Nat. Biotechnol., 2013, 31, 1126–1132 CrossRef CAS PubMed.
- R. Stepanauskas, E. A. Fergusson, J. Brown, N. J. Poulton, B. Tupper, J. M. Labonte, E. D. Becraft, J. M. Brown, M. G. Pachiadaki, T. Povilaitis, B. P. Thompson, C. J. Mascena, W. K. Bellows and A. Lubys, Nat. Commun., 2017, 8, 84 CrossRef.
- H. M. Vergara, C. Pape, K. I. Meechan, V. Zinchenko and D. Arendt, Cell, 2021, 150, 402–412 Search PubMed.
- G. X. Zheng, J. M. Terry, P. Belgrader, P. Ryvkin, Z. W. Bent, R. Wilson, S. B. Ziraldo, T. D. Wheeler, G. P. McDermott, J. Zhu, M. T. Gregory, J. Shuga, L. Montesclaros, J. G. Underwood, D. A. Masquelier, S. Y. Nishimura, M. Schnall-Levin, P. W. Wyatt, C. M. Hindson, R. Bharadwaj, A. Wong, K. D. Ness, L. W. Beppu, H. J. Deeg, C. McFarland, K. R. Loeb, W. J. Valente, N. G. Ericson, E. A. Stevens, J. P. Radich, T. S. Mikkelsen, B. J. Hindson and J. H. Bielas, Nat. Commun., 2017, 8, 14049 CrossRef CAS PubMed.
- M. Hagemann-Jensen, C. Ziegenhain, P. Chen, D. Ramskld and R. Sandberg, Nat. Biotechnol., 2020, 38, 1123–1132 CrossRef PubMed.
|
This journal is © The Royal Society of Chemistry 2023 |
Click here to see how this site uses Cookies. View our privacy policy here.