DOI:
10.1039/D3RA00108C
(Paper)
RSC Adv., 2023,
13, 7939-7951
Lead-free 2D MASnBr3 and Ruddlesden–Popper BA2MASn2Br7 as light harvesting materials†
Received
6th January 2023
, Accepted 28th February 2023
First published on 9th March 2023
Abstract
We have explored the structural, electronic, charge transport, and optical properties of lead-free 2D hybrid halide perovskites, MASnBr3 and Ruddlesden–Popper perovskites, BAMASn2Br7 monolayers. Under density functional theory (DFT) calculation, we applied mechanical strain, i.e., tensile and compressive strain up to 10% in both cases. The mechanical strain engineering technique is useful for a tuned bandgap of 2D MASnBr3 and 2D BAMASn2Br7. The calculated carrier mobility for the electron is 404 cm2 V−1 s−1 and for the hole is up to 800 cm2 V−1 s−1 for MASnBr3. For BAMASn2Br7 the highest carrier mobility is up to 557 cm2 V−1 s−1 for electrons and up to 779 cm2 V−1 s−1 for the hole, which is 14% and 24% higher than the reported lead-iodide based perovskites, respectively. The calculated solar cell efficiency of 2D MASnBr3 is 23.46%, which is 18% higher than the reported lead-based perovskites. Furthermore, the optical activity of the 2D MASnBr3 and 2D BAMASn2Br7 shows a high static dielectric constant of 2.48 and 2.14, respectively. This is useful to show nanodevice performance. Also, 2D MASNBr3 shows a high absorption coefficient of 15.25 × 105 cm−1 and 2D BAMASn2Br7 shows an absorption coefficient of up to 13.38 × 105 cm−1. Therefore our theoretical results suggest that the systems are under mechanical strain engineering. This is convenient for experimentalists to improve the performance of the 2D perovskites. The study supports these materials as good candidates for photovoltaic and optoelectronic device applications.
1. Introduction
Nowadays, energy crises and environmental pollution are the main big problems for humans. Many research groups are working on finding alternative solutions for these problems. Globally, educational and industrial purpose scientific communities are now attracted to renewable energy sources, and have developed new technology to overcome such situations and find low-cost solutions. The research community has recently become interested in organic–inorganic hybrid halide perovskites because of their rapid advancement in photovoltaics and optoelectronics over the past ten years.1 Compared with traditional silicon cells, these hybrid halide perovskites solar cells reached a power conversion efficiency (PCE) of more than 24% within a few years.1–4 Their electronic properties include a high extinction coefficient, tunable bandgaps, long carrier mobility, and a long charge transport diffusion path.5–8 However, with these advantages, chemical instability is the central issue. These hybrid halide perovskites being highly reactive with moisture is the main drawback for industrial purposes.9–12
Many technologies have developed and extracted the two-dimensional 2D slab by slicing from the 3D framework of hybrid halide perovskites.13–15 The transformation of 3D to 2D perovskite opens the potential to more clearly analyze electronic, optical, and transport properties.16 These 2D hybrid halide perovskites are a stable candidate for optoelectronic devices. Like light-emitting diodes,17 photocatalysts, field-effect transistors,17–19 lasers, etc.20–25 Also, these 2D perovskites have flexible structural and compositional properties. Long-term stability characteristics in ambient conditions, more moisture resistance than 3D structure, and tunable photovoltaic properties.26–30 2D third-generation hybrid halide perovskites have the structural formula MAZX3 (ref. 31–34) (MA = CH3NH3, Z = Pb, Sn and X = Cl, Br, I). These 2D perovskites interact well with inorganic layers, same as Ruddlesden–Popper 2D structures,35–40 which has structural formula (BA)2MAZ2X7 (BA = CH3(CH2)3NH3, MA = CH3NH3, Z = Pb, Sn, X = Cl, Br, I). Due to the structural flexibility of 2D perovskites, many groups have developed a new technique to find its efficient power conversion efficiency (PCE). 2D material interfacing with 2D perovskites has reached PCE 12.6%.12,15,41 2D lead halide CH3NH3PbI3 and Ruddlesden–Popper (CH3(CH2)3NH3)2CH3NH3Pb2I7 have reached 14.9%, 17%, and 19.7% PCE in theoretical experiments, respectively.42–44 Yang et al.'s group reached 20% PCE by interfacing 2D with 3D perovskites.45 Under condition Shockley–Queisser limit, PCE of perovskites was achieved 31% with the theoretical study.46 The 2D lead (Pb) hybrid halide perovskites have been considered good candidates as light-harvesting materials. But has toxicity issues with environmental effects.47 To solve the problem of environmentally and humanly hazardous hybrid halide perovskites based on lead (Pb). Researchers have discovered that substituting alternative divalent elements, such as In, Sn, Sb, Bi, etc., for lead is advantageous for both the environment and human beings. Experimentally, the tin (Sn) based halide perovskites are isostructural compounds of Pb-based halide.31 Sn-based halide perovskites materials contain a narrow optical bandgap high optical absorption coefficient. Also, it contains high charge carrier mobility with low exiting binding energy and good stability.48–50 Experimentally, the tin-based halide materials work as a light-harvesting material covering a wide range of visible spectrums. Furthermore, we have found that many groups are working on lead-free tin-based hybrid halide perovskites. The Xu et al. group is tuning the optical band gap of perovskites for light harvester materials.51 For thermoelectric devices, these materials were used.52 Ruddlesden–Popper nonlinear band behaviour due to mismatch s-states of Pb and Sn design a metal alloy.36 As the number of 2D layers increases band, behaviour shows charge carries effect well compared with experimental result.53 Therefore, these studies are useful for developing new building blocks for future photovoltaic and optoelectronic devices.54–62
In this paper, we give a comparative analysis of two orthorhombic structures of hybrid halide perovskites using density functional theory (DFT) calculations. CH3NH3SnBr3 as (MASnBr3) (MA = CH3NH3) and Ruddlesden–Popper (CH3(CH2)3NH3)2CH3NH3Sn2Br7 as (BAMASn2Br7) (BA = (CH3(CH2)3NH3)2, MA = CH3NH3) perovskites with their structural, electronic, optical and transport properties. Under mechanical strain conditions, we apply both tensile and compressive strain up to 10%. Mechanical strain condition is a valuable way to tune the electronic and optical properties of 2D halide perovskites because of the flexible structural and composition properties. For the creation of lead-free 2D hybrid halide perovskites for optoelectronic and photovoltaic applications, mechanical strain engineering offers a novel route.
2. Methodology
Density functional theory (DFT) and the Vienna ab initio simulation package (VASP) are used for all structural and electrical calculations.63–66 The projected augmented wave (PAW) method used the generalized gradient approximation (GGA) exchange–correlation Perdew–Burke–Ernzerhof (PBE) functional.66 The van der Waals (vdW) is included in our calculations. We also include SOC and HSE calculation to find more clear results.67 The plane-wave kinetic energy cut-off is set to 500 eV. The Brillouin zone (BZ) k-point grid (5 × 5 × 1) was used for structure optimisation. Also (15 × 15 × 1) k-point grid was selected for further electronic properties. All atomic structures of MASnBr3 (MA = CH3NH3) and Ruddlesden–Popper (BA)2MASn2Br7 (BA = CH3(CH2)3NH3, MA = CH3NH3) are converged up to the force 0.0001 eV Å−1 and total energy at 10−4 eV. To define structural balance, we used the ionic radii based tolerance factor formula t = (RA + RX)/√2(RB + RX) should be in the range between 0.813 and 1.107.5 Optical properties can be estimated through the Kramers–Kronig transformation based on the electronic structure.68–71 The optical absorption coefficient can be calculated using the formula: |
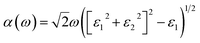 | (1) |
3. Results and discussions
3.1 Structural and electronic properties
Here, we have studied the structural parameter of hybrid halide MASnBr3 and BAMASn2Br7 monolayer, as shown in Fig. 1(a) and 2(a). The lattice parameters exhibit good agreement with those that have been previously reported, as shown in Table 1. We have not found much research on BAMASn2Br7 monolayers during the literature survey. Here we replaced lead (Pb) with tin (Sn),41,43 which has an ionic radius smaller than Pb and the ionic radius of halogen iodide (I) replaced by bromine (Br). The corresponding lattice parameters are of the 2D MASnBr3, and BAMASn2Br7 monolayer smaller respectively as lead based 2D perovskites.10,41,43
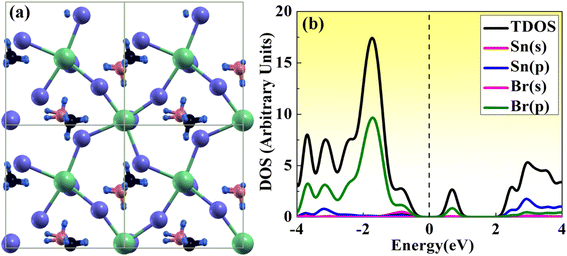 |
| Fig. 1 (a) Atomic structure of MASnBr3, where Sn, Br, C, N, H atom are represented in green, violet, black, dark pink, sky blue respectively. (b) Calculated total (TDOS) and projected density of states (PDOS) of MASnBr3. | |
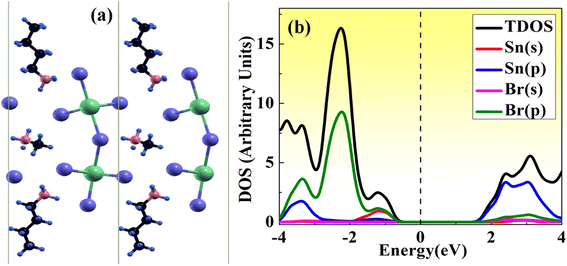 |
| Fig. 2 (a) Atomic structure of BAMASn2Br7, where Sn, Br, C, N, H atom are represented in green, violet, black, dark pink, sky blue respectively. (b) Calculated total (TDOS) and projected density of states (PDOS) of BAMASn2Br7. | |
Table 1 Calculated lattice parameter of MASnBr3 and BAMASn2Br7 monolayer
|
a (Å) |
b (Å) |
Other |
MASnBr3 |
8.08 |
8.5 |
8.5 (ref. 54 and 55) |
BAMASn2Br7 |
7.20 |
5.21 |
6.0 (ref. 57) |
With all these replacements, Sn and Br perovskites structure becomes more stable and does not have much change in the structural phase. Also, for both structures corresponding tolerance factor is in the range of 0.8 < t < 1.0, with consistence formation energy 141 eV for MASnBr3 and 196 eV for BAMASn2Br7.
Furthermore, we have calculated the total density of states (TDOS) and projected density of states (PDOS) of MASnBr3 and BAMASn2Br7 in Fig. 1(b) and 2(b). In Fig. 1(b) it is clearly seen that in the valence band of MASnBr3, most of the part is contributed with the Br-p states with Sn-s states. While in the conduction band, most of the part is covered by the Sn-p states. Similarly, in the case of the Ruddlesden–Popper BAMASn2Br7 perovskites, most of the part valence band is contributed with the Sn-s and Br-p states. The conduction band is coved with the Sn-p states as shown in Fig. 2(b). Also, in both systems, null contributions of organic molecules in valence and conduction bands are observed. In Fig. 3 and 4 shows the calculated band structure of the MASnBr3 and BAMASn2Br7. Fig. 3(a) contain the band structure of MASnBr3 with PBE (without SOC) and Fig. 3(b) with SOC. The calculated indirect bandgap is along with gamma to x point 1.14 eV. Which is consistent with previously reported results.52
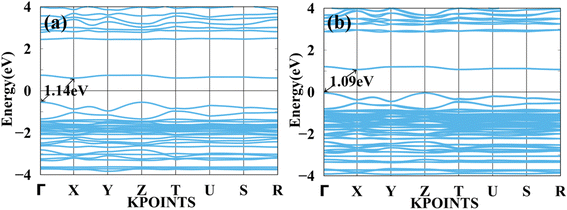 |
| Fig. 3 Calculated band structure of MASnBr3 with (a) PBE and (b) spin orbit coupling (SOC) represented. | |
In Fig. 4(a) shows a bandgap of BAMASn2Br7 PBE (without SOC) and Fig. 4(b) with SOC. Here, the case of Ruddlesden–Popper perovskite BAMASn2Br7 indirect bandgap is at Z to Y point 2.85 eV. BAMASn2Br7 perovskites have not been much studied, but compared with related Ruddlesden–Popper are like BAMASn2I7 consistent.36,43,58,59 Also, when we replace Pb with Sn and iodine by Br, corresponding lattice parameters decrease with increasing corresponding band gaps.41 In both cases, we have seen that under the SOC study, there is not much effective change in indirect bandgap observed.5,41,43 An orbital contribution point of view in valence band maxima (VBM) is mostly contributed by the Sn-5s and Br-4p orbitals. While conduction band minima, most of the part is contributed with the Sn-5p and 4 s, 4p orbitals of Br atom.
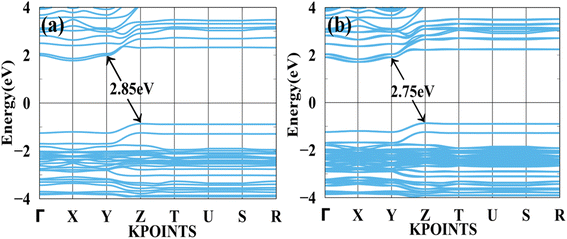 |
| Fig. 4 Calculated band structure of BAMASn2Br7 with (a) PBE and (b) spin orbit coupling (SOC) represented. | |
3.2 Strain engineering
We applied mechanical strain to both systems to moderate electronic properties as shown in Fig. 5 and 6 for MASnBr3, Fig. 7 and 8 for BAMASn2Br7. Due to flexible structural and compositional properties, mechanical strain is an efficient way to tune electronic properties.5,41 As per literature survey, we found that there are many experimental as well as theoretical techniques have been developed to improve only performance of the system or device. In the literature survey we found that there are no other studies on strain dependent on 2D MASnBr3 and Ruddlesden–Popper BA2MASn2Br7 orthorhombic structures. Lattice strain is promising technique to improve performance of the many 2D materials. This has been useful for both experimental as well as theoretical groups. As per experimental researches studies of hetero epitaxial perovskite thin films shows that lattice strain play an important role to determining the physical properties of the thin films.5,41,60,61 In the case of the MASnBr3 system, we have applied both tensile and compressive strain along the x and y directions from 1% to 10% in the interval of 2%. Under tensile strain, condition bandgap was consistently decreased from 1% to 10% from 1.12 eV to 0.93 eV, as shown in Fig. 5. Similarly, compressive strain band gap decreases consistently 1% to 10% from 1.12 eV to 0.95 eV as shown in Fig. 6. Compared with the unstrained system with both tensile and compressive strain systems, the bandgap was decreased because the valence band contains most of the part of antibonding states of Br-p and Sn-s orbitals. In comparison, the conduction band contains most of the non-antibonding states of Br-p and Sn-p orbitals. Therefore near the Fermi level, downward shifting of conduction bands and upward shifting of valence band occurred effectively.
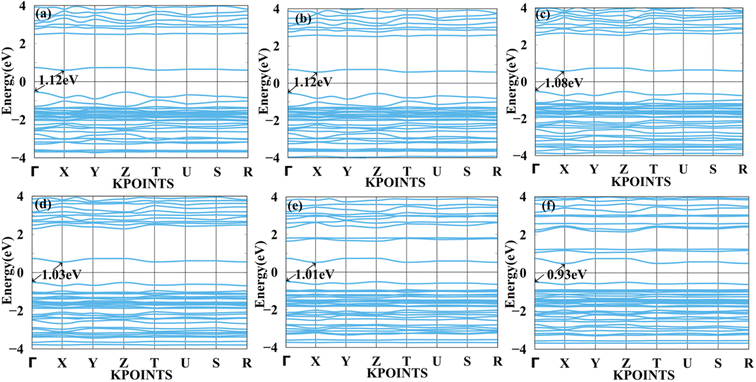 |
| Fig. 5 Calculated tensile strain band structure of MASnBr3 with (a) 1%, (b) 2%, (c) 4%, (d) 6%, (e) 8% and (f) 10% respectively. | |
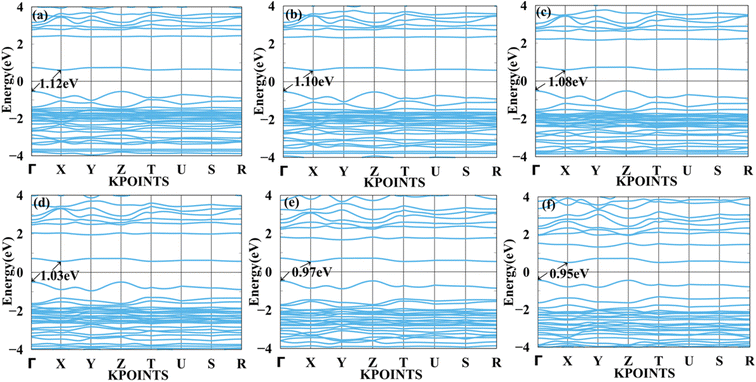 |
| Fig. 6 Calculated compressive strain band structure of MASnBr3 with (a) 1%, (b) 2%, (c) 4%, (d) 6%, (e) 8% and (f) 10% respectively. | |
Fig. 7 and 8 show the tensile and compressive strain-dependent band structure of BAMASn2Br7 perovskites. Here, under conditions of tensile strain, bandgap reduces as strain is increased by 1%, 2%, 4%, 6%, or 8%, going from 2.84 eV to 2.792 eV to 2.70 eV to 2.61 eV to 2.50 eV. Similarly, in compressive strain conditions, band gaps decrease from 1% to 8% to 2.84 eV to 1.96 eV. At 10% both types of strain show metallic behaviour. That means the sustainability of the structure is up to 8%. Further, in Ruddlesden–Popper BAMASn2Br7. Comparison between the unstrained systems with strain systems of nature of the bandgap varies. Due to the presence of antibonding states of Br-p and Sn-s orbitals in valence bands. Non-antibonding states of Br-p and Sn-p orbital's in conduction bands are similar as that of the MASnBr3 perovskites. Overall, the mechanical strain technique is useful for tuning the bandgap and open the path for experimentalists to study thin films' physical and chemical properties.5,60
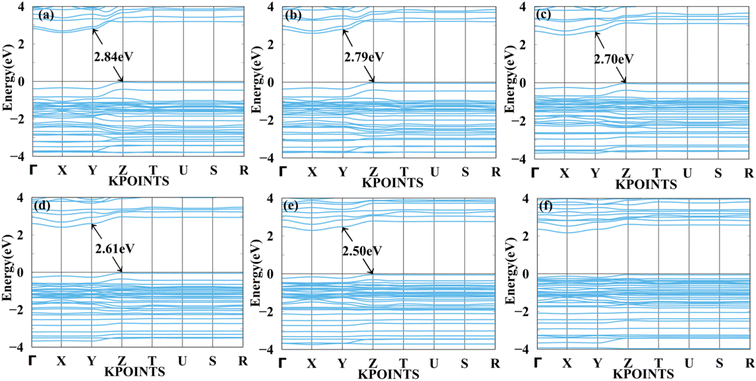 |
| Fig. 7 Calculated tensile strain band structure of BAMASn2Br7 with (a) 1%, (b) 2%, (c) 4%, (d) 6%, (e) 8% and (f) 10% respectively. | |
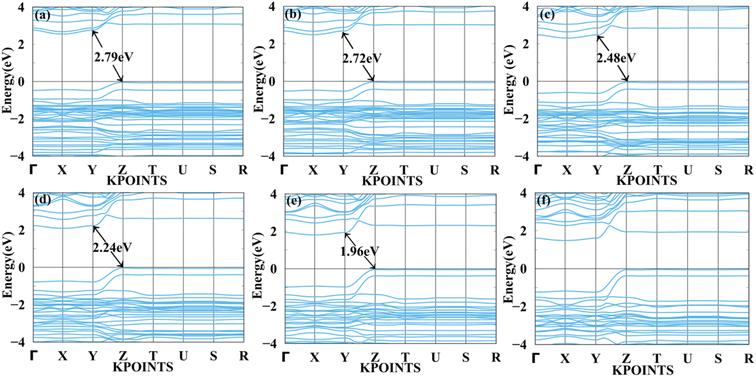 |
| Fig. 8 Calculated compressive strain band structure of BAMASn2Br7 with (a) 1%, (b) 2%, (c) 4%, (d) 6%, (e) 8% and (f) 10% respectively. | |
Using the Hellmann–Feynman theorem61 In Fig. 9 and 10 we have calculated stress on both systems. In both cases, we applied strain on the overall system along the x-axis and y-axis for MASnBr3 x-axis and z-axis for BAMASn2Br7. Overall stress was increased from 1% to 10%. Compared with tensile and compressive strain, in both systems, compressive stress is more and tensile stress is less. This change is happened due to contractions and expansion of lattice parameters of the system as shown in 2D barplot in Fig. S1.† Which effect the inter-bonding activity of the atoms. In the case of the MASnBr3 system, the structure's sustainability is up to 10% in both tensile and compressive. While in the case of BAMASn2Br7, the sustainability of the strain is up to 8%. After that, both systems show metallic nature.
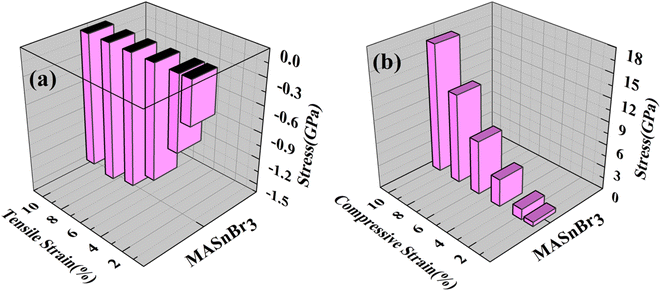 |
| Fig. 9 Applied (a) tensile and (b) compressive strain versus stress for the MASnBr3. | |
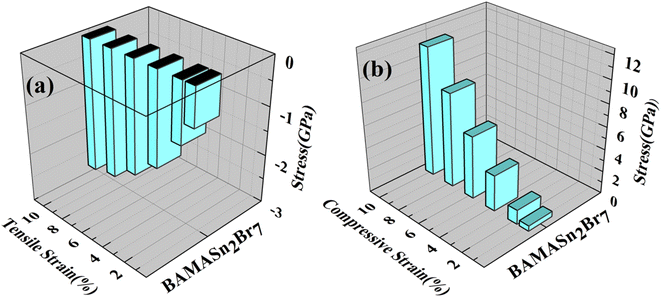 |
| Fig. 10 Applied (a) tensile and (b) compressive strain versus stress to the BAMASn2Br7. | |
3.3 Charge carrier mobility
Further, we have a focus on the charge transport properties of both systems. Due to flexible structural and compositional properties, these systems are well sustained in strain conditions, bands experience a high degree of dispersion and have a bandgap near optical band gaps.51 Near the Fermi level, the directional dependent effective mass of both electrons and holes with the slope of x, y, z-axis direction are shown in Table 2. The effective mass of the electron and holes for MASnBr3 along x and y directions are 0.36 to 0.057 and for Ruddlesden–Popper BAMASn2Br7 along x and z-direction are 0.33 to 0.146. In both cases, the effective mass of electrons and holes is remarkably more than the previously reported.35,43,62
Table 2 Calculated effective mass, carrier mobility, and relaxation time of MASnBr3 and BAMASn2Br7 respectively
System |

|

|
C (N m−1) |
μe (cm2 V−1 s−1) |
μh (cm2 V−1 s−1) |
τe (fs) |
τh (fs) |
MASnBr3 |
X |
0.122 |
0.0578 |
19.17 |
248.0 |
181.6 |
30.25 |
10.49 |
Y |
0.361 |
0.0633 |
19.17 |
404.2 |
800 |
146 |
50.64 |
BAMASn2Br7 |
X |
0.160 |
0.336 |
7.97 |
557 |
124.1 |
89.34 |
41.69 |
Z |
0.146 |
0.320 |
7.97 |
367.4 |
779 |
53.64 |
249 |
We also calculate the charge transport carrier mobility and relaxation time using deformation potential theory. Which leads to calculate using formula
|
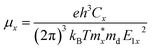 | (2) |
Also carrier mobility dependent relaxation time (τ), τ = (μm*)/e as shown in Table 2. Here e stand for electron charge, h for Planck's constant, m* for effective mass
, E1x = ΔV/(Δax/ax) deformation potentials.5 In the case of MASnBr3, the calculated carrier mobility of holes is higher than the electrons. Similar trends were observed in BAMASn2Br7, the hole carrier mobility is higher than the electrons. In compared with lead-iodide-based perovskites, carrier mobility of Sn-bromide-based perovskite is 14% and 24% higher, which are quite larger than the reported experimental and theoretical data.5,35,41,43,62 This anisotropic nature of carrier mobility is due to smaller effective mass and flexible structural properties, affecting the band gaps. This high carrier mobility leads to better device performance. Open a new path for experimentalists to find solar cell efficiency and developed optoelectronic devices.
3.4 Optical properties
Here, both perovskites structures have flexible electronic and structural properties, which is useful for determining their optical behaviour in both tensile and compressive strains. Using frequency-dependent Kramer–Kronig relation developed by Drude model.70,71 We are focused on optical properties in Fig. 11 and 12 shown in MASnBr3 under tensile and compressive strains, while in Fig. 13 and 14 shows optical properties of BAMASn2Br7 under mechanical strain conditions.
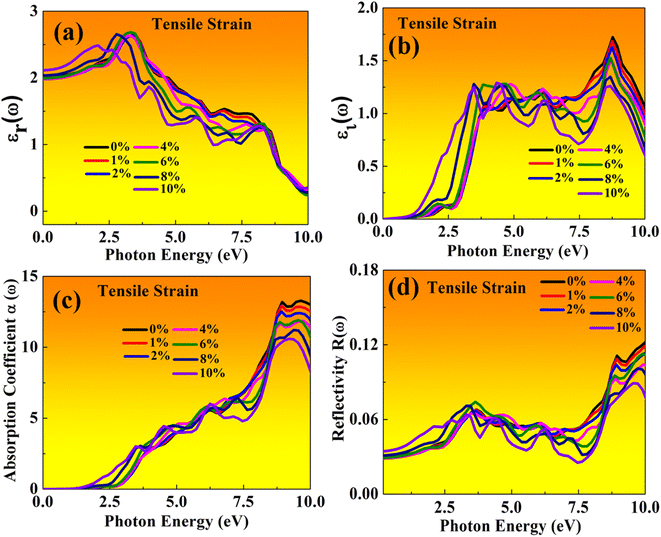 |
| Fig. 11 Optical properties of MASnBr3 perovskites represented with tensile strain: (a) real εr(ω), (b) imaginary εi(ω), (c) absorption coefficient, (d) reflectivity. | |
In the real part of the MASnBr3, the static dielectric constant is 2.011. Further, increases in tensile strain the static dielectric constant from 1.99 to 2.11. In compressive strain from 2.02 to 2.48 for 1% to 10%, as shown in Fig. 11(a) and 12(a), respectively. In the case Ruddlesden–Popper BAMASn2Br7 optical properties, the real part static dielectric constant is 2.01. Under mechanical strain conditions, the static dielectric constant decreases in tensile strain from 2.01 to 1.98 from 1% to 10%, while in the case of compressive strain static dielectric constant are increases from 1.9 to 2.14 from 1% to 10% as shown in Fig. 13(a) and 14(a) respectively. These high values of static dielectric constant are useful to define optoelectronic device performance. Similarly, in both cases, the imaginary part of static dielectric constant the optical activity is due to the transition of the electron in valence band Sn-s states to Br-p states in the conduction band.
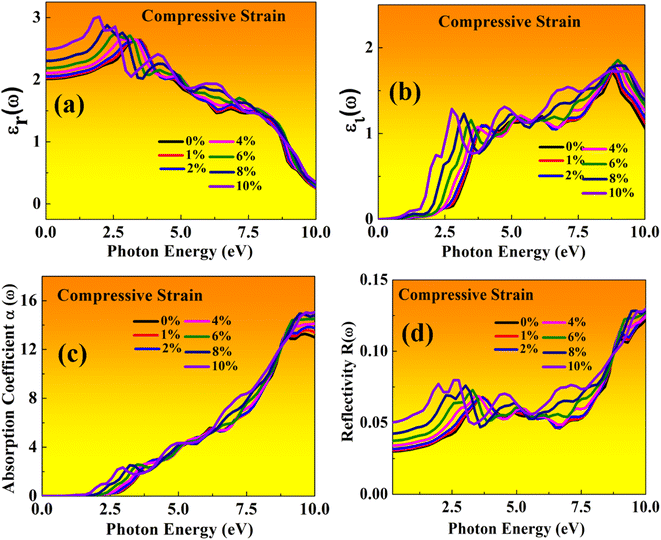 |
| Fig. 12 Optical properties of MASnBr3 perovskites represented with compressive strain: (a) real εr(ω), (b) imaginary εi(ω), (c) absorption coefficient, (d) reflectivity. | |
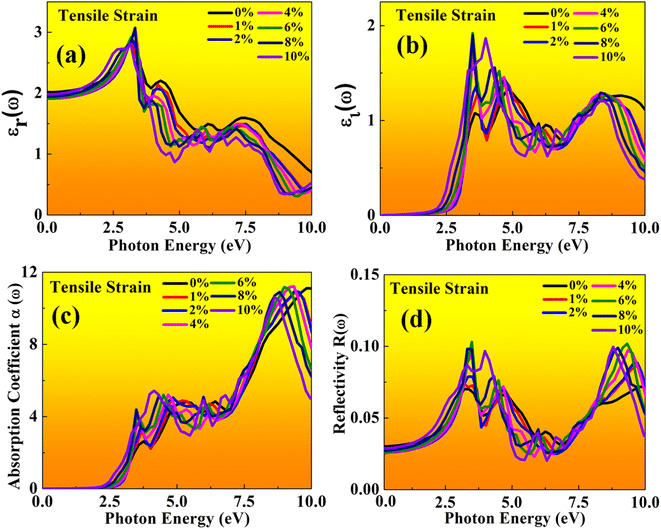 |
| Fig. 13 Optical properties of BAMASn2Br7 perovskites represented with tensile strain: (a) real εr(ω), (b) imaginary εi(ω), (c) absorption coefficient, (d) reflectivity. | |
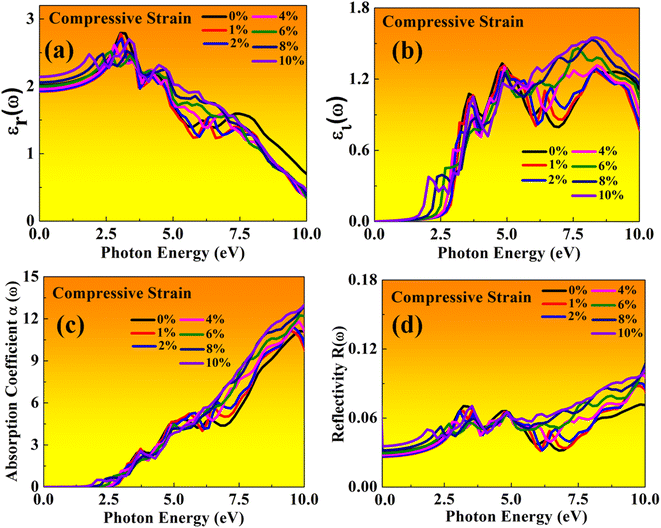 |
| Fig. 14 Optical properties of BAMASn2Br7 perovskites represented with compressive strain: (a) real εr(ω), (b) imaginary εi(ω), (c) absorption coefficient, (d) reflectivity. | |
In both cases, the first peak is at 2.5 eV. After applying both tensile and compressive strain, optical activity orientation is a move towards high energy part 8 eV as shown in Fig. 11(b) and 12(b) for MASnBr3, Fig. 13(b), 14(b) for BAMASn2Br7. Further, optical properties are analyzed by absorption coefficient, reflectivity. In the case of MASnBr3 absorption coefficient is at 13.27 × 105 cm−1 at 8.8 eV. Which are further decreases from 12.91 × 105 cm−1 to 10.58 × 105 cm−1 in tensile strain from 1% to 10% as shown in Fig. 11(c). In case compressive strain absorption coefficient increases from 13.60 × 105 cm−1 to 15.25 × 105 cm−1 for 1% to 10% as shown in Fig. 12(c). Also, in the case of BAMASn2Br7 absorption coefficient is 11.11 × 105 cm−1 for the unstrained system. For tensile strain it is decreases from 10.91 × 105 cm−1 to 10.62 × 105 cm−1 for 1% to 10% as shown in Fig. 13(c) and in case of compressive strain it is increases from 11.14 × 105 cm−1 to 13.38 × 105 cm−1 for 1% to 10% as shown in Fig. 14(c). The first small peak orientation is near 2.5 eV, which shows optical band gap activity is in the range of previously reported data.33,35,47 Overall, the optical activity of MASnBr3 and BAMASn2Br7 are shown. By reflectivity by the orientation of peaks at 0 eV to 2.5 eV and 2.5 eV to 5 eV, and further 10 eV is in the visible (500–414 nm) as well as ultraviolet region(310–177 nm) of the energy spectrum as shown if Fig. 11(d), 12(d), 13(d) and 14(d). Therefore maximum light reflects in the visible as well as in the ultraviolet region. These studies are consistent with the previously reported experimental and theoretical studies.33,35,47 Overall, compared with MASnBr3 and BAMASn2Br7 perovskites with other 2D hybrid halide perovskites. Tunable band gap and optical, charge transport activity useful for photovoltaic and solar cell devices.
3.5 Solar cell efficiency parameter
Further, we have investigated the solar cell efficiency performance of 2D MASnBr3. The Shockley and Queisser (SQ) model depend on the material's bandgap. To calculate the performance of solar cell efficiency, the band gap are required to be in between 1.0 to 1.7 eV. Therefore, the bandgap of 2D MASnBr3 is under SQ limit and band gap of BAMASn2Br7 are higher than the SQ limit. The solar cell parameters like open-circuit voltage (Voc), fill factor (FF), short circuit current density (Jsc), and solar cell efficiency are calculated as per previously reported results.5,41 More information is provided in the ESI† as shown in Tables 3, S1 and S2.†
Table 3 Represents calculated solar cell parameters for open circuit voltage, fill factor, short circuit current density, power conversion efficiency of MASnBr3 monolayer
|
Voc (eV) |
FF |
Jsc (mA cm−2) |
H (%) |
MASnBr3 |
2.64 |
0.94 |
9.40 |
23.46 |
For 2D MASnBr3 solar cell efficiency is 23.46%. Which is 18% larger than that of the previously reported lead-based 2D MAPbI3 (ref. 43) perovskites. Also, we have found that in comparison with experimental results. The power conversion efficiency of lead-free perovskites developed by Hao et al. and Xu et al. group have reached up to 5.73% and 8.79%, respectively.49,51 Overall in comparison with lead-free 3D hybrid halide perovskites with 2D hybrid halide perovskites. The 2D hybrid halide perovskites are more efficient and suitable candidates to develop new generation solar cells.5,41,43,49,51,57 Under both tensile and compressive strain conditions. The calculated solar cell efficiency varies from 13% to 23% as shown in Tables 3, S1 and S2.† Therefore we conclude that the lead-free 2D MASnBr3 is a suitable candidate for solar cell and photovoltaic applications.
4. Conclusions
Here, the 2D MASnBr3 and Ruddlesden–Popper perovskites BAMASn2Br7 hybrid halide perovskites have flexible structural and compositional properties. Also, the calculated bandgap and lattice parameters of MASnBr3 and Ruddlesden–Popper perovskites BAMASn2Br7 are consistent with previously reported experimental and theoretical results. Under mechanical strain, i.e., tensile and compressive strain tunable bandgap activity is consistent with previously reported, showing sustainability of the structure up 10% in MASnBr3 and up to 8% in BAMASn2Br7. We found highest carrier mobility for electron up to 404 cm2 V−1 s−1 and holes 800 cm2 V−1 s−1 for MASnBr3, and BAMASn2Br7 highest carrier mobility up to 557 cm2 V−1 s−1 for electron and up to 779 cm2 V−1 s−1 for the hole, which are 14% and 24% higher than the reported data. The calculated solar cell parameters open-circuit voltage (Voc), fill factor (FF), short circuit current density (Jsc), and solar cell efficiency are consistent with the previously reported experimental and theoretical results. Here, tin-based 2D MASnBr3 shows high efficiency up to 23.46%, 18% higher than the lead-based perovskites. In optical activity, a high static dielectric constant shows good device performance. With high absorption coefficient up to 15.25 × 105 cm−1 for MASnBr3 and BAMASn2Br7 absorption coefficient up to 13.38 × 105 cm−1. Therefore overall results of this theoretical study. With mechanical strain engineering may be convenient to the experimentalist support to improve the performance of the 2D perovskites. This study supports these materials as promising candidates for photovoltaic and solar cell device applications.
Conflicts of interest
There are no conflicts to declare.
Acknowledgements
S. R. K. and Y. A. S. are thankful to the India government (SERB), grant number: EEQ/2016/000217 for the financial support. We also thankful C-DAC Pune for providing us cluster computational facilities for work.
References
- C. Liu, W. Ding, X. Zhou, J. Gao, C. Cheng, X. Zhao and B. Xu, Efficient and Stable Perovskite Solar Cells Prepared in Ambient Air Based on Surface-Modified Perovskite Layer, J. Phys. Chem. C, 2017, 121, 6546–6553 CrossRef CAS.
- G. Grancini, C. Roldan-Carmona, I. Zimmermann, E. Masconi, X. Lee, D. Martineau, S. Narbey, F. Oswald, F. D. Angelis, M. Gratzel and M. K. Nazeeruddin, One-Year Stable Perovskite Solar Cells by 2D/3D Interface Engineering, Nat. Commun., 2017, 8, 15684 CrossRef CAS PubMed.
- G. Lozano, The Role of Metal Halide Perovskites in Next Generation Lighting Devices, J. Phys. Chem. Lett., 2018, 9, 3987–3997 CrossRef CAS PubMed.
- Z. Zhang, L. Ren, H. Yan, S. Guo, S. Wang, M. Wang and K. Jin, Bandgap Narrowing in Bi-Doped CHNPbCl Perovskite Single Crystals and Thin Films, J. Phys. Chem. C, 2017, 121, 17436–17441 CrossRef CAS.
- S. Kumavat, Y. Sonvane, D. Singh and S. Gupta, Two-Dimensional CH3NH3PbI3 with High Efficiency and Superior Carrier Mobility: A Theoretical Study, J. Phys. Chem. C, 2019, 123, 5231–5239 CrossRef CAS.
- H. Jung, C. Stompus, M. Kanatzidis and V. Dravid, Self-Passivation of 2D Ruddlesden–Popper Perovskite by Polytypic Surface PbI2 Encapsulation, Nano Lett., 2019, 19, 6109–6117 CrossRef CAS PubMed.
- C. Motta, F. El-Mellouhi and S. Sanvito, Charge Carrier Mobility in Hybrid Halide Perovskite, Sci. Rep., 2015, 5, 12746 CrossRef CAS PubMed.
- G. Giorgi, J.-I. Fujisawa, H. Segawa and K. Yamashita, Small Photocarrier Effective Masses Featuring Ambipolar Transport in Methylammonium Lead Iodide Perovskite: A Density Functional Analysis, J. Phys. Chem. Lett., 2013, 4, 4213–4216 CrossRef CAS.
- A. Krishna, S. Gottis, M. Nazeeruddin and F. Sauvage, Mixed Dimensional 2D/3D Hybrid Perovskite Absorbers: The Future of Perovskite Solar Cells?, Adv. Funct. Mater., 2018, 1806482 Search PubMed.
- A. Bala, A. Deb and V. Kumar, Atomic and electronic Structure of Two Dimensional Inorganic Halide perovskites An+1MnX3n+1 (n = 1–6, A = Cs, M = Pb and Sn, and X = Cl, Br, and I) from Ab Initio Calculations, J. Phys. Chem. C, 2018, 12, 7464–7473 CrossRef.
- D. O. Demchenko, N. Izyumskaya, M. Feneberg, V. Avrutin, U. Ozgur, R. Goldhahn and H. Morkoc, Optical Properties of the Organic-Inorganic Hybrid Perovskite CH3NH3PbI3: Theory and Experiment, Phys. Rev. B, 2016, 94, 075206 CrossRef.
- A. Agresti, S. Pescetelli, A. L. Palma, A. Castillo, D. Konios, G. Kakavelakis, S. Razza, L. Cina, E. Kymakis, F. Bonaccorso and A. Carlo, Graphene Interface Engineering for Perovskite Solar Modulus: 12.6% Power Conversion Efficiency over 50 cm2 active Area, ACS Energy Lett., 2017, 2, 279–287 CrossRef CAS.
- L. Zhang and W. Liang, How the Structures and Properties of Two-Dimensional Layered Perovskites MAPbI3 and CaPbI3 Vary with the Number Layers, J. Phys. Chem. Lett., 2017, 8, 1517–1523 CrossRef CAS PubMed.
- H. Tsai, W. Nie, J.-C. Blancon, C. C. Stoumpos and R. Asadpour, et al., High-Efficiency Two-Dimensional Ruddlesden-Popper Perovskite Solar Cells, Nature, 2016, 536, 312–316 CrossRef CAS PubMed.
- C. Stoumpos, D. Cao, D. Clark, J. Young, J. Rondinelli, J. Jang, J. Hupp and M. Kanatzidis, Ruddlesden-Popper Hybrid Lead Iodide Perovskite 2D homologus Semiconductors, Chem. Mater., 2016, 28, 2852–2867 CrossRef CAS.
- F. Corsini and G. Griffini, Recent progress in encapsulation strategies to enhance the stability of organometal halide perovskite solar cells, J. Phys. Energy, 2020, 2, 031002 CrossRef CAS.
- X. Gao, X. Zhang, W. Yin, H. Wang, Y. Hu, Q. Zhang, Z. Shi, V. Colvin, W. Yu and Y. Zhang, Ruddlesden-Popper Perovskites: Synthesis and Optical Properties for Optoelectronic Applications, Adv. Sci., 2019, 6, 1900941 CrossRef CAS.
- M. E. Kamming, H.-H. Fang, M. A. Loi, G. Brink, G. Black, T. Palstra and J. Elshof, Micropatterned 2D Hybride Perovskite Thin Films with Enhanced Photoluminescence Lifetimes, ACS Appl. Mater. Interfaces, 2018, 10, 12878–12885 CrossRef PubMed.
- R. K. Misra, B. Cohen, L. Iagher and L. Etgar, Low Dimensional Organic–Inorganic Halide Perovskite: Structure, Properties, and Applications, ChemSusChem, 2017, 10, 3712–3721 CrossRef CAS.
- J. Lu and Z. Wei, The Strategies for preparing blue perovskites light-emitting diodes, J. Semicond., 2020, 41, 051203 CrossRef CAS.
- X. Chin, D. Cortecchia, J. Yin, A. Bruno and C. Soci, Lead Iodide Perovskite Light-Emitting Filed Effect Transistor, Nat. Commun., 2015, 6, 7383 CrossRef CAS PubMed.
- Z. Wang, J. Liu, Z. Q. Xu, Y. Xue, L. Jiang, J. Song, F. Huang, Y. Wang, Y. L. Zhong, Y. Zhang, Y.-B. Cheng and Q. Bao, Wavelength-Tunable Waveguide Based on Polycrystalline Organic- Inorganic Perovskite Microwave, Nanoscale, 2016, 8, 6258–6264 RSC.
- E. J. Yoo, M. Lyu, J. H. Yun, C. J. Kang, Y. J. Choi and L. Wang, Resistive Switching Behavior in Organic-Inorganic Hybrid CH3NH3PbI3−xClx Perovskite for Resistive Random Access Memory Devices, Adv. Mater., 2015, 27, 6170–6175 CrossRef CAS PubMed.
- G. Xing, N. Mathews, S. S. Lim, N. Yantara, X. Liu, D. Sabba, M. Gratzel, S. Mhaisalkar and T. C. Sum, Low-Temperature Solution Processed Wavelength-Tunable Perovskite for Lasing, Nat. Mater., 2014, 13, 476–480 CrossRef CAS PubMed.
- Y. Fang, Q. Dong, Y. Shao, Y. Yuan and J. Huang, Highly Narrowband Perovskite Single-crystal Photodetectors Enable by Surface-Charge Recombination, Nat. Photonics, 2015, 9, 679–686 CrossRef CAS.
- G. Niu, W. Li, F. Meng, L. Wang, H. Dong and Y. Qiu, Study on the stability of CH3NH3PbI3 films and the effect of post-modification by aluminum oxide in all-solid-state hybrid solar cells, J. Mater. Chem. A, 2014, 2, 705–710 RSC.
- I. C. Smith, E. Hoke, D. Solis-lbarra, M. McGehee and H. Karunadasa, A Layered Hybrid Perovskite Solar Cell Absorber with Enhanced Moisture Stability, Angew. Chem., Int. Ed., 2014, 53, 11232–11235 CrossRef CAS.
- Y. Zhou and Y. Zhao, Chemical stability and instability of inorganic halide perovskites, Energy Environ. Sci., 2019, 12, 1495–1511 RSC.
- Z. Wang, Z. Shi, T. Li, Y. Chen and W. Huang, Stability of Perovskites Solar Cells: A Prospective on the Substitution of the A Cation and X Anion, Angew. Chem., Int. Ed., 2017, 56, 1190–1212 CrossRef CAS PubMed.
- D. Liang, Y. Peng, Y. Fu, M. J. Shearer, J. Zhang, J. Zhai, Y. Zhang, R. J. Hamers, T. L. Andrew and S. Jin, Colorpure violet-light-emitting diodes based on layered lead halide perovskite nanoplates, ACS Nano, 2016, 10, 6897–6904 CrossRef CAS PubMed.
- F. Chiarella, A. Zappettini, F. Licci, I. Borriello, G. Cantele, D. Ninno, A. Cassinese and R. Vaglio, Combined experimental and theoretical investigation
of optical, structural, and electronic properties of CH3NH3SnX3 thin films (X = Cl, Br), Phys. Rev. B: Condens. Matter Mater. Phys., 2008, 77, 045129 CrossRef.
- K. Yamada, K. Nakada, Y. Takeuchi, K. Nawa and Y. Yamane, Tunable Perovskite Semiconductor CH3NH3SnX3 (X = Cl, Br, or I) Characterized by X-ray and DTA, Bull. Chem. Soc. Jpn., 2011, 84, 926–932 CrossRef CAS.
- F. Funabiki, Y. Toda and H. Hosono, Optical and Electrical Properties of Perovskites Variant (CH3NH3)2SnI6, J. Phys. Chem. C, 2018, 122, 10749–10754 CrossRef CAS.
- K. Zheng and T. Pullerits, Two Dimensions Are Better for Perovskites, J. Phys. Chem. Lett., 2019, 10, 5881–5885 CrossRef CAS PubMed.
- C. Stoumpos, C. Soe, H. Tsai, W. Nie, J. Blancon, D. Cao, F. Liu, B. Traore, C. Katan, J. Even, A. Mohite and M. Kanatzidis, High Members of the 2D Ruddlesden-Popper Halide Perovskites: Synthesis, Optical Properties, and Solar Cells of (CH3(CH2)3NH3)2(CH3NH3)4Pb5I16, Chem, 2017, 2, 427–440 CAS.
- C. Underwood, J. Carey and S. Silva, Nonlinear Band Gap Dependence of Mixed Pb-Sn 2D Ruddlesden-Popper PEA2Pb1−XSnXI4 Perovskites, J. Phys. Chem. Lett., 2021, 12, 1501–1506 CrossRef CAS.
- Y. Hua, Y. Zhou, D. Hong, S. Wan, X. Hu, D. Xie and Y. Tian, Identification of the Band Gap Energy of Two-dimensional (OA)2(MA)n−1PbnI3n+1 Perovskite with up to 10 Layers, J. Phys. Chem. Lett., 2019, 10, 7025–7030 CrossRef CAS PubMed.
- R. Dong, C. Lan, X. Xu, X. Liang, X. Hu, D. Li, Z. Zhou, L. Shu, S. Yip and C. Li, et al., Novel Series of Quasi-2D Ruddlesden- Popper Perovskites Based on Short-Chained Spacer Cation for Enhanced Photodetection, ACS Appl. Mater. Interfaces, 2018, 10, 19019–19026 CrossRef CAS.
- X. Tian, Y. Zhang, R. Zheng, D. Wei and J. Liu, Two-dimensional Organic–inorganic Hybrid Ruddlesden–Popper Perovskite Materials: Preparation, Enhanced Stability, and Applications in Photodetection, Sustainable Energy Fuels, 2020, 4, 2087–2113 RSC.
- A. Z. Chen, M. Shiu, J. H. Ma, M. R. Alpert, D. Zhang, B. J. Foley, D.-M. Smilgies, S.-H. Lee and J. J. Choi, Origin of Vertical Orientation in Two-Dimensional Metal Halide Perovskite and Its Effect on Photovoltaic Performance, Nat. Commun., 2018, 9, 1336 CrossRef PubMed.
- S. Kumavat, Y. Sonvane and S. Gupta, Structural, optical, transport, and solar cell properties of 2D halide perovskite MAZX3 (Z = Pb, Sn, and X = Cl, Br, I), J. Appl. Phys., 2020, 128, 114304 CrossRef CAS.
- H. Zheng, G. Liu, L. Zhu, J. Ye, X. Zhang, A. Alsaedi, T. Hayat, X. Pan and S. Dai, The Effect of Hydrophobicity of Ammonium Salts on Stability of Quasi-2D Perovskite Materials in Moist Condition, Adv. Energy Mater., 2018, 8, 1800051 CrossRef.
- H. Kagdada, S. Gupta, S. Sahoo and D. Singh, Rashba Splitting in Two Dimensional Hybrid Perovskite Materials for High Efficient Solar and Heat Energy Harvesting, J. Phys. Chem. Lett., 2020, 11, 7679–7686 CrossRef CAS PubMed.
- J.-H. Yang, Q. Yuan and I. Yakobson, Chemical Trends of Electronic Properties of Two-Dimensional Halide Perovskite and Their Potential Application for Electronic and Optoelectronics, J. Phys. Chem. C, 2016, 120, 24682–24687 CrossRef CAS.
- J.-H. Yang, Q. Yuan and I. Yakobson, Chemical Trends of Electronic Properties of Two-Dimensional Halide Perovskite and Their Potential Application for Electronic and Optoelectronics, J. Phys. Chem. C, 2016, 120, 24682–24687 CrossRef CAS.
- W. E. I. Sha, X. Ren, L. Chen and W. C. H. Choy, The Efficiency Limit of CH3NH3PbI3 Perovskite Solar Cells, Appl. Phys. Lett., 2015, 106, 221104 CrossRef.
- C. Lopez, C. Abia, J. Gainza, P. Kayser, N. Nemes, O. Dura, J. Martinez, M. Fernandez-Diaz, C. Alvarez-Galvan and J. Alonso, Structural evolution, optical gap and thermoelectric properties of CH3NH3SnBr3 hybrid perovskite, prepared by mechanochemistry, Mater. Adv., 2021, 2, 3620 RSC.
- R. Chakraborty and A. Nag, Correlation of Dielectric Confinement and Excitonic Binding Energy in 2D Layered Hybrid Perovskites Using Temperature Dependent Photoluminescence, J. Phys. Chem. C, 2020, 124, 16177–16185 CrossRef CAS.
- F. Hao, C. C. Stoumpos, D. H. Cao, R. P. H. Chang and M. G. Kanatzidis, Lead free solid-state organic-inorganic halide perovskite solar cells, Nat. Photonics, 2014, 8, 489–494 CrossRef CAS.
- Q. Sun and W. Yin, Thermodynamic Stability Trend of Cubic Perovskites, J. Am. Chem. Soc., 2017, 139, 14905–14908 CrossRef CAS PubMed.
- H. Xu, H. Yuan, J. Duan, Y. Zhao, Z. Jiao and Q. Tang, Lead-free CH3NH3SnBr3-xIx perovskite quantum dots for mesoscopic solar cell applications, Electrochim. Acta, 2018, 282, 807–812 CrossRef CAS.
- A. Shukla, V. Sharma, S. Gupta and A. Verma, Computational determination of the physical thermoelectric parameter of tin based organometallic halide perovskites (CH3NH3SnX3 X= Br and I): Emerging materials for optoelectronic devices, Mater. Chem. Phys., 2020, 253, 123389 CrossRef CAS.
- N. Zibouche and M. S. Islam, Structure–Electronic Property Relationships of 2D Ruddlesden–Popper Tin- and Lead-based Iodide Perovskites, ACS Appl. Mater. Interfaces, 2020, 12, 15328–15337 CrossRef CAS PubMed.
- K. Korshunova, L. Winterfeld, W. Beenken and E. Runge, Thermodynamic stability of mixed Pb:Sn methyl-ammonium halide perovskites, Phys. Status Solidi B, 2016, 253, 1907–1915 CrossRef CAS.
- K. Amnuyswat and P. Thanomngam, Roles of spin-orbit coupling in tetragonal hybrid halide perovskite for photovoltaics light-absorber, Mater. Today: Proc., 2018, 5, 14857–14861 CAS.
- Z. Wang, F. Wang, B. Zhao, S. Qu, T. Hayat, A. Alsaedi, L. Sui, K. Yuan, J. Zhang, Z. Wei and Z. Tan, Efficient Two-Dimensional Tin Halide Perovskite Light-Emitting Diodes via a Spacer Cation Substitution Strategy, J. Phys. Chem. Lett., 2020, 11, 1120–1127 CrossRef CAS.
- A. Iefanova, N. Adhikari, A. Dubey, D. Khatiwada and Q. Qiaoa, Lead free CH3NH3SnI3 perovskite thin-film with p-type semiconducting nature and metal-like conductivity, AIP Adv., 2016, 6, 085312 CrossRef.
- E. McClure, A. McCormick and P. Woodward, Four Lead-free Layered Double Perovskites with the n =1 Ruddlesden–Popper Structure, ACS Inorg. Chem., 2020, 59, 6010–6017 CrossRef CAS PubMed.
- L. Ma, M. Ju, J. Daia and X. Zeng, Tin and Germanium Based Two-dimensional Ruddlesden–Popper Hybrid Perovskites for Potential Lead-Free Photovoltaic and Photoelectronic Applications, Nanoscale, 2018, 10, 11314–11319 RSC.
- S. Ghosh, D. D. Sante and A. Stroppa, Strain Tuning of Ferroelectric Polarization in Hybrid Organic Inorganic Perovskite Compounds, J. Phys. Chem. Lett., 2015, 6, 4553–4559 CrossRef CAS.
- M. D. Ventra and S. T. Pantelides, Hellmann-Feynman Theorem and the Definition of Force in Quantum Time-Dependent and Transport Problems, Phys. Rev. B: Condens. Matter Mater. Phys., 2000, 61, 16207 CrossRef.
- M. Baranowski, S. J. Zelewski, M. Kepenekian, B. Traoré, J. M. Urban, A. Surrente, K. Galkowski, D. K. Maude, A. Kuc, E. Booker, R. Kudrawiec, S. D. Stranks and P. Plochocka, Phase-Transition-Induced Carrier Mass Enhancement in 2D Ruddlesden–Popper Perovskites, ACS Energy Lett., 2019, 4, 2386–2392 CrossRef CAS.
- P. Blöchl, Projected Augmented-Wave Method, Phys. Rev. B: Condens. Matter Mater. Phys., 1994, 50, 17953 CrossRef.
- G. Kresse and J. Furthermullaer, Efficient Iterative Schemes for ab initio Total Energy Calculations Using a Plane-Wave Basis Set, Phys. Rev. B: Condens. Matter Mater. Phys., 1996, 54, 11169 CrossRef CAS.
- G. Kresse and D. Joubert, From Ultrasoft Pseudopotentials to the Projected Augmented-Wave Method, Phys. Rev. B: Condens. Matter Mater. Phys., 1999, 59, 1758 CrossRef CAS.
- J. Perdew, K. Burke and M. Ernzerhof, Generalized Gradient Approximation Made Simple, Phys. Rev. Lett., 1997, 78, 1396 CrossRef CAS.
- J. Heyd, G. E. Scuseria and M. Ernzerhof, Hybrid Functionals Based on Screened Coulomb Potential, J. Chem. Phys., 2003, 118, 8207–8215 CrossRef CAS.
- Y. Yamada, T. N. M. Endo, A. Wakamiya and Y. Kanemitsu, et al., Photo Carrier Recombination Dynamics in Perovskite CH3NH3PbI3 for Solar Cell Applications, J. Am. Chem. Soc., 2014, 136, 11610–11613 CrossRef CAS PubMed.
- W.-J. Yin, J.-H. Yang, J. Kang, Y. Yan and S.-H. Wei, Halide Perovskite Materials for Solar Cells: a Theoretical Review, J. Mater. Chem. A, 2015, 3, 8926–8942 RSC.
- B. W. Veal and A. P. Paulikas, Optical Properties of Molybdenum. I. Experiment and Kramers-Kronig Analysis, Phys. Rev. B: Solid State, 1974, 10, 1280 CrossRef CAS.
- M. Dressel and M. Scheffler, Verifying the Drude Response, Ann. Phys., 2006, 15, 535–544 CrossRef CAS.
|
This journal is © The Royal Society of Chemistry 2023 |
Click here to see how this site uses Cookies. View our privacy policy here.