DOI:
10.1039/D3RA01514A
(Paper)
RSC Adv., 2023,
13, 12114-12122
Nitrogen-doped carbon dots enhanced seedling growth and salt tolerance with distinct requirements of excitation light†
Received
7th March 2023
, Accepted 10th April 2023
First published on 18th April 2023
Abstract
Numerous nanomaterials with optical properties have demonstrated excellent capacities to enhance plant growth and stress tolerance. However, the corresponding mechanisms have only been partially characterized, especially the excitation-light dependencies of different actions. Here, nitrogen-doped carbon dots (N-CDs) were developed to explore the excitation-light dependence in N-CD-induced growth enhancement and salt tolerance. Compared to the control, N-CDs induced significant enhancements in Arabidopsis thaliana growth under excitation light, including fresh/dry weight of shoot (21.07% and 16.87%), chlorophyll content (9.17%), soluble sugar content (23.41%), leaf area (28.68%), total root length (34.07%) and root tip number (46.69%). In the absence of excitation light, N-CD-treated seedlings exhibited little differences in these parameters, except the enhancements in root length (24.51%) and root tip number (10.24%). On the other hand, N-CD-treatment could improve seedling salt tolerance with or without excitation light. Under salt stress (150 mM NaCl), in the presence of excitation light, the N-CDs treatment significantly increased shoot/root fresh weight and chlorophyll content by 43.29%, 50.66% and 22.59%, and reduced malondialdehyde (MDA) content and relative conductivity by 17.59% and 32.58% compared to the control group. In the absence of excitation light, significant enhancements in shoot/root fresh weight (34.22%, 32.60%) and chlorophyll content (10.45%), and obvious decreases in MDA content (28.84%) and relative conductivity (16.13%) were also found. These results indicated that N-CDs only induced growth enhancement under excitation light, but they improved salt tolerance with and without excitation light, suggesting that the two effects occurred via distinct signaling pathways. This study revealed the excitation-light dependencies of nanomaterial-involved agriculture applications, providing insight into designing more efficient nanomaterials in the future.
Introduction
As the cornerstone of modern society, agriculture and the food security it provides are critical to sustainable human development.1,2 Although the green revolution has enabled crops to increase yields year-over-year, the increasing demand on agricultural production due to the growing population is flattening enhancement gains.3,4 Furthermore, soil degradation and climate change have exerted severe stresses on modern crops, threatening global food safety.5 It was found that approximately 50% of crop yield losses were caused by biotic/abiotic stresses, such as increased soil salinity, water deficiency, crop pests, land desertification, etc.6–8 To address these problems, several strategies have allowed modest increases in global crop yields, primarily through improving the cultivation environment,9–11 innovations in agrochemicals,12,13 and the exploitation of genetic engineering techniques.14–17 Despite the great achievements in growth enhancement and stress resistance achieved through these strategies, several questions regarding inefficiency, high pollution, and social controversies remain. Therefore, efficient strategies to enhance both growth and stress tolerance are still research hotspots in the agriculture field.
Recently, researches in agriculture nanotechnology, referred to as “plant nanoscience”, have produced promising results in recent years, demonstrating its potential as a tool for improving plant growth and stress tolerance.18,19 Nanomaterials (<100 nm in size) have excellent optical properties, environmental friendliness, high biocompatibility, and high cost performance efficiency, and they can easily enter plant cells where they regulate plant physiological activities.20 The effects of nanomaterials on plant growth and stress tolerance have been widely reported.19 Among the examples, single-walled carbon nanotubes (SWNTs) have been shown to localize within chloroplasts and promote photosynthetic activity.21 It was also reported that silica nanoparticles (NPs) not only improved seedling photosynthesis in wheat and lupin, but also protected rice against abiotic/biotic stresses.22,23 Similarly, Wu et al. demonstrated that cerium oxide (CeO2) NPs with a low Ce3+/Ce4+ ratio protected seedling photosynthesis by scavenging reactive oxygen species (ROS) induced by abiotic stresses.24 Rossi et al. showed that CeO2 NPs altered Na+ fluxes and reduced Na+ accumulation in roots under salt stress, resulting in a better performance of Brassica.25 Collectively, numerous nanomaterials have shown to have the capacity to promote seedling growth and stress tolerance.
As a promising carbon-based nanomaterial family, carbon dots (CDs) have shown excellent biocompatibility and optical performance, and in the last decade have been shown to have a wide variety of applications in the nanoagriculture field,20 exhibiting beneficial effects on seedling germination,26–28 photosynthesis enhancement,29–40 and stress resistance.41–50 For instance, degradable CDs,39 far-red CDs,51 element-doped CDs,32,52–54 glucose-functionalized CDs,55 and spirulina-based CDs56 have all demonstrated outstanding abilities to enhance photosynthesis and plant growth. Several mechanisms of how CDs affect photosynthesis have been proposed. Electron/energy transfer from CDs to chloroplasts40,57,58 and light conversion capacity59,60 have been suggested to contribute to these effects, although no conclusive evidence has been obtained. Furthermore, remarkable progress has been made in using CDs for stress tolerance enhancement, such as carbon dots (CDs) with different oxygen contents,30 salvia miltiorrhiza-derived CDs48,49 and nitrogen/sulfur co-doped CDs.61 In enhancing stress tolerance, CDs have been shown to reduce stress-induced oxidative damage, probably due to their ROS scavenging capacities49,62 and ROS-independent Ca2+ mobilization.48
Despite these great achievements, the underlying mechanisms have not been fully characterized, especially the excitation-light dependencies. As optical excitation nanomaterials, the roles of excitation light in these two CD-induced effects (improved growth and stress tolerance) have not been systemically explored. Recently, xenon lamps mimicking natural sunlight were employed,37,51,63,64 and in other cases no information on cultivation light was provided,32,35,43,54,60 leading to authors neglecting the effect of excitation light dependence. Therefore, this work explored the mechanisms underlying the effects of nitrogen-doped carbon dots (N-CDs) in enhancing seedling growth and salt tolerance. Specifically, the excitation light requirements of the two actions were shown to differ, indicating that N-CDs promoting plant growth and stress resistance had distinct mechanisms of action. Taken together, this work provides new insight into the application potential of CDs in agriculture.
Experimental
N-CDs synthesis
N-CDs were synthesized via hydrothermal treatment of diethylenetriamine and citric acid.65 In detail, 0.5 g citric acid and 280 μL diethylenetriamine were dissolved in 10 mL distilled water in a 100 mL Teflon-lined stainless steel autoclave. The mixture was heated at 180 °C for 4 h to obtain crude N-CD solutions. The N-CDs were further purified by filtration, freeze-drying, and acetone precipitation to finally obtain yellow-brown powders. To determine the effect of nitrogen doping on the optical band gap of N-CDs, undoped CDs were also prepared through the similar procedure, in which no diethylenetriamine was used.
N-CDs characterization
The UV-vis absorption spectra were measured using a Shimadzu UV3600 UV-vis absorption spectrophotometer and the corresponding optical band gaps (Eg) of CDs were estimated through the Tauc plot [the curve of (αhν)2 versus hν converted from the UV-vis spectrum].66 The photoluminescence spectra were characterized using a fluorescence spectrophotometer (LUMINA, Thermo Scientific). The morphology of the N-CDs was observed by transmission electron microscope (TEM, FEI Tecnai G2 F20) and atomic force microscope (AFM, Bruker Dimension Icon), and the particle size was calculated using the Nano Measurer software. The XRD spectra were measured by Rigaku SmartLab SE (2θ = 10°–90°). The structure and composition of the N-CDs were examined by X-ray photoelectron spectrometer (XPS, Thermo Scientific ESCALAB 250Xi) and Fourier transform infrared spectrophotometer (FT-IR). The zeta potentials of the N-CDs were determined by nanoparticle size analyzer (Zetasizer nano ZS90, Malvern) under different pH conditions.
Plant materials and growth conditions
Arabidopsis thaliana. The Arabidopsis (wild-type Col-0) seeds were sterilized with 75% ethanol and 2% sodium hypochlorite and sown in 1/2 MS medium.10 After vernalization (4 °C, darkness) for 72 h, they were transferred into a light incubator (22 °C, light/dark 16 h/8 h). After 3 days, seedlings with uniform growth were selected and used in different experiments. (1) Some seedlings were transplanted into soil environments (100% vermiculite) and acclimatized in the greenhouse for 10 days under normal light illumination (20.4267 μmol m−2 s−1). After acclimation, the seedlings were irrigated with or without N-CDs (300 mg L−1) and with or without excitation light (λmax = 365 nm, 0.8325 μmol m−2 s−1), respectively. The seedlings were photographed and phenotypes were recorded at 20 days, at which point the bolting stems were cut to allow better observation of the growth pattern of the rosette leaves. The fresh and dry weights, chlorophyll contents, soluble sugar contents, root and leaf parameters, and other related indices were measured. (2) Seedlings were transplanted into soil and acclimatized for 10 days under normal light illumination. Then the seedlings were irrigated with 150 mM NaCl solution with or without N-CDs (300 mg L−1) and with or without excitation light (λmax = 365 nm, 0.8325 μmol m−2 s−1). After 14 days of cultivation the relevant indices were measured, including fresh weight, chlorophyll content, MDA content, relative conductivity, and Fv/Fm value. (3) Some seedlings were transferred directly to 1/2 MS medium containing 0/50/100/150 mM NaCl with or without the addition of N-CDs (300 mg L−1) and with or without excitation light (λmax = 365 nm, 0.8325 μmol m−2 s−1), respectively. After 14 days of cultivation, the fresh weight, chlorophyll content, and other indices were measured.
Uptake and distribution of N-CDs in Arabidopsis
After 20 days of culturing in N-CD solutions (300 mg L−1), images of the control and N-CDs treated groups were taken in dark room under excitation light (λmax = 365 nm, 0.8325 μmol m−2 s−1) irradiation to observe the distribution of N-CDs in the whole Arabidopsis plant. Further, the fluorescence of different tissues such as roots, leaves, and fruit pods in Arabidopsis seedlings was observed by high-resolution confocal laser microscopy (LSM 880) to confirm the distribution of N-CDs in plants (408 nm excitation, 430–500 nm collection).
Measurement of plant physiology and growth
Plant weight. Several plant seedlings were randomly selected from each treatment, then weighed for fresh weight. Subsequently, they were killed at 105 °C for 30 minutes and dried at 80 °C to a constant weight to obtain the dry weight.
Stem length. Images of entire plants were taken against a black background and then the stem lengths were measured using the ImageJ (v1.52p) software.
Root system shape parameters. Pictures of root scans were analyzed using the WinPHIZO Pro 2019 software to obtain the total root length and root tips.
Leaf parameters. The leaf area and leaf number of plant leaves were measured using the ImageJ (v1.52p) software.
Elemental content. The elemental content was measured based on previous experimental methods with appropriate modifications.67 Completely dried plant materials from different treatments were ground into powder. 0.5 g of each sample was placed into a 50 mL conical flask and 5 mL concentrated sulfuric acid was added, then the mixture was shaken well and left overnight. The samples were digested by heating to 340 °C on an electric hot plate. When a few bubbles appeared in the liquid and white haze appeared in the triangular flask, 30% hydrogen peroxide was added dropwise until the solution became clear. The boiling product was diluted to 50 mL to obtain the final elemental solution which would be measured. After filtering through a 0.22 μm membrane, the elemental content was determined by ICP-AES.
Soluble sugar content. The soluble sugar content was determined using the phenol method.68 Samples were extracted in boiling water for 30 min. After cooling, the water with sample extract was centrifuged at 12
000 rpm for 10 min and the supernatant was placed into a new 15 mL centrifuge tube. The process was repeated twice to obtain the extract solution. Subsequently, 0.5 mL the extract solution, 1.5 mL distilled water, 1 mL 0.09 g mL−1 phenol solution, and 5 mL concentrated sulfuric acid were added in turn. After 30 min, the OD485 value was determined using UV-vis (Shimadzu UV3600), calculated as follows: soluble sugar content (mg g−1) = (108.49027 × OD485 − 18.99511)/[sample weight (g) × volume of extract solution for determination (mL)/volume of extract solution (mL)].
Chlorophyll content. The chlorophyll content was determined by the method described by Arnon.69 Leaves were placed in 95% ethanol solution and extracted in the dark until they had become completely discolored. After extraction, the absorption rates at 665 nm and 649 nm were measured with 95% ethanol as the control. Chlorophyll content was calculated as follows: chlorophyll a content (mg g−1) = (13.95 × OD665 − 6.88 × OD649) (mg L−1) × volume of extraction solution (mL) × dilution times/sample weight (g), chlorophyll b content (mg g−1) = (24.96 × OD649 − 7.32 × OD665) (mg L−1) × volume of extraction solution (mL) × dilution times/sample weight (g), chlorophyll content (mg g−1) = chlorophyll a content (mg g−1) + chlorophyll b content (mg g−1).
Malondialdehyde (MDA) content. The MDA content was determined using the thiobarbituric acid method.70 0.5 g sample was placed into 2 mL phosphate buffer, then ground until homogenous before adding 5 mL 0.5% TBA solution and boiling for 10 min. Then, the mixture was centrifuged at 12
000 rpm for 15 min and the supernatant was aspirated after cooling. Finally, the absorption rates at 450 nm and 532 nm were measured, to determine the OD450 and OD532 values. MDA content was calculated as follows: MDA (mmol g−1 FW) = (6.452 × OD532 − 0.559 × OD450) × volume of extraction solution (mL)/(volume of extraction solution for determination (mL) × sample weight (g)).
Relative conductivity. The samples were soaked in distilled water for 2 h at 25 °C and the initial conductivity of the solution (R1) was determined. Afterwards, the mixture was heated for 30 min at 100 °C and the conductivity of the solution (R2) was determined after cooling. Calculation formula: relative conductivity (%) = R1/R2 × 100%.71
Fv/Fm. After an adequate dark adaptation period, the FluorCam Enclosed Chlorophyll Fluorescence Imaging System was used to measure the Fv/Fm value.
Statistical analysis
All treatments had at least three biological replicates and all measurements had at least three technical replicates. Values were expressed as means ± standard deviation (SD). One-way ANOVA was used for the statistical analysis and groups were compared with the Tukey or LSD test in DPS7.05. Different letters were used to represent significant differences (P < 0.05). The F-test ANOVA of all data was in Tables S3–S9.†
Results and discussions
Characterization of N-doped CDs
N-CDs with UV-A light excitation and blue fluorescence emission were synthesized via the hydrothermal treatment of diethylenetriamine and citric acid at 180 °C for 4 h. As depicted in Fig. 1A, with 365 nm light irradiation, the N-CD solutions emitted blue fluorescence at 432 nm. Additionally, a strong absorption peak was observed at 350 nm, which was ascribed to the n–π* electronic transitions of the C
O bond.52,54 After synthesis, the N-CDs were morphologically characterized using TEM and AFM. As shown in Fig. 1B, C and S2A,† N-CDs exhibited spherical morphologies with an average particle diameter of 2.03 nm (TEM result) and height of 3.88 nm (AFM result). These results established the intrinsic nano-size features of N-CDs, which enable them to enter plant cells. The differences between the TEM and AFM results were likely due to the distinct measurement principles on which the two tests are based.72,73 The XRD profile of N-CDs exhibited a broad diffraction peak around 2θ of 25.0° (Fig. 1E), suggesting disordered carbon species. Subsequently, the chemical structure of the N-CDs was assessed using the FTIR and XPS spectra. Fig. 1D showed the FTIR spectrum of N-CDs. The peak at 1556 cm−1 belonged to the stretching vibration of C
C/C
N bonds. The strong vibration peak of carboxylate ions was observed around 1658 cm−1. The XPS analysis (Fig. S2B–E†) indicated that N-CDs were composed of C, N, and O elements with atomic ratios of 50.66%, 15.68%, and 27.68%, respectively. The high-resolution C 1s XPS spectrum illustrated that the peaks at 284.8, 286.4, 288.0, and 288.9 eV were attributed to the C–C/C
C, C–O/C–N, C
O/C
N, and O–C
O moieties, respectively. The O–C
O peak at 288.9 eV indicated the presence of carboxyl moieties on the surfaces of N-CDs. The ξ-potential characterization demonstrated that N-CDs presented negatively charged surfaces at pH 5.7–7.3 (Fig. 1F), suggesting there were carboxylic acid moieties on the N-CDs surfaces, which was in agreement with the XPS results. Furthermore, the corresponding optical band gap (Eg) of N-CDs was estimated from the Tauc plot. Fig. S3† demonstrated a direct band gap (2.73 eV) of N-CDs, which was higher than that of undoped CDs (2.53 eV). The increase of Eg value induced by N-doping, decreases the recombination rate of electron–hole pairs, and probably facilitates the charge delocalization and electron transfer,66,74,75 which is probably favourable for N-CD-involved plant phenotype.53
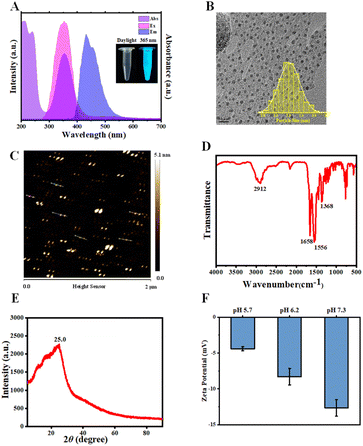 |
| Fig. 1 Chemical characterization of N-CDs. (A) UV-vis absorption and excitation and emission spectra of N-CDs. (B) Transmission electron microscopy (TEM) images of N-CDs and the size distribution of N-CDs (inserted image). (C) AFM image of N-CDs. (D) Fourier transform infrared (FTIR) spectra of N-CDs. (E) X-Ray diffraction (XRD) of N-CDs. (F) Zeta potential of N-CDs under pH 5.7/6.2/7.3 conditions. Error bars denote standard deviation. | |
Absorption and distribution of N-CDs
The uptake and transport of N-CDs in plants are curial for the N-CD-involved regulation of plant growth and development. The fluorescence properties of N-CDs can be employed to track their transport and distribution inside seedlings. After root irrigation with N-CDs (300 mg L−1) for 2 weeks, entire seedlings were rendered obviously blue due to the fluorescence of N-CDs, while the control seedlings exhibited red color, ascribed to the chloroplast autofluorescence (Fig. 2). Confocal microscopy (Fig. 2) showed that N-CDs were successively present in roots, leaves, and fruit pods. This indicated that the N-CDs could be absorbed by roots and transported to the above-ground tissues, such as leaves and fruit pods, a process likely driven primarily by transpiration. Furthermore, N-CDs could be observed in cells (Fig. 2). These results suggested that N-CDs can enter into cells, endowing themselves with the potential to regulate plant physiological activities, such as growth and stress resistance.
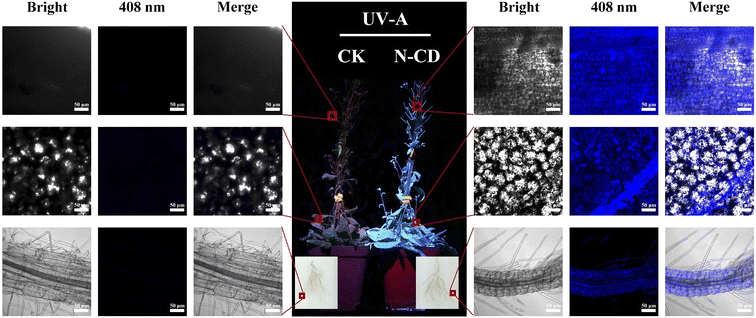 |
| Fig. 2 Absorption and distribution of N-CDs in Arabidopsis. (Middle) Picture captured under excitation light irradiation (365 nm). (Left and right) Confocal microscopy images of root, leaf, and fruit pods in seedlings treated with or without 300 mg L−1 N-CDs (408 nm excitation, 430–500 nm collection). | |
N-CDs enhanced seedling growth via excitation light dependence
After examining absorption, the effects of N-CDs on seedling growth were explored using Arabidopsis seedlings with a focus on the excitation light dependence. After root irrigation with N-CDs (300 mg L−1) for 20 days in the presence or absence of excitation light (365 nm), the growth phenotypes and associated indicators were measured (Fig. 3). Interestingly, under excitation light-free conditions, the N-CD-treated seedlings exhibited little difference in growth phenotype, fresh/dry weight of shoot, chlorophyll content, soluble sugar, and leaf area/number compared to the control group (Fig. 3). However, the underground tissues under N-CDs treatment increased (Fig. S4†), with increased total root length (24.51%) and root tip number (10.24%), thereby leading to increased fresh and dry weights of roots (19.86% and 12.70%, respectively). This phenomenon was likely due to the effects of CDs on endogenous auxin levels.76,77 Collectively, the N-CD-induced growth enhancement was relatively low and not significant in the absence of excitation light.
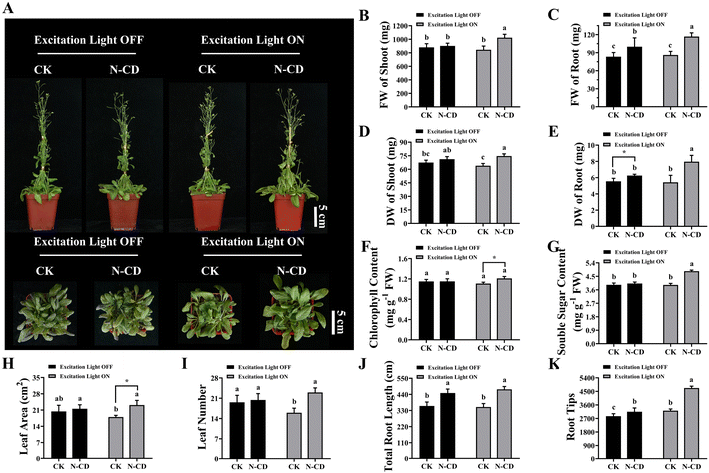 |
| Fig. 3 Effects of N-CDs on growth in the presence or absence of excitation light. (A) Effects of N-CDs (300 mg L−1) on the mature Arabidopsis seedlings on growth under light illumination with or without excitation light. Pictures captured after 20 days of cultivation. In the lower images in (A), the bolting stems of seedlings were cut to observe the seedling growth smoothly. (B–K) Fresh weights of shoots (B), fresh weights of roots (C), dry weights of shoots (D), dry weights of roots (E), chlorophyll contents (F), soluble sugar contents (G), leaf areas (H), leaf numbers (I), total root lengths (J), and root tips (K) in all treatments. All data (n ≥ 3) were analyzed using one-way analysis of variance (ANOVA), where different letters represent significant differences (P < 0.05, Tukey test). | |
Meanwhile, several seedlings were treated with N-CD solutions (300 mg L−1) root irrigation in the presence of excitation light for 20 days (Fig. 3 and S4†). As expected, N-CD-treated seedlings showed significant increases in growth phenotypes, fresh and dry weights of shoots (21.07% and 16.87%, respectively), fresh and dry weights of roots (35.64% and 46.99%, respectively), chlorophyll content (9.17%), soluble sugar (23.41%), leaf area (28.68%), leaf number (43.75%), total root length (34.07%), and root tip numbers (46.69%) (Fig. 3B–I). What's more, as shown in Fig. S5,† the N-CD-treated seedling growth rate increased much more quickly in the presence of excitation light than those in the absence of excitation light. Notably, N-CD-treated seedlings presented outstanding increases in total root length (34.07%) and root tip number (46.69%) under excitation light irradiation, which were much greater than under excitation light-free conditions (Fig. 3J, K and S4†). To further confirm the influence of N-CDs on plant growth, seedlings of the woody plant M. hupehensis (Rosaceae family) were also treated with the N-CD solutions in the presence of excitation light. As in Arabidopsis, M. hupehensis exhibited significant growth enhancements in fresh weight, chlorophyll content, soluble sugar, stem length, leaf area, root length, and root surface area (Fig. S6†). Moreover, N-CDs also effectively promoted the absorption of nutrients, including the macro elements P, K, and Mg and the micro elements Fe, Zn, Cu, Mn, Mo, and B, which probably contributed to the enhanced seedling growth (Fig. S7†).
Based on these results, it was found that the N-CDs exerted a more pronounced enhancement effect on seedling growth in the presence of excitation light. As a proposed mechanism by which nanomaterials enhance photosynthesis, their optical properties are probably the main contributors to growth enhancement, facilitating electron/energy transfer40,57,58 and enhancing light conversion capacity.59,60 As shown in Fig. S8,† in these proposed mechanisms, when N-CDs are irradiated by excitation light, photogenerated electrons and holes are produced. The electrons can be transferred to chloroplasts where they enhance photosynthesis. The recombination irradiation of photogenerated electrons and holes results in fluorescence, converting excitation light to blue/red wavelengths that the photosynthesis system can employ. Therefore, N-CD-induced growth enhancement is excitation light-dependent to some extent. Furthermore, it has been reported that CDs can affect root development by influencing root apical auxin levels in illumination incubators,76,77 although the illumination light corresponding to these observations was not clearly characterized. This phenotypic effect was also observed in our work, with enhanced total root length and increased root tip number under excitation light.
N-CDs induced salt tolerance without excitation light dependence
Having demonstrated the N-CD-induced growth enhancement, we subsequently explored the role of N-CDs on salt tolerance, focusing on the effect of excitation light. With the growing threat of climate change, plants are exposed to various adverse stresses at greater frequencies.5 In this work, seedlings were cultivated in 1/2 MS medium containing 0/50/100/150 mM NaCl in the presence and absence of excitation light (Fig. S9 and S10†). Inspiringly, in the presence of excitation light, N-CD-treated seedlings presented greater growth performance (Fig. S9†) in all NaCl-containing groups compared to the control. These N-CD-treated seedlings also exhibited greater fresh weights (16.25%, 27.06%, and 49.86%, respectively), chlorophyll content (7.82%, 11.75%, and 39.75%, respectively), and lower relative conductivity (12.35%, 11.79%, and 15.02%, respectively) and malondialdehyde (MDA) content (34.24%, 41.10%, and 39.76%, respectively) (Fig. S9†). Identical experiments were performed in the absence of excitation light, and contrary to the poor effect of N-CDs on enhancing growth under excitation light-free conditions, N-CDs effectively alleviated the salt stress without excitation light. Without excitation light, there were also significant increases in fresh weight (12.84%, 27.55%, and 33.47%, respectively) and chlorophyll content (14.56%, 14.20%, and 27.41%, respectively), and decreases in relative conductivity (14.44%, 12.60%, and 13.46%, respectively) and MDA content (20.11%, 23.08%, and 25.95%, respectively) relative to the control (Fig. S10†). It is worth noting that with no salt stress (0 mM NaCl), the N-CD-induced enhanced growth was also found to be excitation light-dependent in this experiment (Fig. S9 and S10†), which was in agreement with the results in Fig. 3.
To further verify these results, mature Arabidopsis seedlings were irrigated with a 150 mM NaCl solution and cultivated in the presence or absence of excitation light. As shown in Fig. 4 and 5, N-CD-treated seedlings presented greater growth compared to the control groups under excitation light on/off conditions. With and without excitation light, the fresh weights of the rosette leaves increased by 43.29% and 34.22% and roots increased by 50.66% and 32.60%, respectively (Fig. 4C, D and 5C, D). Furthermore, chlorophyll contents increased by 22.59% and 10.45% with and without excitation light, respectively (Fig. 4F and 5F). Similar results were also found in the Fv/Fm values (Fig. 4E and 5E), which reflect the health status of photosynthetic apparatus.72 The chlorophyll fluorescence images in Fig. 4B and 5B show that N-CD-treatment performed higher Fv/Fm values, which indicated that N-CDs alleviated the damage to photosynthetic system caused by salt stress. Furthermore, the relative conductivity and MDA content, indirectly reflecting the extent of oxidative damage to the plant, exhibited significant decreases of 32.58% and 17.59%, and 16.13% and 28.84% when the excitation light was on and off, respectively (Fig. 4G, H and 5G, H). Collectively, these results suggested that N-CDs can effectively improve salt stress tolerance in an excitation light-independent manner. This improvement was slightly greater in the presence of excitation light, probably because N-CDs also enhanced seedling growth when exposed to excitation light.
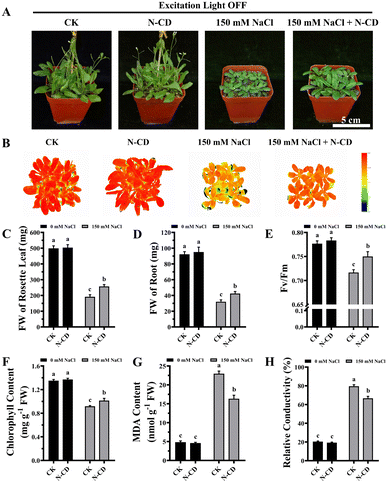 |
| Fig. 4 Effects of N-CDs in alleviating salt stress in the absence of excitation light. (A) Effects of N-CDs (300 mg L−1) on mature Arabidopsis seedlings in the mitigation of salt stress under light illumination without excitation light. Pictures captured after 14 days of cultivation. (B) Chlorophyll fluorescence images of all treatments. (C–H) Fresh weights of rosette leaves (C), fresh weights of roots (D), Fv/Fm values (E), chlorophyll contents (F), MDA contents (G), and relative conductivities (H) of all treatments. All data (n ≥ 3) were analyzed using one-way ANOVA, where different letters represent significant differences (P < 0.05, Tukey test). | |
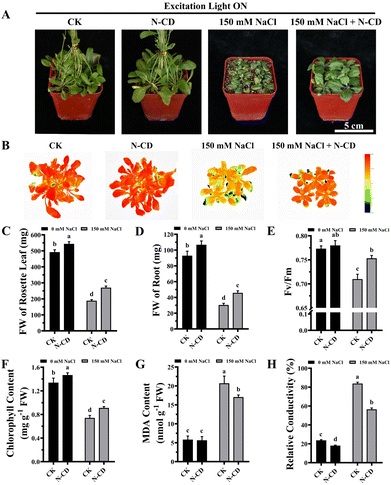 |
| Fig. 5 Effects of N-CDs in alleviating salt stress in the presence of excitation light. (A) Effects of N-CDs (300 mg L−1) on mature Arabidopsis seedlings in the mitigation of salt stress under light illumination with excitation light. Pictures captured after 14 days of cultivation. (B) Chlorophyll fluorescence images of all treatments. (C–H) Fresh weights of rosette leaves (C), fresh weights of roots (D), Fv/Fm values (E), chlorophyll contents (F), MDA contents (G), and relative conductivities (H) of all treatments. All data (n ≥ 3) were analyzed using one-way ANOVA, where different letters represent significant differences (P < 0.05, Tukey test). | |
Previous reports have indicated that the ROS scavenging capacity played a role in the CD-induced increase in stress tolerance.49,62 In this work, N-CDs enhanced salt tolerance independent of excitation light, suggesting that the ROS scavenging capacity of N-CDs is not related to their optical properties. In other words, the photo-generated electrons from light-excited N-CDs were not associated with their ROS scavenging capacity. Therefore, this ability probably originated from the intrinsic reduction capacity of N-CDs, such as the reductive raw materials of CDs. Recently, a mechanism for ROS-independent Ca2+ mobilization was proposed48 in which CDs served as a simultaneous Ca2+ signaling amplifier, with the hydroxyl and carboxyl groups on CDs contributing to this function. According to this mechanism, the optical properties of CDs should not be related to their Ca2+ mobilization ability and thereby stress tolerance role, in agreement with the results of this study. Taken together, N-CDs significantly enhance seedling growth and salt tolerance, but each role has different excitation light dependencies, indicating that N-CDs work via different pathways to achieve the two effects.
Conclusions
In this work, N-CDs prepared using the hydrothermal method had strong enhancing effects on seedling growth and salt stress tolerance. Furthermore, the N-CD-induced growth enhancement was found to have a dependence on excitation light, while the N-CD-improved seedling salt tolerance did not require excitation light. This work provides new insight into plant nanoscience, which will pave the way for further agricultural applications of nanomaterials.
Author contributions
The manuscript was designed and written through contributions of all authors. All authors have given approval to the final version of the manuscript.
Conflicts of interest
There are no conflicts to declare.
Acknowledgements
This work was financially supported by Major basic research projects of Shandong Province (ZR2020ZD43), National Natural Science Foundation of China (22207067), National Key Research and Development Program of China (2022YFD1201700), China Agriculture Research System of MOF and MARA (CARS-27), Taishan Industrial Leading Talents (LJNY202026) and Shandong Province Key R&D Program (2022TZXD008-02).
References
- L. M. Gilbertson, L. Pourzahedi, S. Laughton, X. Gao, J. B. Zimmerman, T. L. Theis, P. Westerhoff and G. V. Lowry, Nat. Nanotechnol., 2020, 15, 801–810 CrossRef CAS.
- D. R. Ort, S. S. Merchant, J. Alric, A. Barkan, R. E. Blankenship, R. Bock, R. Croce, M. R. Hanson, J. M. Hibberd, S. P. Long, T. A. Moore, J. Moroney, K. K. Niyogi, M. A. Parry, P. P. Peralta-Yahya, R. C. Prince, K. E. Redding, M. H. Spalding, K. J. van Wijk, W. F. Vermaas, S. von Caemmerer, A. P. Weber, T. O. Yeates, J. S. Yuan and X. G. Zhu, Proc. Natl. Acad. Sci. U. S. A., 2015, 112, 8529–8536 CrossRef CAS.
- G. V. Lowry, A. Avellan and L. M. Gilbertson, Nat. Nanotechnol., 2019, 14, 517–522 CrossRef CAS.
- National Academies of Sciences and Medicine, Science Breakthroughs to Advance Food and Agricultural Research by 2030, The National Academies Press, Washington, DC, 2019 Search PubMed.
- N. Ramankutty, Z. Mehrabi, K. Waha, L. Jarvis, C. Kremen, M. Herrero and L. H. Rieseberg, Annu. Rev. Plant Biol., 2018, 69, 789–815 CrossRef CAS PubMed.
- D. Tilman, C. Balzer, J. Hill and B. L. Befort, Proc. Natl. Acad. Sci. U. S. A., 2011, 108, 20260–20264 CrossRef CAS.
- J. K. Zhu, Cell, 2016, 167, 313–324 CrossRef CAS.
- A. E. Douglas, Annu. Rev. Plant Biol., 2018, 69, 637–660 CrossRef CAS PubMed.
- L. Holm, A. H. Malik and E. Johansson, J. Cereal Sci., 2018, 82, 230–242 CrossRef CAS.
- Y. Chen, T. Li, Q. Yang, Y. Zhang, J. Zou, Z. Bian and X. Wen, Front. Plant Sci., 2019, 10, 1563 CrossRef.
- H. Zhang, D.-p. Hou, X.-l. Peng, B.-j. Ma, S.-m. Shao, W.-j. Jing, J.-f. Gu, L.-j. Liu, Z.-q. Wang, Y.-y. Liu and J.-c. Yang, J. Integr. Agric., 2019, 18, 2716–2731 CrossRef.
- M. Nayak, D. K. Swain and R. Sen, Sci. Total Environ., 2019, 682, 475–484 CrossRef CAS PubMed.
- X.-K. Guan, L. Wei, N. C. Turner, S.-C. Ma, M.-D. Yang and T.-C. Wang, J. Cleaner Prod., 2020, 250, 119514 CrossRef CAS.
- J. Bailey-Serres, J. E. Parker, E. A. Ainsworth, G. E. D. Oldroyd and J. I. Schroeder, Nature, 2019, 575, 109–118 CrossRef CAS.
- L. Wang and Q. Zhang, Trends Plant Sci., 2017, 22, 643–646 CrossRef CAS PubMed.
- X. Huang, S. Yang, J. Gong, Q. Zhao, Q. Feng, Q. Zhan, Y. Zhao, W. Li, B. Cheng, J. Xia, N. Chen, T. Huang, L. Zhang, D. Fan, J. Chen, C. Zhou, Y. Lu, Q. Weng and B. Han, Nature, 2016, 537, 629–633 CrossRef CAS PubMed.
- J. Wu, S. J. Lawit, B. Weers, J. Sun, N. Mongar, J. Van Hemert, R. Melo, X. Meng, M. Rupe, J. Clapp, K. Haug Collet, L. Trecker, K. Roesler, L. Peddicord, J. Thomas, J. Hunt, W. Zhou, Z. Hou, M. Wimmer, J. Jantes, H. Mo, L. Liu, Y. Wang, C. Walker, O. Danilevskaya, R. H. Lafitte, J. R. Schussler, B. Shen and J. E. Habben, Proc. Natl. Acad. Sci. U. S. A., 2019, 116, 23850–23858 CrossRef CAS.
- D. F. Fiol, M. C. Terrile, J. Frik, F. A. Mesas, V. A. Álvarez and C. A. Casalongué, J. Chem. Technol. Biotechnol., 2021, 96, 2095–2108 CrossRef CAS.
- L. Zhao, L. Lu, A. Wang, H. Zhang, M. Huang, H. Wu, B. Xing, Z. Wang and R. Ji, J. Agric. Food Chem., 2020, 68, 1935–1947 CrossRef CAS.
- Q. Zhang, Y. Ying and J. Ping, Adv. Sci., 2022, 9, 2103414 CrossRef CAS.
- J. P. Giraldo, M. P. Landry, S. M. Faltermeier, T. P. McNicholas, N. M. Iverson, A. A. Boghossian, N. F. Reuel, A. J. Hilmer, F. Sen, J. A. Brew and M. S. Strano, Nat. Mater., 2014, 13, 400–408 CrossRef CAS.
- J. Du, B. Liu, T. Zhao, X. Xu, H. Lin, Y. Ji, Y. Li, Z. Li, C. Lu, P. Li, H. Zhao, Y. Li, Z. Yin and X. Ding, J. Nanobiotechnol., 2022, 20, 197 CrossRef CAS PubMed.
- D. Sun, H. I. Hussain, Z. Yi, J. E. Rookes, L. Kong and D. M. Cahill, Chemosphere, 2016, 152, 81–91 CrossRef CAS.
- H. Wu, N. Tito and J. P. Giraldo, ACS Nano, 2017, 11, 11283–11297 CrossRef CAS PubMed.
- L. Rossi, W. Zhang and X. Ma, Environ. Pollut., 2017, 229, 132–138 CrossRef CAS PubMed.
- L. Liang, S. C. Wong and G. Lisak, Chemosphere, 2023, 316, 137868 CrossRef CAS PubMed.
- P. Chauhan, M. Kumari, S. Chaudhary, G. R. Chaudhary, A. Umar and S. Baskoutas, Sci. Total Environ., 2022, 837, 155593 CrossRef CAS.
- B. Guo, G. Liu, W. Li, C. Hu, B. Lei, J. Zhuang, M. Zheng and Y. Liu, Ind. Crops Prod., 2022, 187, 115427 CrossRef CAS.
- Y. Yao, L. Yue, X. Cao, F. Chen, J. Li, B. Cheng, C. Wang and Z. Wang, ACS Appl. Nano Mater., 2023, 6, 110–118 CrossRef CAS.
- H. Li, J. Huang, F. Lu, Y. Liu, Y. Song, Y. Sun, J. Zhong, H. Huang, Y. Wang, S. Li, Y. Lifshitz, S.-T. Lee and Z. Kang, ACS Appl. Nano Mater., 2018, 1, 663–672 CrossRef CAS.
- Y. Gong and J. Zhao, J. Agric. Food Chem., 2018, 66, 9159–9161 CrossRef CAS PubMed.
- H. Wang, M. Zhang, Y. Song, H. Li, H. Huang, M. Shao, Y. Liu and Z. Kang, Carbon, 2018, 136, 94–102 CrossRef CAS.
- I. Milenkovic, M. Borisev, Y. Zhou, S. Z. Spasic, R. M. Leblanc and K. Radotic, J. Agric. Food Chem., 2021, 69, 5446–5451 CrossRef CAS PubMed.
- X. Xu, X. Mao, J. Zhuang, B. Lei, Y. Li, W. Li, X. Zhang, C. Hu, Y. Fang and Y. Liu, ACS Sustainable Chem. Eng., 2020, 8, 3938–3949 CrossRef CAS.
- E. Kou, Y. Yao, X. Yang, S. Song, W. Li, Y. Kang, S. Qu, R. Dong, X. Pan, D. Li, H. Zhang and B. Lei, ACS Sustainable Chem. Eng., 2021, 9, 944–953 CrossRef CAS.
- A. Wang, F. Kang, Z. Wang, Q. Shao, Z. Li, G. Zhu, J. Lu and Y. Y. Li, Adv. Sustainable Syst., 2019, 3, 1800132 CrossRef.
- Y. Li, X. Pan, X. Xu, Y. Wu, J. Zhuang, X. Zhang, H. Zhang, B. Lei, C. Hu and Y. Liu, J. Hazard. Mater., 2021, 410, 124534 CrossRef CAS PubMed.
- M. Zhang, L. Hu, H. Wang, Y. Song, Y. Liu, H. Li, M. Shao, H. Huang and Z. Kang, Nanoscale, 2018, 10, 12734–12742 RSC.
- H. Li, J. Huang, Y. Liu, F. Lu, J. Zhong, Y. Wang, S. Li, Y. Lifshitz, S.-T. Lee and Z. Kang, Nano Res., 2019, 12, 1585–1593 CrossRef CAS.
- J. Hu, W. Jia, X. Yu, C. Yan, J. C. White, J. Liu, G. Shen, S. Tao and X. Wang, Environ. Sci.: Nano, 2022, 9, 1651–1661 RSC.
- C. Wang, Y. Ji, X. Cao, L. Yue, F. Chen, J. Li, H. Yang, Z. Wang and B. Xing, ACS Nano, 2022, 16, 12415–12424 CrossRef CAS.
- Q. Chen, X. Cao, X. Nie, Y. Li, T. Liang and L. Ci, J. Hazard. Mater., 2022, 423, 127260 CrossRef CAS PubMed.
- Y. Ji, L. Yue, X. Cao, F. Chen, J. Li, J. Zhang, C. Wang, Z. Wang and B. Xing, Sci. Total Environ., 2023, 856, 159125 CrossRef CAS PubMed.
- H. Yang, C. Wang, F. Chen, L. Yue, X. Cao, J. Li, X. Zhao, F. Wu, Z. Wang and B. Xing, Sci. Total Environ., 2022, 809, 151105 CrossRef CAS.
- M. Zhong, L. Yue, Q. Chen, H. Wang, B. Lei, X. Yang and Y. Kang, Environ. Sci.: Nano, 2023, 10, 595–610 RSC.
- X. Luo, X. Cao, C. Wang, L. Yue, X. Chen, H. Yang, X. Le, X. Zhao, F. Wu, Z. Wang and B. Xing, Environ. Sci.: Nano, 2021, 8, 3806–3819 RSC.
- W. Elmer and J. C. White, Annu. Rev. Phytopathol., 2018, 56, 111–133 CrossRef CAS PubMed.
- Y. Li, Z. Tang, Z. Pan, R. Wang, X. Wang, P. Zhao, M. Liu, Y. Zhu, C. Liu, W. Wang, Q. Liang, J. Gao, Y. Yu, Z. Li, B. Lei and J. Sun, ACS Nano, 2022, 16, 4357–4370 CrossRef CAS PubMed.
- Y. Li, W. Li, X. Yang, Y. Kang, H. Zhang, Y. Liu and B. Lei, ACS Appl. Nano Mater., 2021, 4, 113–120 CrossRef CAS.
- Q. Chen, X. Cao, B. Liu, X. Nie, T. Liang, J. Suhr and L. Ci, J. Environ. Manage., 2021, 299, 113624 CrossRef CAS PubMed.
- D. Li, W. Li, H. Zhang, X. Zhang, J. Zhuang, Y. Liu, C. Hu and B. Lei, ACS Appl. Mater. Interfaces, 2020, 12, 21009–21019 CrossRef CAS PubMed.
- Z. Huang, B. Guo, Y. Zou, J. He, C. Hu, J. Zhuang and Y. Liu, ACS Agric. Sci. Technol., 2022, 2, 684–692 CrossRef CAS.
- C. Wang, H. Yang, F. Chen, L. Yue, Z. Wang and B. Xing, Environ. Sci. Technol., 2021, 55, 12317–12325 CrossRef CAS PubMed.
- Y. Li, X. Xu, B. Lei, J. Zhuang, X. Zhang, C. Hu, J. Cui and Y. Liu, Chem. Eng. J., 2021, 422, 130114 CrossRef CAS.
- T. A. Swift, D. Fagan, D. Benito-Alifonso, S. A. Hill, M. L. Yallop, T. A. A. Oliver, T. Lawson, M. C. Galan and H. M. Whitney, New Phytol., 2021, 229, 783–790 CrossRef CAS PubMed.
- L. D. Agnol, R. M. Neves, M. Maraschin, S. Moura, H. L. Ornaghi, F. T. G. Dias and O. Bianchi, Sustainable Mater. Technol., 2021, 30, e00347 CrossRef CAS.
- S. Chandra, S. Pradhan, S. Mitra, P. Patra, A. Bhattacharya, P. Pramanik and A. Goswami, Nanoscale, 2014, 6, 3647–3655 RSC.
- I. Nabiev, A. Rakovich, A. Sukhanova, E. Lukashev, V. Zagidullin, V. Pachenko, Y. P. Rakovich, J. F. Donegan, A. B. Rubin and A. O. Govorov, Angew. Chem., Int. Ed., 2010, 49, 7217–7221 CrossRef PubMed.
- W. Li, S. Wu, H. Zhang, X. Zhang, J. Zhuang, C. Hu, Y. Liu, B. Lei, L. Ma and X. Wang, Adv. Funct. Mater., 2018, 28, 1804004 CrossRef.
- Y. Wang, Z. Xie, X. Wang, X. Peng and J. Zheng, J. Nanobiotechnol., 2021, 19, 260 CrossRef CAS PubMed.
- E. Kou, W. Li, H. Zhang, X. Yang, Y. Kang, M. Zheng, S. Qu and B. Lei, ACS Appl. Bio Mater., 2021, 4, 6093–6102 CrossRef CAS PubMed.
- Y. Li, J. Gao, X. Xu, Y. Wu, J. Zhuang, X. Zhang, H. Zhang, B. Lei, M. Zheng, Y. Liu and C. Hu, ACS Appl. Mater. Interfaces, 2020, 12, 33575–33585 CrossRef CAS PubMed.
- Y. Li, W. Li, H. Zhang, Y. Liu, L. Ma and B. Lei, Nanoscale, 2020, 12, 155–166 RSC.
- X. Pan, D. Li, Y. Fang, Z. Liang, H. Zhang, J. Z. Zhang, B. Lei and S. Song, J. Phys. Chem. Lett., 2020, 11, 1822–1827 CrossRef CAS PubMed.
- B. Vercelli, R. Donnini, F. Ghezzi, A. Sansonetti, U. Giovanella and B. La Ferla, Electrochim. Acta, 2021, 387, 138557 CrossRef CAS.
- S. He, M. J. Turnbull, Y. Nie, X. Sun and Z. Ding, Surf. Sci., 2018, 676, 51–55 CrossRef CAS.
- M. M. Ali, R. Anwar, M. W. Shafique, A. F. Yousef and F. Chen, Agronomy, 2021, 11, 224 CrossRef CAS.
- M. Dubois, Nature, 1951, 168, 167 CrossRef CAS PubMed.
- D. I. Arnon, Plant Physiol., 1949, 24, 1–15 CrossRef CAS PubMed.
- H. H. Draper, Free Radical Biol. Med., 1993, 15, 353–363 CrossRef CAS PubMed.
- S. Lutts, J. M. Kinet and J. Bouharmont, Ann. Bot., 1996, 78, 389–398 CrossRef CAS.
- Y. Liu, S. Zhang, F. Yang, G. Wang, X. Jing, X. Wang and C. You, RSC Adv., 2021, 11, 26620–26628 RSC.
- J. Liu, Y. Geng, D. Li, H. Yao, Z. Huo, Y. Li, K. Zhang, S. Zhu, H. Wei, W. Xu, J. Jiang and B. Yang, Adv. Mater., 2020, 32, 1906641 CrossRef CAS PubMed.
- J. Manioudakis, F. Victoria, C. A. Thompson, L. Brown, M. Movsum, R. Lucifero and R. Naccache, J. Mater. Chem. C, 2019, 7, 853–862 RSC.
- M. Esmaeili, Z. Wu, D. Chen, A. Singh, P. Sonar, D. Thiel and Q. Li, Adv. Powder Technol., 2022, 33, 103560 CrossRef CAS.
- X. Yan, J. Wang, D. Li, J. Feng, Q. Xu, H. Chen and R. Han, Environ. Sci.: Nano, 2021, 8, 1352–1363 RSC.
- X. Yan, Q. Xu, D. Li, J. Wang and R. Han, Ecotoxicol. Environ. Saf., 2021, 216, 112168 CrossRef CAS PubMed.
|
This journal is © The Royal Society of Chemistry 2023 |
Click here to see how this site uses Cookies. View our privacy policy here.