DOI:
10.1039/D3RA02946H
(Paper)
RSC Adv., 2023,
13, 18443-18449
Balancing “on” and “off” response of hydroxyl groups to a nanozyme-catalyzing system for the construction of an ultra-sensitive and selective “signal-on” detection platform for dopamine†
Received
4th May 2023
, Accepted 30th May 2023
First published on 19th June 2023
Abstract
Targeting the functional groups present in analytes by nanozyme-catalyzed systems is a promising strategy to construct sensitive and selective platforms for the sensing of specific analytes. Herein, various groups (–COOH, –CHO, –OH, and –NH2) on benzene were introduced in an Fe-based nanozyme system with MoS2–MIL-101(Fe) as the model peroxidase nanozyme, H2O2 as the oxidizing agent, and TMB as the chromogenic substrate, and the effects of these groups at both a low concentration and high concentration were further investigated. It was found that the hydroxyl group-based substance catechol showed an “on” effect at a low concentration to increase the catalytic rate and enhance the absorbance signal, whereas an “off” effect at a high concentration with a decreased absorbance signal. Based on these results, the “on” mode and “off” mode for the biological molecule dopamine, a type of catechol derivative, were proposed. In the control system, MoS2–MIL-101(Fe) catalyzed the decomposition of H2O2 to produce ROS, which further oxidized TMB. In the “on” mode, the hydroxyl groups of dopamine could combine with the Fe(III) site of the nanozyme to lower its oxidation state, resulting in higher catalytic activity. In the “off” mode, the excess dopamine could consume ROS, which inhibited the catalytic process. Under the optimal conditions, by balancing the “on” and “off” modes, the “on” mode for the detection of dopamine was found to have better sensitivity and selectivity. The LOD was as low as 0.5 nM. This detection platform was successfully applied for the detection of dopamine in human serum with satisfactory recovery. Our results can pave the way for the design of nanozyme sensing systems with sensitivity and selectivity.
1 Introduction
Natural enzymes with high catalytic activity and precise substrate specificity have been widely used in the field of analytical sensing.1–3 However, they also show the intrinsic disadvantages of easy inactivation, difficult purification, and high cost, prompting research on the development of stable and cheap alternatives to natural enzymes. As a new generation of enzyme alternatives, nanozymes, which are defined as nanomaterials with enzyme-like activity, can overcome the disadvantages of natural enzymes, while retaining the unique advantages of nanomaterials such as tunable catalytic activity and type and optical, magnetic, and electrical properties, which have attracted extensive interest in recent years.4–6 However, compared with natural enzymes, nanozymes exhibit limited catalytic activity and specificity, which restrict their practical application for the construction of analytical sensing platforms with sensitivity and selectivity. Thus, it is highly desirable to develop efficient modes to achieve highly sensitive and selective sensing for specific analytes based on nanozyme systems.
For high-quality sensing, extensive efforts have been devoted to the rational design of nanozyme structures, including altering their size,7 controlling their morphology,8 surface modification,9 and changing their chemical composition.10 Among them, modification of the surface functional groups is an efficient approach to obtain nanozymes with a superior catalytic performance.11–14 Qu's group measured the peroxidase-like activity of graphene quantum dots after selectively deactivating their ketonic carbonyl, carboxylic, or hydroxyl groups and concluded that the –C
O groups acted as the catalytically active sites, the O
C–O– groups as the substrate-binding sites, and the –C–OH groups inhibited the activity.11 Hwang's group functionalized the MIL-100(Fe) nanozyme by grafting various aliphatic diamines on its coordinatively unsaturated metals sites and found that the enhanced peroxidase-like activity of amine-functionalized MIL-100(Fe) is due to the synergistic effect of the enhanced negative potential and precisely controlled molecular size of the grafted diamines.12 Zhu's group introduced two functional groups (nitro and amino) in MIL-101(Fe) to tune its atomically dispersed metal active sites and demonstrated that functionalization with nitro (–NO2) increased the peroxidase-like activity of MIL-101(Fe), whereas amino (–NH2) decreased it.13 Wei's group investigated the oxidase-like activity of a series of substituted MIL-53(Fe) with similar coordination. A Hammett-type structure–activity linear free energy relationship was observed, indicating that an increase in the Hammett sigma(m) value with electron-withdrawing ligands increases the oxidase-like activity. MIL-53(Fe)–NO2 with the strongest electron-withdrawing NO2 substituent showed a ten-fold higher activity than the unsubstituted MIL-53(Fe).14 Most reports focused on the design of functional groups on nanozymes for the improvement of their catalytical performance, which is attractive for analytical sensing. Alternatively, the analytes being detected also have rich functional groups, which can significantly affect the activity of nanozymes, and thus be considered in the construction of analytical sensing platform with high sensitivity and selectivity. To date, to the best of our knowledge, research on nanozymes and the functional groups on the analytes is rare.
Inspired by these facts, herein, we investigated the response of a nanozyme to various functional groups (–COOH, –CHO, –OH, and –NH2) to construct a sensing platform for specific analytes instead of anchoring these group on the nanozyme to design a high-quality nanozyme for sensing. Herein, MoS2–MIL-101(Fe) was selected as the peroxidase nanozyme because it has intrinsic unsaturated metal sites for coordinating functional group and MoS2 can increase the catalytic activity of MIL-101(Fe).15 Catechol (Ph–(OH)2), phthalic acid (Ph–(COOH)2), 1,2-phthalic dicarboxaldehyde (Ph–(CHO)2), and o-phenylenediamine (Ph–(NH2)2) were used as representatives with hydroxyl, carboxyl, aldehyde, and amino groups, respectively. The typical chromogenic agent 3,3′,5,5′-tetramethylbenzidine (TMB) was chosen as the peroxidase substrate. The peroxidase-like activity of MoS2–MIL-101(Fe) was evaluated by testing its catalytic ability to convert TMB into the blue-colored oxidized TMB in the presence of H2O2. The effect of Ph–(OH)2, Ph–(COOH)2, Ph–(CHO)2, Ph–(NH2)2 on the MoS2–MIL-101(Fe)-catalyzed H2O2–TMB system was investigated. It was found that the “on” effect and “off” effect on the nanozyme-catalyzed system could simultaneously exist for one substance (Ph–(OH)2, catechol) at different concentration levels. Dopamine, a derivative of Ph–(OH)2 catechol, was selected as the analyte. Based on the results, the “on” mode and “off” mode for the dopamine based on MoS2–MIL-101(Fe)-catalyzed H2O2–TMB system were proposed due to the “signal-on” effect with less DA and “signal-off” effect with excess DA (Scheme 1). In addition, the sensitivity and selectivity of the “on” mode and “off” mode were balanced and applied for the analysis of practical serum samples.
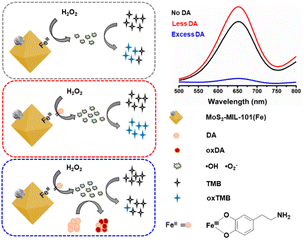 |
| Scheme 1 Schematic diagram of the “signal-on” mode and “signal-off” mode of MoS2–MIL-101(Fe)–H2O2–TMB system for less DA and excess DA. | |
2 Experimental
2.1 Reagents
TMB and ammonium thiomolybdate ((NH4)2MoS4) were purchase from Sigma-Aldrich Chemical Reagent Co., Ltd. (USA). Ferric chloride hexahydrate (FeCl3·6H2O) was obtained from Kermel Chemical Co., Ltd. (Tianjin, China). Ethanol, acetic acid (HAc) and hydrogen peroxide were provided by Chuandong Chemical Reagents Company (Chongqing, China). N,N′-Dimethylformamide (DMF) was purchased from Kelong Chemical Reagent Co., Ltd. (Chengdu, China). 1,2-Phthalic dicarboxaldehyde was provided by Sinopharm Chemical Reagent Co., Ltd. (Beijing, China). NaCl, KCl, catechol, phthalic acid, o-phenylenediamine, sodium acetate (NaAc), terephthalic acid, dopamine, glucose, uric acid, alanine, phenylalanine, arginine, proline, histidine, homocysteine, glutathione, and ascorbic acid were provided by Shanghai Titan Scientific Co., Ltd. (Shanghai, China). Serum samples were provided by the University Hospital (Fuling, Chongqing). Dopamine hydrochloride injections were obtained from a chemist's shop.
2.2 Instruments
Scanning electron microscopy (SEM) images of the nanozyme were recorded on a scanning electron microscope (JEOL, Japan). The transmission electron microscopy (TEM) images of the nanozyme were recorded on a TF20 field-emission transmission electron microscope at 200 kV (FEI, USA). The X-ray diffraction (XRD) patterns of the nanozyme were recorded on a Ultima IV powder X-ray diffractometer (Rigaku, Japan). The UV-vis spectra of the nanozyme catalyzed systems were recorded on a U-4100 spectrophotometer (Hitachi, Japan).
2.3 Preparation of MoS2–MIL-101(Fe) nanocomposite
The MoS2–MIL-101(Fe) nanocomposite was prepared based on our previous work.15
2.4 Determination of dopamine
The typical process for evaluating MoS2–MIL-101(Fe) as a peroxidase mimetic was performed in acetate buffer solution (2 mL, 0.2 M, pH 3.0) composed of 0.25 mL MoS2–MIL-101(Fe) (50 mg L−1), 0.10 mL TMB (2.5 mM in EtOH), and 0.15 mL H2O2 (1 mM). The MoS2–MIL-101(Fe)-catalyzed system was incubated at 30 °C for 20 min and then its absorption spectrum was recorded using a UV-vis spectrophotometer.
A typical process for the “signal-on” mode for the determination of dopamine based on MoS2–MIL-101(Fe) as a peroxidase mimetic was carried out by measuring the UV-vis spectra of solutions composed of acetate buffer solution (1.9 mL, 0.2 M, pH 4.0), 0.25 mL MoS2–MIL-101(Fe) (50 mg L−1), 0.10 mL TMB (2.5 mM in EtOH), 0.15 mL H2O2 (1 mM) in the presence of 0.10 mL different concentrations of dopamine after incubation at 30 °C for 15 min. The typical process for the “signal-off” mode was conducted by recording the UV-vis spectra of the same solutions as that in the “signal-on” mode after incubation at 40 °C for 25 min.
For practical use of the “signal-on” mode for dopamine determination, five serum samples taken from the University Hospital and two dopamine hydrochloride injections from a chemist's shop were chosen. In the case of the former, the serum samples were centrifuged at 10
000 rpm for 10 min, and then the obtained supernatant was diluted 20 times for further use. The next operation was the same as that for the typical “signal-on” dopamine determination except that the diluted serum supernatant was used in place of dopamine solution. In the case of the latter, the two dopamine hydrochloride injections were diluted 20
000 times and 10
000 times to the detectable range of “signal-on” mode, respectively. The diluted solution replaced dopamine solution for further determination.
3 Results and discussion
3.1 Characterisation of MoS2–MIL-101(Fe)
MoS2–MIL-101(Fe) was synthesized by growing MIL-101(Fe) crystals in the presence of as-prepared MoS2. As shown in Fig. 1A and B, the MoS2–MIL-101(Fe) composite appears as MoS2 nanoparticles anchored on MIL-101(Fe) octahedra. The size of MIL-101(Fe) is not very uniform with an average size of 2.29 μm and a size in the range of 0.74 to 6.08 μm (Fig. 1A and S1†). The element mapping demonstrated the presence of Fe, O, C, Mo, and S in the composite and confirmed the structure of MoS2 nanoparticles anchored around MIL-101(Fe) (Fig. 1C). The XRD pattern of MoS2–MIL-101(Fe) is consistent with that of simulated MIL-101(Fe) (Fig. 1D), confirming the existence of MIL-101(Fe). Besides, the obvious characteristic peak at about 9.6° is attributed to MoS2, indicating that MoS2 was also maintained in the composite during the growth process of MIL-101(Fe).
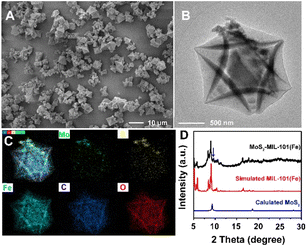 |
| Fig. 1 (A) SEM image of MoS2–MIL-101(Fe). (B) TEM image of MoS2–MIL-101(Fe). (C) Element mapping of MoS2–MIL-101(Fe). (D) XRD pattern of MoS2–MIL-101(Fe). | |
3.2 The effect of groups on MoS2–MIL-101(Fe) nanozyme-catalyzed system
In our previous report, MoS2–MIL-101(Fe) was demonstrated to have peroxidase-like activity to catalyze the oxidation of TMB by H2O2 accompanied with an adsorption at 652 nm for the oxidized TMB.15 Herein, the effect of different groups with a concentration in the range of 0.5–50 μM on the MoS2–MIL-101(Fe) nanozyme system was investigated. Phthalic acid (Ph–(COOH)2), 1,2-phthalic dicarboxaldehyde (Ph–(CHO)2), catechol (Ph–(OH)2), and o-phenylenediamine (Ph–(NH2)2) were used as representatives with carboxyl, aldehyde, hydroxyl, and amino groups, respectively. As shown in Fig. 2, an increase in Ph–(COOH)2 concentration did not accelerate or decelerate the MoS2–MIL-101(Fe) nanozyme-catalyzed H2O2–TMB reaction, while an increase in the concentration of Ph–(CHO)2 and Ph–(OH)2 at a relatively high range (higher than 8 μM) and an increase in the concentration of Ph–(NH2)2 inhibited the signal of the MoS2–MIL-101(Fe)–H2O2–TMB reaction system. Moreover, the inhibition of this catalytic redox reaction increased in the order of Ph–(COOH)2, Ph–(CHO)2, Ph–(OH)2 and Ph–(NH2)2, which can be attributed to the order of the reducing ability of these groups (–COOH < –CHO < –OH < –NH2). In addition, Ph–(OH)2 at a low concentration of 0.5–8 μM exhibited a promotion effect on the MoS2–MIL-101(Fe)–H2O2–TMB reaction system, which differs from other representatives with groups at a low concentration of 0.5–8 μM (unchanged effect for Ph–(COOH)2 and Ph–(CHO)2, and inhibition effect for Ph–(NH2)2). The interaction of MoS2–MIL-101(Fe) with Ph–(NH2)2, Ph–(OH)2, Ph–(CHO)2, and Ph–(COOH)2 was investigated through UV-vis spectroscopy (Fig. S2†). Ph–(NH2)2 and Ph–(OH)2 can efficiently coordinate with the unsaturated FeIII site of MoS2–MIL-101(Fe) due to the obvious increasing signal at about 240 nm with time, which resulted from the ligand to metal charge transfer (LMCT) after the addition of Ph–(NH2)2 and Ph–(OH)2 to MoS2–MIL-101(Fe), while inconspicuous changes were observed in the presence of Ph–(CHO)2 and Ph–(COOH)2, indicating the absence of or weak coordination effect. A concentration lower than 0.5 μM of Ph–(NH2)2 could also increase the absorbance signal (Fig. S3†). The above-mentioned results suggest that the formation of coordination compound groups such as –OH and –NH2 at low concentration results in improved catalytic activity for the “on” effect. Simultaneously, substances with functional groups can also consume the reactive oxygen species (ROS, ˙OH and O2˙−) produced by MoS2–MIL-101(Fe) catalyzing H2O2,15–18 resulting in a “off” effect. Thus, the “on” effect and “off” effect depend on the balance between the groups influencing the catalyst and groups influencing the catalytic reaction. Among the substances with groups, Ph–(OH)2 exhibited a distinct “on” effect on the reaction system at a low concentration and “off” effect at a high concentration, which is promising to develop two analytical modes for substances with hydroxyl groups.
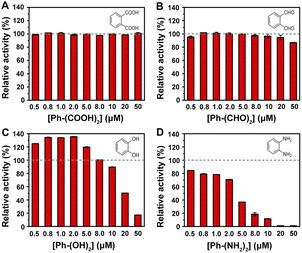 |
| Fig. 2 Effect of Ph–(COOH)2 (A), Ph–(CHO)2 (B), Ph–(OH)2 (C) and Ph–(NH2)2 (D) with the concentration range of 0.5–50 μM on MoS2–MIL-101(Fe)-catalyzed H2O2–TMB system. The grey dash line indicates the criterion of the activity of MoS2–MIL-101(Fe) in the H2O2–TMB system. The relative activity (%) means the ratio of the activity of the MoS2–MIL-101(Fe)–H2O2–TMB system in the presence of various concentrations of representatives to that in the absence of these representatives. | |
3.3 Dopamine detection for “on” and “off” modes based on MoS2–MIL-101(Fe) nanozyme
Dopamine (DA) is an important neurotransmitter with the function of regulating the central nervous system, and its abnormal concentration in the human body is related to many diseases such as Parkinson's disease, schizophrenia, and Tourette syndrome. Thus, the detection of DA with high accuracy has considerable significance for the early diagnosis of diseases. DA contains a catechol structure with two hydroxyl groups on the benzene ring, which makes it possible to monitor the DA concentration though the MoS2–MIL-101(Fe) nanozyme system. As seen in Fig. S4A,† similar to catechol, a low concentration of DA at 0.5 μM could trigger this chromogenic reaction to produce a deeper absorption at 652 nm, whereas a high concentration at 50 μM could lower this optical signal to obtain a weak absorption at 652 nm. In less DA, a complex of DA and MoS2–MIL-101(Fe) was formed, which exhibited higher reactivity with H2O2 to produce more ROS for the oxidation of with a higher absorption at 652 nm. The kinetic behaviours of MoS2–MIL-101(Fe) and DA-coordinated MoS2–MIL-101(Fe) were studied by measuring the initial reaction rate by varying one substance (H2O2 or TMB) and fixing another substance (TMB or H2O2) (Fig. S5†). Their kinetic curves were well fitted by the Michaelis–Menten equation to obtain their apparent kinetic parameters (Km and Vmax). After complexing with DA, MoS2–MIL-101(Fe) showed a lower Km for TMB, indicating its stronger affinity with TMB. It was deduced that the existence of DA increased the π–π interaction to enhance its affinity with TMB, further improving the catalytic ability of the H2O2–TMB system. In excess DA, the fluorescence emission observed at 530 nm in the DA–MoS2–MIL-101(Fe)–H2O2 system is attributed to the oxidation product of DA,19 and the existence of TMB could impair the formation of the DA oxidation product (Fig. S4B†), indicating the competition of TMB and DA as peroxidase mimetic substrates. It was deduced that a high concentration of DA can saturate the uncoordinated FeIII site of MoS2–MIL-101(Fe) and excess DA will consume the ROS produced by the DA-coordinated MoS2–MIL-101(Fe)-catalyzed H2O2 system to obtain the oxidized form of DA (Scheme 1). The competition between DA and TMB to consume ROS caused a decrease in the generation of oxidized TMB with a low absorption at 652 nm. Considering the molecular formula of dopamine with the presence of both hydroxyl and amino groups (Fig. S6A†), we investigated the effect of phenylethylamine (Ph–CH2–CH2–NH2) (which has the structure of dopamine without the two hydroxyl groups, as shown in Fig. S6A†) in the concentration range of 0.5–50 μM on the MoS2–MIL-101(Fe)-catalyzed H2O2–TMB system (Fig. S6B†). It was found that Ph–CH2–CH2–NH2 had no impact on the system, which could eliminate the effect of the amino group in dopamine on the “signal-on” effect and “signal-off” effect, and further confirmed the effect of hydroxyl groups on the “signal-on” effect and “signal-off” effect. The differentiation of phenylethylamine in the catalytic system (inconspicuous change) with o-phenylenediamine (strong inhibition) originates from the different positions of the amino group. Compared with a carbon chain, benzene can provide a richer electron cloud for the amino group, and thus the amino groups bonded with benzene are more likely to be oxidized. Specifically, the amino group bonded with benzene or other benzene-like structure (aromatic amine) has much stronger reducing ability than the amino group bonded with a carbon chain (aliphatic amine), which makes this differentiation. Based on these results, the “on” mode and “off” mode for the detection of dopamine based on the MoS2–MIL-101(Fe)-catalyzed H2O2–TMB system are proposed.
The “signal-on” effect of 0.5 μM DA on the nanozyme-catalyzed system with a change in pH, temperature, nanozyme concentration and reaction time was investigated to acquire the optimal conditions for DA detection in the “signal-on” mode. The responses of the “signal-on” mode for DA in the pH range of 2.5–7.0, temperature range of 10–60 °C, nanozyme concentration range of 5–30 mg L−1, reaction time of 5–60 min were studied, as shown in Fig. S7A–D,† respectively. The response for DA was evaluated through the difference (A − A0) between the absorbance of the nanozyme system in the presence of DA (A) and that in the absence of DA (A0). The response reached the maximum under the conditions of pH of 4.0, temperature of 30 °C, nanozyme concentration of 5 mg L−1 and reaction time of 15 min. Under the optimal conditions, the absorption at 652 nm of the reaction system increased with an increase in DA concentration in the range of 0–500 nM (Fig. 3A), which indicates the typical “signal-on” mode. The enhanced absorbance difference (A − A0) at 652 nm displayed a good linear relationship with the DA concentration of 0.5 to 20 nM (Fig. 3B). The linear regression equation is A = 0.0013[DA] + 0.4422 (R2 = 0.9991, n = 6) with a limit of detection (LOD) of 0.5 nM. In addition, the good linear relationship can also be monitored in the DA range of 20–500 nM (Fig. 3B) with a linear regression equation of A = 0.0002[DA] + 0.4690 (R2 = 0.9930, n = 7).
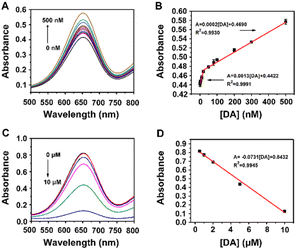 |
| Fig. 3 (A) UV-vis spectra and (B) absorbance response of MoS2–MIL-101(Fe)–H2O2–TMB system in different concentrations of DA in the “signal-on” mode. (C) UV-vis spectra and (D) absorbance response of MoS2–MIL-101(Fe)–H2O2–TMB system in different concentrations of DA in the “signal-off” mode. | |
In the presence of 50 μM DA, the nanozyme-catalyzed system displayed the “signal-off” effect. The responses of the “signal-off” mode for 50 μM DA in the pH range of 2.5–7.0, temperature range of 10–60 °C, the nanozyme concentration range of 5–30 mg L−1, and reaction time of 5–60 min were also studied in Fig. S8A–D,† respectively. The response for DA was evaluated through the difference (A0 − A) between the absorbance of the nanozyme system in the presence of DA (A) and that in the absence of DA (A0). The maximum response was observed under the conditions of pH of 4.0, temperature of 40 °C, nanozyme concentration of 5 mg L−1 and reaction time of 25 min. Under the optimal conditions, the absorption at 652 nm of the reaction system decreased with an increase in the concentration of DA in the range of 0–10 μM (Fig. 3C), which indicates the typical “signal-off” mode. The inhibited absorbance difference (A0 − A) at 652 nm displayed a good linear relationship with a DA concentration of 0.5 to 10 μM (Fig. 3D). The linear regression equation is A = −0.0731[DA] + 0.8432 (R2 = 0.9945, n = 5) with a limit of detection (LOD) of 0.5 μM.
The detectable ranges of the “signal-on” mode and “signal-off” mode were at the nanomole and micromole levels, respectively, which suggests the higher sensitivity of the “signal-on” mode over the “signal-off” mode. The “signal-on” mode is attributed to the role of the target DA as a catalyst promotor, whereas the “signal-off” mode is ascribed to its role as the catalytic reaction inhibitor. In this system, the strong coordination ability of the hydroxyl groups in DA to the MoS2–MIL-101(Fe) catalyst is balanced with its reduction ability in the MoS2–MIL-101(Fe)-catalyzed H2O2–TMB reaction. The analytical performance of the proposed “signal-on” mode and “signal-off” mode for the detection of DA based on the MoS2–MIL-101(Fe) system was compared with other reported methods based on nanozyme systems. It was found that the limit of detection (LOD) of the “signal-on” mode is lower than that obtained from other methods (Table 1),20–31 indicating the superiority of the proposed “signal-on” method for sensing DA in terms of sensitivity.
Table 1 Comparison of the proposed methods of the MoS2–MIL-101(Fe)-catalyzed system with a “signal-on” mode and “signal-off” mode with other reported methods with nanozyme systems
Nanozyme |
Method |
Mode |
Linear range (μM) |
LOD (μM) |
Ref. |
Fe3O4@Au |
Electrochemistry |
Signal-on |
10–1000 |
0.058 |
20 |
rGO–Co3O4 |
Electrochemistry |
Signal-on |
0–30 |
0.277 |
21 |
Fe–MIL-88 |
Fluorometry |
Signal-on |
0.05–30 |
0.046 |
22 |
β-CD@AuNCs |
Fluorometry |
Signal-off |
0.1–80 |
0.02 |
23 |
Fe3O4 NPs |
Colorimetry |
Signal-on |
0.010–4.0 |
0.0035 |
24 |
Fe/NC-800 |
Colorimetry |
Signal-off |
0.01–40 |
0.01 |
25 |
PtNPs@MnO2 |
Colorimetry |
Signal-off |
0.1–1.1 |
0.025 |
26 |
h-CuS NCs |
Colorimetry |
Signal-off |
2–150 |
1.67 |
27 |
Pt/hBNNSs-5 |
Colorimetry |
Signal-off |
2–55 |
0.76 |
28 |
[Cu(PDA)(DMF)] |
Colorimetry |
Signal-off |
10–100 |
ND |
29 |
Por–CuCo2O4 |
Colorimetry |
Signal-off |
10–100 |
0.94 |
30 |
Pt/CoSn(OH)6 |
Colorimetry |
Signal-off |
5–60 |
0.76 |
31 |
MoS2–MIL-101(Fe) |
Colorimetry |
Signal-on |
0.0005–0.02, 0.02–0.5 |
0.0005 |
This work |
MoS2–MIL-101(Fe) |
Colorimetry |
Signal-off |
0.5–10 |
0.5 |
This work |
For practical application, selectivity experiments of the proposed “signal-on” and “signal-off” modes were also conducted by testing the response of DA and various substances (including NaCl, KCl, glucose (Glu), uric acid (UA), alanine (Ala), phenylalanine (Phe), arginine (Arg), proline (Pro), histidine (His), homocysteine (Hcy), glutathione (GSH), and ascorbic acid (AA)) at the same concentration. The DA response was not interfered by the other tested substances in the “signal-on” mode due to the strong coordination ability of the hydroxyl groups in DA with MoS2–MIL-101(Fe) (Fig. 4A), whereas the DA response was partially affected by some tested substances with reducing ability (such as Hcy, GSH, and AA) due to the non-specific function of the redox catalytic reaction (Fig. 4B). It was noted that GSH showed a higher response than DA. GSH is type of tripeptide, which is constructed by glycine (Gly), glutamic acid (Glut) and cysteine (Cys) (Fig. S9A†). Thus, the response of Gly, Glut and Cys was also investigated (Fig. S9B†). It was found that Cys has obvious inhibition response, which is similar with GSH and higher than DA, while Gly and Glut have no obvious effect on this system. Gly and Glut have functional groups including carboxyl groups and amino groups on their carbon chains, and Cys has thiol functional groups besides carboxyl groups and amino groups. Therefore, the thiol group in GSH is the reason for the high response of the “signal-off” effect. The higher selectivity for GSH than DA is caused by the stronger reducing ability of the thiol group than hydroxyl group. Sulfur and oxygen belong to the same main group of elements, and the atomic radius of oxygen atom is greater than that of sulfur atom; thus, the sulfur atom has weaker binding ability to the outer electrons, which makes it more likely to lose electrons and be oxidized. These results confirm the higher selectivity of the “signal-on” mode over “signal-off” mode.
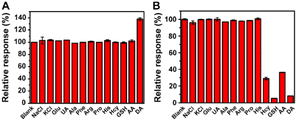 |
| Fig. 4 (A) Selectivity experiment of the “signal-on” mode to DA and various substances at 0.5 μM. (B) Selectivity experiment of the “signal-off” mode to DA and various substances at 50 μM. | |
By balancing the “signal-on” mode and “signal-off” mode for the detection of DA, it was found that the “signal-on” mode is superior to the “signal-off” mode based on its milder reaction temperature (30 °C), shorter reaction time (15 min), and higher sensitivity and selectivity, which is applicable for practical samples.
3.4 Dopamine detection in human serum samples and dopamine hydrochloride injections using the “signal-on” mode
Human serum samples were employed to evaluate the applicability of the “signal-on” mode based on the MoS2–MIL-101(Fe) nanozyme for detecting DA in real samples. After these samples were pre-treated according to the steps in the Experimental section, they were spiked with DA standard solution and utilized for the analysis of DA. As can be seen in Table 2, satisfactory recoveries of 91.54–112.31% were acquired for detecting DA in the human serum samples by using this “signal-on” mode. In addition, the content of dopamine in two dopamine hydrochloride injections is also measured by “signal-on” mode (Table S1†), obtaining a consistent result with the labelled value given by the manufacturers. These results indicate the feasibility and reliability of the proposed “signal-on” mode for the detection of DA.
Table 2 Real sample analysis through the signal-on mode based on MoS2–MIL-101(Fe) as peroxidase mimeticsa
Sample |
Detected (nM) |
Spiked (nM) |
Found (nM) |
Recovery (%) |
RSD (%) (n = 3) |
N.D. means not detected. |
1 |
N.D. |
100 |
98.53 |
98.53 |
6.91 |
300 |
302.20 |
100.73 |
1.63 |
500 |
509.51 |
98.24 |
3.66 |
2 |
N.D. |
100 |
97.31 |
97.31 |
4.88 |
300 |
287.56 |
95.85 |
4.88 |
500 |
476.59 |
95.31 |
3.17 |
3 |
N.D. |
100 |
92.44 |
92.43 |
2.44 |
300 |
319.27 |
106.42 |
3.25 |
500 |
468.05 |
93.61 |
1.46 |
4 |
N.D. |
5 |
5.62 |
112.31 |
7.69 |
10 |
10.10 |
101.03 |
4.44 |
20 |
18.31 |
91.54 |
6.66 |
5 |
N.D. |
5 |
5.23 |
104.62 |
15.38 |
10 |
10.23 |
102.31 |
11.54 |
20 |
18.31 |
91.54 |
7.69 |
4 Conclusion
In summary, the response of various functional groups (–COOH, –CHO, –OH, and –NH2) to the MoS2–MIL-101(Fe) nanozyme-catalyzed H2O2–TMB colorimetric system was investigated. The substance with –OH groups (catechol) at a low concentration could increase the signal of the catalytic system due to the strong coordination ability of the –OH groups with MoS2–MIL-101(Fe), and catechol at a high concentration decreased the signal due to its reduction ability. The detection of DA with a catechol-like structure was proposed based on the “signal-on” mode and “signal-off” mode. By balancing these two modes, the “signal-on” mode was found to have a milder reaction temperature, shorter reaction time, and higher sensitivity and selectivity. The detectable range realized was at the nanomole level, and the LOD was as low as 0.5 nM. In addition, the proposed mode for the detection of DA could be applied to human serum samples with satisfactory recoveries and commercial injections with good accuracy. Our results will pave a pathway for designing ultra-sensitive and selective methods for clinical diagnosis.
Author contributions
Hongmei Lan: investigation, methodology, writing – original draft. Gaoya Li: investigation, methodology, validation. Guo Chen: writing – original draft, visualization. Mengyao Ding: investigation, validation. Shuanglin Xiao: investigation. Jianglin Xiang: investigation. Xingwu Duan: resources. Haiyan Cao: writing – reviewing and editing. Wenbing Shi: resources, writing – reviewing and editing. Wenfei Dong: supervision, conceptualization, methodology, writing – reviewing and editing.
Conflicts of interest
There are no conflicts to declare.
Acknowledgements
The work was supported by the Natural Science Foundation of Chongqing City (cstc2020jcyj-msxmX0430), the Undergraduate Innovation and Entrepreneurship Training Project of Chongqing City (S202110647014), the Postgraduate Scientific Research Innovation Project of Hunan Province (CX20210170) and the Fundamental Research Funds for the Central Universities of Central South University (2021zzts0069).
Notes and references
- S. Ye, Y. Mao, Y. Guo and S. Zhang, TrAC, Trends Anal. Chem., 2014, 55, 43–54 CrossRef CAS.
- X.-m. Chen, B.-y. Su, X.-h. Song, Q.-a. Chen, X. Chen and X.-r. Wang, TrAC, Trends Anal. Chem., 2011, 30, 665–676 CrossRef CAS.
- P. Rahimi and Y. Joseph, TrAC, Trends Anal. Chem., 2019, 110, 367–374 CrossRef CAS.
- J. Wu, X. Wang, Q. Wang, Z. Lou, S. Li, Y. Zhu, L. Qin and H. Wei, Chem. Soc. Rev., 2019, 48, 1004–1076 RSC.
- M. Liang and X. Yan, Acc. Chem. Res., 2019, 52, 2190–2200 CrossRef CAS PubMed.
- Y. Li and J. Liu, Mater. Horiz., 2021, 8, 336–350 RSC.
- Q. Chen, S. Li, Y. Liu, X. Zhang, Y. Tang, H. Chai and Y. Huang, Sens. Actuators, B, 2020, 305, 127511 CrossRef CAS.
- X. Zhang, X. Mao, S. Li, W. Dong and Y. Huang, Sens. Actuators, B, 2018, 258, 80–87 CrossRef CAS.
- B. Liu and J. Liu, Nano Res., 2017, 10, 1125–1148 CrossRef CAS.
- S. Li, Y. Hou, Q. Chen, X. Zhang, H. Cao and Y. Huang, ACS Appl. Mater. Interfaces, 2020, 12, 2581–2590 CrossRef CAS PubMed.
- H. Sun, A. Zhao, N. Gao, K. Li, J. Ren and X. Qu, Angew. Chem., Int. Ed., 2015, 54, 7176–7180 CrossRef CAS PubMed.
- A. H. Valekar, B. S. Batule, M. I. Kim, K.-H. Cho, D.-Y. Hong, U.-H. Lee, J.-S. Chang, H. G. Park and Y. K. Hwang, Biosens. Bioelectron., 2018, 100, 161–168 CrossRef CAS PubMed.
- W. Xu, Y. Kang, L. Jiao, Y. Wu, H. Yan, J. Li, W. Gu, W. Song and C. Zhu, Nano-Micro Lett., 2020, 12, 184 CrossRef CAS PubMed.
- J. Wu, Z. Wang, X. Jin, S. Zhang, T. Li, Y. Zhang, H. Xing, Y. Yu, H. Zhang, X. Gao and H. Wei, Adv. Mater., 2021, 33, 2005024 CrossRef CAS PubMed.
- W. Dong, G. Chen, X. Hu, X. Zhang, W. Shi and Z. Fu, Sens. Actuators, B, 2020, 305, 127530 CrossRef CAS.
- S. Tamagaki, K. Suzuki and W. Tagaki, Bull. Chem. Soc. Jpn., 1989, 62, 148–152 CrossRef CAS.
- W. Li, Y. Wang and A. Irini, Chem. Eng. J., 2014, 244, 1–8 CrossRef CAS.
- C. Iuga, J. R. Alvarez-Idaboy and A. Vivier-Bunge, J. Phys. Chem. B, 2011, 115, 12234–12246 CrossRef CAS PubMed.
- X. Xu, X. Zou, S. Wu, L. Wang, X. Niu, X. Li, J. Pan, H. Zhao and M. Lan, Anal. Chim. Acta, 2019, 1053, 89–97 CrossRef CAS PubMed.
- H. Guan, B. Liu, D. Gong, B. Peng, B. Han and N. Zhang, Microchem. J., 2021, 164, 105943 CrossRef CAS.
- A. Numan, M. M. Shahid, F. S. Omar, K. Ramesh and S. Ramesh, Sens. Actuators, B, 2017, 238, 1043–1051 CrossRef CAS.
- C. Zhao, Z. Jiang, R. Mu and Y. Li, Talanta, 2016, 159, 365–370 CrossRef CAS PubMed.
- M. I. Halawa, F. Wu, T. H. Fereja, B. Lou and G. Xu, Sens. Actuators, B, 2018, 254, 1017–1024 CrossRef CAS.
- M. Yin, S. Li, Y. Wan, L. Feng, X. Zhao, S. Zhang, S. Liu, P. Cao and H. Wang, Chem. Commun., 2019, 55, 12008–12011 RSC.
- Q. Chen, C. Liang, X. Zhang and Y. Huang, Talanta, 2018, 182, 476–483 CrossRef CAS PubMed.
- J. Liu, L. Meng, Z. Fei, P. J. Dyson and L. Zhang, Biosens. Bioelectron., 2018, 121, 159–165 CrossRef CAS PubMed.
- J. Zhu, X. Peng, W. Nie, Y. Wang, J. Gao, W. Wen, J. N. Selvaraj, X. Zhang and S. Wang, Biosens. Bioelectron., 2019, 141, 111450 CrossRef CAS PubMed.
- M. N. Ivanova, E. D. Grayfer, E. E. Plotnikova, L. S. Kibis, G. Darabdhara, P. K. Boruah, M. R. Das and V. E. Fedorov, ACS Appl. Mater. Interfaces, 2019, 11, 22102–22112 CrossRef CAS PubMed.
- J. Wang, Y. Hu, Q. Zhou, L. Hu, W. Fu and Y. Wang, ACS Appl. Mater. Interfaces, 2019, 11, 44466–44473 CrossRef CAS PubMed.
- Y. He, N. Li, X. Liu, W. Chen, X. Zhu and Q. Liu, Microchim. Acta, 2021, 188, 171 CrossRef CAS PubMed.
- H. Liu, Y.-N. Ding, B. Bian, L. Li, R. Li, X. Zhang, Z. Liu, X. Zhang, G. Fan and Q. Liu, Microchim. Acta, 2019, 186, 755 CrossRef CAS PubMed.
|
This journal is © The Royal Society of Chemistry 2023 |
Click here to see how this site uses Cookies. View our privacy policy here.