DOI:
10.1039/D3RA05346F
(Paper)
RSC Adv., 2023,
13, 28729-28735
Porous acid–base hybrid polymers for enhanced NH3 uptake with assistance from cooperative hydrogen bonds†
Received
7th August 2023
, Accepted 15th September 2023
First published on 2nd October 2023
Abstract
Carboxylic acid-modified materials are a common means of achieving efficient NH3 adsorption. In this study, we report that improved NH3 adsorption capacity and easier desorption can be achieved through the introduction of substances containing Lewis basic groups into carboxylic acid-modified materials. Easily synthesized mesoporous acid–base hybrid polymers were constructed with polymers rich in carboxylic acid and Lewis base moieties through cooperative hydrogen bonding interactions (CHBs). The hybrid polymer PAA–P4VP presented higher NH3 capacity (18.2 mmol g−1 at 298 K and 1 bar NH3 pressure) than PAA (6.0 mmol g−1) through the acid–base reaction and the assistance from CHBs with NH3, while the NH3 desorption from PAA–P4VP was easier for the reformation of CHBs. Based on the introduction of CHBs, a series of mesoporous acid–base hybrid polymers was synthesized with NH3 adsorption capacity of 15.8–19.3 mmol g−1 and high selectivity of NH3 over CO2 (SNH3/CO2 = 25.4–56.3) and N2 (SNH3/N2 = 254–1068), and the possible co-existing gases, such as SO2, had a lower effect on NH3 uptake by hybrid polymers. Overall, the hybrid polymers present efficient NH3 adsorption owing to the abundant acidic moieties and CHBs, while the concomitant Lewis bases promote NH3 desorption.
Introduction
Ammonia (NH3) is indispensable in our lives today for producing artificial fertilizers and several military and commercial products, including explosives, refrigerants, pharmaceuticals, and synthetic fibers. NH3 is also a potential fuel, providing a way to store and transport hydrogen owing to its exploitable energy density. However, the unavoidable leakage of NH3 during its utilization has huge adverse impacts on the environment and human health. In these applications associated with the critical risks of this gas, effective adsorbents possessing the ability to store more NH3 have been attracting substantial attention.
Porous materials with high surface areas, such as active carbon1 and zeolites,2–4 have been used as NH3 adsorbents; however, they suffer from relatively low affinity and limited capacity for NH3. Simultaneously, porous materials, including metal–organic frameworks (MOFs),5,6 porous organic polymers (POPs),7 covalent organic frameworks (COFs),8 and hydrogen-bonded organic frameworks (HOFs),9 were developed as efficient NH3 adsorbents owing to their highly porous nature, strong stability, and designable abundant binding sites.10 The modification of porous materials with functionalized moieties was expected to result in superior adsorbed amounts. Functionalized UiO-66-A/B/C11 and UiO-66-ox synthesized via post-synthetic modification with free carboxylic acids indicated their positive utilization in NH3 capture,12 likewise the NH3 adsorption performance of Zr-based UiO-66 analogues.13,14 Various Brønsted acidic groups, such as –CO2H and –SO3H, were used to functionalize the water-stable framework UiO-66 and were reported with improved NH3 capacity.15 Acid-loaded porphyrin-based MOFs16 for capturing NH3 show remarkable stability and the isoreticular porphyrin-based MOFs17 were reported with rod-like secondary building units of Brønsted acid bridging hydroxyl groups for NH3 sorption. Similarly, the zirconium-based MOF, NU-300 with free Brønsted acid sites benefited the binding of NH3 even at low pressures.18 Recently, carboxylic-functionalized mesoporous copolymers PDVB-xAA were developed for fast, highly efficient, selective, and reversible NH3 adsorption.19 These studies revealed that NH3 capture relies on the interplay of the functional groups, especially the –COOH of adsorbents.
It was found that the COFs modified with weak acid groups and hydrogen bonding interactions presented more efficient NH3 uptake than strong acid-functionalized COFs. BBP-5, a COF with carboxylate acid group showed more efficient and reversible NH3 uptake than PPN-6-SO3H due to the existence of cooperative hydrogen bonds (CHBs) along with the acid-Lewis base interactions between carboxylic acid and NH3, which indicated that multiple chemical interactions were optimized to single strong interactions.20 Similarly, urea-functionalized Zn-MOFs with an increased number of hydrogen bonds21 were also reported to have outstanding NH3 adsorption. HOFs, mainly constructed by the self-assembly of organic molecules via intermolecular hydrogen bonding interactions, were explored as a potential corrosive gas trapping agent because of their abundant CHBs and porous structure. Jancik22 explored UNAM-1 constructed of hydrogen-bonded frameworks, which achieved the porosity required for reversible SO2 uptake. Kang9 reported a hydrogen-bonded network KUF-1 for NH3 capture with a sigmodal adsorption isotherm and achieved the NH3 capacity of 6.67 mmol g−1 at 1 bar. They found that the design of an adsorbent/absorbent with a flexible hydrogen bonding network presented superior NH3 capacity and desorption regeneration. However, the refined design and cost-intensive synthesis of MOFs, COFs, and HOFs are very real problems against their application.
It was found that the hydrogen bonding interactions between the ionic liquids (ILs) and NH3 were the key to the significant increase in NH3 capacity according to Palomar's work.23 Therefore, protic ILs24,25 and ILs substituted with hydroxyl groups,23,26 and poly ionic liquids (PILs) served as adsorbents of ammonia.27,28 In our previous work,29,30 amidine and pyridine-based protic ILs constructed with CHBs presented sigmodal isotherms, and the results indicated a high NH3 capacity of 7.5–9.3 mmol g−1 at 1 bar, 30 °C; furthermore, the threshold pressure could be regulated through the CHBs. Deep eutectic solvents (DESs), known as new ILs analogues, consist of hydrogen bond acceptors (HBA) and hydrogen bond donors (HBD) in suitable molar ratios based on hydrogen bonding interactions and other intermolecular noncovalent interactions.31–38 It has been proposed that DESs are very promising NH3 absorbents through Lewis acid–base and hydrogen bonding interactions.39 For instance, choline chloride-composed DESs were developed for NH3 absorbents,40–42 and choline chloride/resorcinol/glycerol (1
:
3
:
5) presented the NH3 absorption capacity of 13 wt% at 313.2 K and 0.1 MPa for the internal hydrogen-bonded network of DESs.40 Alcohol, phenol,43,44 and sugar45 were also selected as components of DESs by taking advantage of their hydroxyl groups for effective NH3 absorption. Protic DESs with NH4SCN, ethylamine hydrochloride and ethanolamine hydrochloride as HBAs were reported as excellent NH3 absorbents through strong hydrogen bonding interactions,46,47 and the influences of HBA of EDSs on the NH3 absorption performances were systematically investigated. According to the weak acidity of azole, azole-based DESs were also used as NH3 absorbents;48–50 it was found that the greater acidity of the azole benefited the NH3 capacity but went against the reversibility.51 Recently, it was reported that DESs involving metal chlorides including LiCl,52 M(II)Cl2,53,54 and M(III)Cl3,53 could achieve greater NH3 capacity for the coordination of NH3 with metal and hydrogen bonding interactions. For instance, it was found that the CHBs-rich ILs/DESs had a good trapping effect on NH3, while the NH3 absorption capacity would be enhanced by 18.1–36.9% when a small amount of metal chlorides was added to Res/EG (1
:
2) DES.54
The reports of sorbents substituted with carboxylic acid for efficient NH3 adsorption and the utilization of hydrogen bonds to improve NH3 desorption inspired us to construct self-assembled hybrid polymers based on the formation of CHBs between carboxylate acids and Lewis bases (Fig. 1a), which would enhance the affinity for NH3 as well as promote NH3 desorption by the reconstruction of CHBs. To obtain this target, various acid–base hybrid polymers (Fig. 1b) were explored to analyze the effects of the interactions between acidic and basic groups on NH3 uptake.
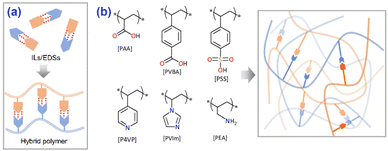 |
| Fig. 1 (a) A diagram showing the idea of developing acid–base hybrid polymers for NH3 uptake. (b) Structures of the agents used for synthesizing hybrid polymers. | |
Results and discussion
The reagents, preparation and characterization of hybrid polymers, and the NH3 adsorption and desorption experiments are described in the ESI File† in detail.
The characterization of hybrid polymers
To explore the feasibility of the utilization of CHBs in enhancing the NH3 uptake by acid–base hybrid polymers, PAA and PVBA with carboxylic acid groups were hybridized with P4VP/PVIm containing Lewis base groups to synthesize hybrid polymers as NH3 adsorbents. The CHBs were further proposed from the optimized structures calculated through the DFT method as shown in Fig. S2.† The interaction energies between these acid and basic moieties were between −40.23 and −46.90 kJ mol−1 as listed in Table 1, which allows the possibility to self-assemble the acid–base hybrid polymers. For PAA–P4VP, the interactions between PAA and P4VP were calculated through propionic acid and 4-ethylpyridine, the reaction enthalpy was −40.23 kJ mol−1 and the Gibbs free energy was −1.28 kJ mol−1, which indicate the possibility of interaction between PAA and P4VP.
Table 1 The interaction energies between acidic and basic groups and the NH3 desorbed active energy from the TPD test
Entry |
Complex |
Interaction energya (kJ mol−1) |
TDP peak (°C) |
Desorbed active energyb (kJ mol−1) |
The interaction energies between acidic and basic groups were obtained through DTF calculations on the B3LYP basis set at the 6-31G++ level. The active energies of NH3 desorption were calculated based on the Redhead method55 according to the desorption temperature from NH3-TPD. |
1 |
PAA–P4VP |
−40.23 |
78 |
104.0 |
2 |
PAA–PVIm |
−46.90 |
90 |
107.6 |
3 |
PVBA–P4VP |
−40.05 |
75 |
103.2 |
4 |
PVBA–PVIm |
−46.75 |
87 |
106.8 |
5 |
PAA |
— |
112 |
114.3 |
The elemental contents of C, N, and H listed in Table S1† correspond with the theoretical data calculated from the molar ratio of acid and base moieties of 1
:
1, indicating that the hybrid polymers self-assemble in the equimolar reaction of –COOH and Lewis base. The IR spectra of acid–base hybrid polymers in Fig. S3† exhibit the changes in the vibration of C
O as compared with acid polymers for the formation of CHBs. The IR spectra of PAA and PAA–P4VP in Fig. S3a† show that the stretching vibration of –COOH at 1698 cm−1 was blue shifted to 1713 cm−1 with the hybridization with P4VP (marked with a star). According to the 2D correlation IR spectra in Fig. S4,† the absorption of ν(COOH) was correlated with the vibration of the pyridine group at about 1600 cm−1 (marked with a red star), which indicates the interaction between PAA and P4VP.
The SEM images in Fig. 2a present the mesoporosity of these hybrid polymers. The BET surface areas of hybrid polymers according to N2 adsorption at 77 K in Fig. 2b and S5† range from 11.2 to 23.6 m2 g−1 (Table 2) and their pore size distribution is 2–30 nm, which explains the mesoporous structure of these hybrid polymers. On comparison of the SEM images and N2 adsorptions of nonporous PAA in Fig. S6b† and 2b, it was concluded that the porosity of PAA–P4VP was due to the multiple interactions between PAA and P4VP.
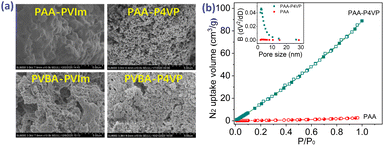 |
| Fig. 2 (a) The SEM images of hybrid polymers. (b) The N2 adsorption isotherm curve at 77 K and the pore distribution (inner picture) of PAA and PAA–P4VP. | |
Table 2 The BET surface areas and gas uptake capacities of hybrid polymers and PAA
Entry |
Complex |
SBETa (m2 g−1) |
Gas capacity (mmol g−1) |
Selectivityd |
NH3b |
N2c |
CO2c |
NH3/N2 |
NH3/CO2 |
The specific surface areas were calculated by the Brunauer–Emmett–Teller equation according to the N2 adsorption–desorption isotherm at 77 K. The NH3 uptake was operated under the self-made device at 25 °C. The N2 and CO2 adsorptions were detected via Micromeritics BAFLEX surface characterization measurements at 25 °C. The selectivity of NH3 over N2 (CO2) was calculated based on the capacity of NH3 divided by the capacity of N2 (CO2). |
1 |
PAA–P4VP |
23.6 |
18.2 |
0.0445 |
0.396 |
408 |
54.0 |
2 |
PAA–PVIm |
11.2 |
19.3 |
0.0181 |
0.398 |
1068 |
48.6 |
3 |
PVBA–P4VP |
23.0 |
18.4 |
0.0723 |
0.557 |
254 |
33.0 |
4 |
PVBA–PVIm |
23.6 |
15.8 |
0.0478 |
0.622 |
331 |
25.4 |
5 |
PAA |
0.7 |
6.0 |
— |
— |
— |
— |
NH3 uptake of PAA–P4VP vs. pure PAA
It was reported that materials rich in CHBs are suitable for efficient NH3 uptake; thus, hybrid polymers were used for NH3 uptake. Interestingly, PAA–P4VP presents a higher NH3 uptake capacity of 18.2 mmol g−1 than PAA (6.0 mmol g−1) from Fig. 3a, and the NH3 trapped in PAA–P4VP would be released feasibly. From the reported data according to the properties of DESs, ILs, and acid-modified MOFs listed in Table S2,† the hybrid polymer showed excellent NH3 uptake capacity and mild desorption conditions. The comparison of the IR spectra of fresh and NH3 saturated samples in Fig. 3b indicates that –COOH reacts with NH3 to form –COONH4 according to the vibration of –COO− at 1500 cm−1, and new peaks at 850 cm−1 (marked with #) ascribed to the hydrogen-bonded NH3.19 From the NH3-TPD detection curve in Fig. 3c, the most ammonia was released from PAA–P4VP when the temperature reached 78 °C, while the NH3 desorption peak occurred at 112 °C for PAA, indicating that the NH3 release from PAA–P4VP was easier. It should be noted the peaks at about 200 °C, which arise from polymer fragments, collapsed according to the TGA results as shown in Fig. S6;† this indicates that the weights of PAA and PAA–P4VP begin to decline from 207 and 198 °C, respectively. The comparison of the IR spectra of the fresh and recovered samples after NH3 desorption in Fig. 3b indicates that the PAA–P4VP could be recovered, and it is probably facilitated by the exothermic CHB formation between PAA and P4VP.29,56 The –COONH4 remains in PAA for the obvious absorption of COO− at 1540 cm−1 (marked with pink shadow), which demonstrates the better properties of PAA–P4VP as an NH3 adsorbent as compared to PAA. For 8 consecutive cycles of NH3 adsorption–desorption experiments (Fig. 3d), the efficient NH3 capacity of PAA–P4VP remained steady while there was an obvious decrease for PAA, which might be due to part of the unrecovered carboxylic acid in PAA. The uptake of NH3 and other gases by PAA–P4VP in Fig. S7† shows that there was not considerable CO2 or N2 adsorption, and the selectivity of NH3 as compared to CO2 and N2 was 54.0 and 408, respectively. As can be seen, the acid–base hybrid polymer PAA–P4VP presented enhanced NH3 adsorption and desorption as compared with PAA and high selectivity for NH3, which indicate the potential application of acid–base polymers as NH3 adsorbents.
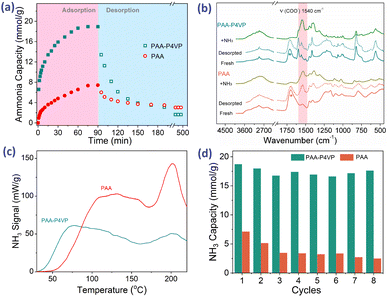 |
| Fig. 3 (a) The NH3 uptake and residual capacity of PAA and PAA–P4VP with time. (b) FT-IR spectra of fresh, NH3 saturated, and recovered PAA and PAA–P4VP. (c) NH3-TPD curves of PAA and PAA–P4VP with temperature increase ratio of 2 °C min−1 to 220 °C. (d) 8 consecutive NH3 adsorption–desorption results for PAA and PAA–P4VP. NH3 uptake at 25 °C, 1 bar. NH3 desorption at 80 °C under vacuum. | |
The effects of CHBs on the NH3 uptake of PAA–P4VP
To investigate the effects of P4VP on the NH3 uptake of PAA–P4VP, other complexes including PAA-BPY and PAA-PS were synthesized (Fig. S8a†). PS has the same chainlike structure like P4VP but without the Lewis base moieties, while BPY has the same Lewis base moieties as P4VP. The PAA-BPY and PAA-PS are non-porous as seen from the SEM in Fig. S8b,† which indicates that the Lewis base moieties and the chainlike structure are important for forming porous complexes. The FT-IR spectra in Fig. S9a† show a blue shift of ν(COOH) when PAA reacts with BPY but it was less affected by PS, indicating the formation of the CHBs between PAA and BPY. The NH3 uptake properties from Fig. S10† show that the adsorption capacity and desorption of PAA-PS are close to those of PAA, indicating that there was no obvious improvement in the NH3 uptake of PAA for the mixture of PS. The NH3 capacity of PAA-BPY was 13.0 mmol g−1 and just 1.5 mmol NH3 g−1 remained after desorption under vacuum at 80 °C for 90 min. The superior properties of PAA-BPY as compared to PAA-PS indicate that the insertion of Lewis base moieties benefits NH3 uptake capacity and desorption, and the competitive formation of CHBs between carboxylic acid and pyridine groups, which implies that the hybrid polymers are excellent ammonia sorbents.
NH3 uptake of acid–base hybrid polymers
Based on the strategy of developing acid–base hybrid polymers for improved NH3 uptake, hybrid acid–base polymers constructed with PAA and PVBA as acid polymers, P4VP and PVIm as Lewis base polymers were synthesized to investigate the effects of the interactions between acid and base moieties on NH3 uptake properties. These hybrid polymers were used for NH3 uptake at 1 bar and 25 °C; their NH3 capacity was 15.8–19.3 mmol g−1 from Fig. 4a and most of the fixed NH3 could be desorbed under vacuum at 80 °C for 100 min. Simultaneously, the N2 and CO2 adsorption by the hybrid polymers was also measured at 1 bar and 25 °C, which showed that tiny amounts of CO2 but non-considerable N2 could be adsorbed, as shown in Table 2. The selectivity of NH3 as compared to CO2 and N2 was 25.4–54.0 and 254–1068, respectively, which suggests the potential for the efficient separation of NH3 from these mixture gases. Besides, some interfering gases are inevitably present in industry, and the effects of the adsorbed CO2, SO2, and H2O on NH3 uptake were investigated. Fig. 4b shows that 18.44 mmol g−1 NH3 would be fixed after 0.77 mmol g−1 SO2 adsorption of PAA–P4VP, and the same phenomenon for CO2, which indicates no obvious effect of the acid gas on the NH3 uptake by PAA–P4VP. There was a considerable H2O uptake capacity of 7.22 mmol g−1 by PAA–P4VP but the subsequent ammonia adsorption capacity was reduced to 16.71 mmol g−1, likely due to the active hydrogen bonding sites being occupied by H2O.
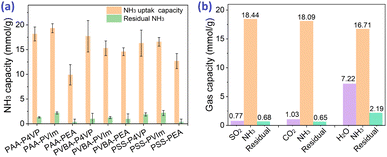 |
| Fig. 4 (a) NH3 uptake capacity of the acid-basic hybrid polymers at 25 °C and 1 bar. (b) Staged adsorption of 5% SO2 and NH3, CO2 and NH3, as well as 3.1% H2O and NH3 of PAA–P4VP at 25 °C. The residual gases were obtained after desorption in a vacuum at 80 °C for 100 min. The remaining capacity of adsorbate after desorption was converted into NH3 gas. | |
The capacity of acid-basic hybrid polymers is susceptible to ammonia pressure and temperature; from Fig. 5, the NH3 capacity decreases along with the increase in temperature and decrease in NH3 pressure, which indicates that the NH3 would be desorbed with the variation of temperature and pressure. It should be noted that there were uptake plateaus at P/P0 = 0.05 of PAA–P4VP and P/P0 = 0.2 of PAA-PVIm and PVBA-PVIm, and their stepwise uptake of approximately 6 mmol g−1 from Fig. 5a, corresponding to about 1 equivalent of NH3 per mol –COOH for the acid–base reaction to –COONH4. Another 10–13 mmol g−1 NH3 uptake of these hybrid polymers probably contributed to the hydrogen interaction.
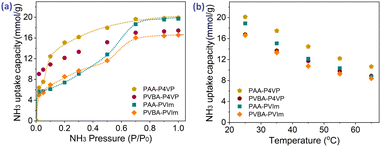 |
| Fig. 5 NH3 uptake capacity of acid-basic hybrid polymers at various temperatures under 1 bar NH3 pressure (a) and under various pressures at 25 °C (b). | |
The analysis of NH3 uptake of acid–base hybrid polymers
The IR spectra of the hybrid polymers compared with their NH3 saturated state are shown in Fig. 6. The increase in absorption at about 3400 cm−1 was due to the N–H stretching of adsorbed ammonia. Another characteristic peak at about 1470 cm−1 increased after ammonia absorption, which can be attributed to the symmetric deformation of the ammonium ion.57,58 Meanwhile, the disappearance of νs(COOH) at about 1710 cm−1 (marked with *) and the increase in νs(COO−) and νas(COO−) at about 1520 cm−1 and 1350 cm−1 (marked with #) indicate the carboxylic acid of hybrid polymers forming the carboxylate salt.59 These results support the formation of NH4+ from the reaction of NH3 with the proton of hybrid polymers.60 A new peak at about 910 cm−1 (marked with ▼) is ascribed to the hydrogen bonding of NH3 from PAA-PVIm and PVBA-PVIm, while that at 850 cm−1 was due to PVBA–P4VP.19 The results claim the hybrid polymers from carboxylic acid polymers and Lewis base polymers achieved high NH3 uptake capacity, which is attributed to the cooperative acid–base interaction and hydrogen bonding interactions.
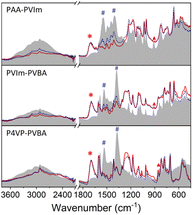 |
| Fig. 6 Comparison of the partial IR spectra of hybrid polymers (red solid line) with their NH3 saturated (gray background) and desorbed states (blue dotted lines). | |
The properties of NH3 desorption from the hybrid acid–base polymers were investigated through NH3-TPD measurements. The results presented in Fig. S11† indicate NH3 desorption peak before 100 °C for all these hybrid polymers, the active energy of desorption of 103.2–107.6 kJ mol−1 as listed in Table 1, which belonged to the NH3 released from the NH3 fixed by acid–base interactions. The ammonia trapped by PVBA–P4VP and PVBA-PVIm would be released completely within 200 °C, indicating the feasible desorption of NH3 from these hybrid polymers. The IR spectra of the hybrid polymers after the NH3-TPD test compared with the pristine sample in Fig. 6 indicate that carboxylic acid would be recovered after NH3 desorption. The reusability of these hybrid polymers for NH3 uptake was further investigated. Fig. S12–14† show that the NH3 uptake capacity did not decrease within 6 cycles of consecutive NH3 uptake and desorption. About 2.8 mmol g−1 NH3 remained in PAA-PVIm (2.3 mmol g−1 NH3 remained in PVBA-PVIm) after desorption, which would not affect the subsequent NH3 adsorption. The reversibility of these hybrid polymers indicates that the acidic polymer hybrid with appropriate basic polymer is one of the designable strategies to promote NH3 desorption and the recovery of hybrid polymers.
Conclusions
The simple acid–base hybrid polymer PAA–P4VP presents a porous structure and superior NH3 uptake properties as compared to PAA for synergetic NH3 capture through acid–base and CHB interactions. Inspired by this result, a series of mesoporous acid–base hybrid polymers presenting surface areas of 11.2 to 23.6 m2 g−1 were synthesized according to the predicted interactions between the acidic and basic moieties. These acid–base hybrid polymers were used for efficient NH3 uptake with the capacity of 15.8–19.3 mmol NH3 g−1 through the cooperative acid–base reaction and hydrogen bonding interactions. The trapped NH3 can be released at 80 °C under vacuum along with the reformation of CHBs between the –COOH and Lewis base moieties. This indicates that the incorporation of the basic moiety is a feasible method for improving the NH3 uptake and desorption of materials with the carboxylic acid groups.
Author contributions
Conceptualization, Xiaoyan Luo and Congmin Wang; data curation, Yibang Liu; formal analysis, Xiaoyan Luo and Yibang Liu; funding acquisition, Xiaoyan Luo; investigation, Xiaoyan Luo and Yibang Liu; methodology, Yibang Liu and Mingxing Li; project administration, Congmin Wang; resources, Ling Ye, Xuegong Cao and Congmin Wang; software, Xuegong Cao; supervision, Xiaoyan Luo and Congmin Wang; validation, Yibang Liu, Mingxing Li and Renhui Ling; writing – original draft, Xiaoyan Luo; writing – review & editing, Congmin Wang.
Conflicts of interest
There are no conflicts to declare.
Acknowledgements
This work was funded by the National Natural Science Foundation of China (21803021, 22278165), Science and Technology Innovation Funding Project from Huaqiao University (ZQN-PY605). We acknowledge the instrumental analysis center of Huaqiao University.
References
- D. Barpaga and M. D. LeVan, Microporous Mesoporous Mater., 2016, 221, 197–203 CrossRef CAS.
- M. R. Adam, M. H. D. Othman, S. H. S. A. Kadir, M. N. M. Sokri, Z. S. Tai, Y. Iwamoto, M. Tanemura, S. Honda, M. H. Puteh, M. A. Rahman and J. Jaafar, Membranes, 2020, 10, 63 CrossRef CAS PubMed.
- P. Assawasaengrat and R. Rueangdechawiwat, IOP Conf. Ser.: Mater. Sci. Eng., 2019, 639, 012050 CAS.
- I. Matito-Martos, A. Martin-Calvo, C. O. Ania, J. B. Parra, J. M. Vicent-Luna and S. Calero, Chem. Eng. J., 2020, 387, 124062 CrossRef CAS.
- A. Gładysiak, Tu N. Nguyen, J. A. R. Navarro, M. J. Rosseinsky and K. C. Stylianou, Chem. – Eur. J., 2017, 23, 13602–13606 CrossRef PubMed.
- A. J. Rieth and M. Dinca, J. Am. Chem. Soc., 2018, 140, 3461–3466 CrossRef CAS PubMed.
- D. W. Kang, M. Kang, M. Moon, H. Kim, S. Eom, J. H. Choe, W. R. Lee and C. S. Hong, Chem. Sci., 2018, 9, 6871–6877 RSC.
- C. J. Doonan, D. J. Tranchemontagne, T. G. Glover, J. R. Hunt and O. M. Yaghi, Nat. Chem., 2010, 2, 235–238 CrossRef CAS PubMed.
- D. W. Kang, M. Kang, H. Kim, J. H. Choe, D. W. Kim, J. R. Park, W. R. Lee, D. Moon and C. S. Hong, Angew. Chem., Int. Ed., 2019, 58, 16152–16155 CrossRef CAS PubMed.
- D. W. Kang, S. E. Ju, D. W. Kim, M. Kang, H. Kim and C. S. Hong, Adv. Sci., 2020, 7, 2002142 CrossRef CAS PubMed.
- W. Morris, C. J. Doonan and O. M. Yaghi, Inorg. Chem., 2011, 50, 6853–6855 CrossRef CAS PubMed.
- J. B. DeCoste, T. J. Demasky, M. J. Katz, O. K. Farha and J. T. Hupp, New J. Chem., 2015, 39, 2396–2399 RSC.
- H. Jasuja, G. W. Peterson, J. B. Decoste, M. A. Browe and K. S. Walton, Chem. Eng. Sci., 2015, 124, 118–124 CrossRef CAS.
- T. Yoskamtorn, P. Zhao, X. P. Wu, K. Purchase, F. Orlandi, P. Manuel, J. Taylor, Y. Y. Li, S. Day, L. Ye, C. C. Tang, Y. F. Zhao and S. C. E. Tsang, J. Am. Chem. Soc., 2021, 143, 3205–3218 CrossRef CAS PubMed.
- G. Barin, G. W. Peterson, V. Crocella, J. Xu, K. A. Colwell, A. Nandy, J. A. Reimer, S. Bordiga and J. R. Long, Chem. Sci., 2017, 8, 4399–4409 RSC.
- O. T. Wilcox, A. Fateeva, A. P. Katsoulidis, M. W. Smith, C. A. Stone and M. J. Rosseinsky, Chem. Commun., 2015, 51, 14989–14991 RSC.
- S. Moribe, Z. J. Chen, S. Alayoglu, Z. H. Syed, T. Islamoglu and O. K. Farha, ACS Mater. Lett., 2019, 1, 476–480 CrossRef CAS.
- Y. W. Chen, X. Zhang, K. K. Ma, Z. J. Chen, X. J. Wang, J. Knapp, S. Alayoglu, F. F. Wang, Q. B. Xia, Z. Li, T. Islamoglu and O. K. Farha, ACS Appl. Nano Mater., 2019, 2, 6098–6102 CrossRef CAS.
- J. Zhang, M. Yongde, W. Wu, Z. Cai, Y. Cao, K. Huang and L. Jiang, Chem. Eng. J., 2022, 448, 137640 CrossRef CAS.
- J. F. Van Humbeck, T. M. McDonald, X. Jing, B. M. Wiers, G. Zhu and J. R. Long, J. Am. Chem. Soc., 2014, 136, 2432–2440 CrossRef CAS PubMed.
- S. Glomb, D. Woschko, G. Makhloufi and C. Janiak, ACS Appl. Mater. Interfaces, 2017, 9, 37419–37434 CrossRef CAS PubMed.
- I. R.-L. Ricardo Dominguez-Gonzalez, E. Martinez-Ahumada, D. Martinez-Otero, H. A. Lara-Garcia, J. Balmaseda-Era, I. A. Ibarra, E. G. Percastegui and V. Jancik, J. Mater. Chem. A, 2019, 6, 26812 RSC.
- J. Palomar, M. Gonzalez-Miquel, J. Bedia, F. Rodriguez and J. J. Rodriguez, Sep. Purif. Technol., 2011, 82, 43–52 CrossRef CAS.
- D. S. Deng, X. X. Deng, K. Li and H. Fang, Sep. Purif. Technol., 2021, 276, 119298 CrossRef CAS.
- D. W. Shang, X. P. Zhang, S. J. Zeng, K. Jiang, H. S. Gao, H. F. Dong, Q. Y. Yang and S. J. Zhang, Green Chem., 2017, 19, 937–945 RSC.
- L. Yuan, X. P. Zhang, B. Z. Ren, Y. L. Yang, Y. G. Bai, L. Bai, H. S. Gao and S. J. Zeng, J. Chem. Technol. Biotechnol., 2020, 95, 1815–1824 CrossRef CAS.
- L. Xia, Q. Cui, X. Suo, Y. Li, X. Cui, Q. Yang, J. Xu, Y. Yang and H. Xing, Adv. Funct. Mater., 2018, 28, 1704292 CrossRef.
- X. Suo, X. Cui, L. Yang, N. Xu, Y. Huang, Y. He, S. Dai and H. Xing, Adv. Mater., 2020, 32, 1907601 CrossRef CAS PubMed.
- X. Y. Luo, R. X. Qiu, X. Y. Chen, B. Y. Pei, J. Q. Lin and C. M. Wang, ACS Sustainable Chem. Eng., 2019, 7, 9888–9895 CrossRef CAS.
- R. X. Qiu, X. Y. Luo, L. Yang, J. R. Li, X. Y. Chen, C. Peng and J. Q. Lin, ACS Sustainable Chem. Eng., 2020, 8, 1637–1643 CrossRef CAS.
- Y. Marcus, The Variety of Deep Eutectic Solvents, in Deep eutectic solvents, Springer, Cham, 2019, pp. 13–44, DOI:10.1007/978-3-030-00608-2_2.
- D. Yu and T. Mu, J. Phys. Chem. B, 2019, 123, 4958–4966 CrossRef CAS PubMed.
- Q. Zhang, K. De Oliveira Vigier, S. Royer and F. Jerome, Chem. Soc. Rev., 2012, 41, 7108–7146 RSC.
- A. I. Akhmetshina, A. N. Petukhov, A. Mechergui, A. V. Vorotyntsev, A. V. Nyuchev, A. A. Moskvichev and I. V. Vorotyntsev, J. Chem. Eng. Data, 2018, 63, 1896–1904 CrossRef CAS.
- F. Zhong, H. Peng, D. Tao, P. Wu, J. Fan and K. Huang, ACS Sustain. Chem. Eng., 2019, 7, 3258–3266 CrossRef.
- D. Deng, B. Gao, C. Zhang, X. Duan, Y. Cui and J. Ning, Chem. Eng. J., 2019, 358, 936–943 CrossRef CAS.
- Z.-L. Li, F.-Y. Zhong, L.-S. Zhou, Z.-Q. Tian and K. Huang, Ind. Eng. Chem. Res., 2020, 59, 2060–2067 CrossRef CAS.
- D. S. Deng, X. X. Deng, X. Z. Duan and L. Gong, J. Mol. Liq., 2021, 324, 114719 CrossRef CAS.
- W. Sun, T. Li, H. Chu, J. Liu, K. Zhong and L. Feng, J. Cleaner Prod., 2022, 373, 133764 CrossRef CAS.
- Y. H. Li, M. C. Ali, Q. W. Yang, Z. G. Zhang, Z. B. Bao, B. G. Su, H. B. Xing and Q. L. Ren, ChemSusChem, 2017, 10, 3368–3377 CrossRef CAS PubMed.
- X. Z. Duan, B. Gao, C. Zhang and D. S. Deng, J. Chem. Thermodyn., 2019, 133, 79–84 CrossRef CAS.
- F. Y. Zhong, K. Huang and H. L. Peng, J. Chem. Thermodyn., 2019, 129, 5–11 CrossRef CAS.
- F. Y. Zhong, H. L. Peng, D. J. Tao, P. K. Wu, J. P. Fan and K. Huang, ACS Sustainable Chem. Eng., 2019, 7, 3258–3266 CrossRef.
- N. N. Cheng, Z. L. Li, H. C. Lan, W. L. Xu, W. J. Jiang, K. Huang and H. L. Peng, Sep. Purif. Technol., 2021, 269, 118791 CrossRef CAS.
- Z. L. Li, F. Y. Zhong, J. Y. Huang, H. L. Peng and K. Huang, J. Mol. Liq., 2020, 317, 113992 CrossRef CAS.
- D. S. Deng, B. Gao, C. Zhang, X. Z. Duan, Y. H. Cui and J. H. Ning, Chem. Eng. J., 2019, 358, 936–943 CrossRef CAS.
- K. Li, H. Fang, X. Z. Duan and D. S. Deng, J. Mol. Liq., 2021, 339, 116724 CrossRef CAS.
- D. S. Deng, X. Z. Duan, B. Gao, C. Zhang, X. X. Deng and L. Gong, New J. Chem., 2019, 43, 11636–11642 RSC.
- F. Y. Zhong, L. S. Zhou, J. Shen, Y. Liu, J. P. Fan and K. Huang, ACS Sustainable Chem. Eng., 2019, 7, 14170–14171 CrossRef CAS.
- W. J. Jiang, J. B. Zhang, Y. T. Zou, H. L. Peng and K. Huang, ACS Sustainable Chem. Eng., 2020, 8, 13408–13417 CrossRef CAS.
- Z. L. Li, F. Y. Zhong, L. S. Zhou, Z. Q. Tian and K. Huang, Ind. Eng. Chem. Res., 2020, 59, 2060–2067 CrossRef CAS.
- K. Li, K. Zong, Z. Y. Zhou and D. S. Deng, Sep. Purif. Technol., 2021, 279, 119763 CrossRef CAS.
- N. N. Cheng, Z. L. Li, H. C. Lan, W. L. Xu and K. Huang, AIChE J., 2022, 68, e17660 CrossRef CAS.
- X. X. Sun, Q. H. Wang, S. H. Wu, X. Y. Zhao, L. G. Wei, K. L. Li, J. A. Hao, L. Wei, S. R. Zhai and Q. D. An, Int. J. Hydrogen Energy, 2022, 47, 16121–16131 CrossRef CAS.
- P. A. Redhead, Vacuum, 1962, 12, 203–211 CrossRef CAS.
- B. E. R. Snyder, A. B. Turkiewicz, H. Furukawa, M. V. Paley, E. O. Velasquez, M. N. Dods and J. R. Long, Nature, 2023, 613, 287–291 CrossRef CAS PubMed.
- D. W. Kim, D. W. Kang, M. Kang, D. S. Choi, H. Yun, S. Y. Kim, S. M. Lee, J.-H. Lee and C. S. Hong, J. Am. Chem. Soc., 2022, 144, 9672–9683 CrossRef CAS PubMed.
- C. Petit, B. Mendoza and T. J. Bandosz, Langmuir, 2010, 26, 15302–15309 CrossRef CAS PubMed.
- X. Kan, Z. Liu, F. Liu, F. Li, W. Chen, X. Yi, A. Zheng, L. Jiang and F.-S. Xiao, Chem. Eng. J., 2023, 451, 139085 CrossRef CAS.
- D. Jung, Z. Chen, S. Alayoglu, M. R. Mian, T. A. Goetjen, K. B. Idrees, K. O. Kirlikovali, T. Islamoglu and O. K. Farha, ACS Appl. Mater. Interfaces, 2021, 13, 10409–10415 CrossRef CAS PubMed.
|
This journal is © The Royal Society of Chemistry 2023 |
Click here to see how this site uses Cookies. View our privacy policy here.