DOI:
10.1039/D3SC01346D
(Edge Article)
Chem. Sci., 2023,
14, 7215-7220
A translationally active ligand based on a [2]rotaxane molecular shuttle with a 2,2′-bipyridyl core†
Received
13th March 2023
, Accepted 4th June 2023
First published on 13th June 2023
Abstract
A rigid H-shaped, [2]rotaxane molecular shuttle comprised of an axle containing two benzimidazole recognition sites and a central 2,2′-bipyridyl (bipy) group interlocked with a 24-crown-8 (24C8) wheel was synthesized using a threading followed by stoppering protocol. The central bipy chelating unit was shown to act as a speed bump that raised the barrier to shuttling for the [2]rotaxane. Coordination of a PtCl2 moiety to the bipy unit in a square planar geometry created an insurmountable steric barrier to shuttling. Addition of one equivalent of NaB(3,5-(CF3)2C6H3)4 removed one of the chloride ligands allowing for translation of the crown ether along the axle into the coordination sphere of the Pt(II) centre but full shuttling of the crown ether could not be activated. In contrast, addition of Zn(II) ions in a coordinating solvent (DMF) allowed shuttling to occur using a ligand exchange mechanism. DFT calculations showed this likely occurs via coordination of the 24C8 macrocycle to the Zn(II) centre bound to the bipy chelate. This interplay of the rotaxane axle and wheel components is an example of a translationally active ligand that utilises the large amplitude displacement of a macrocycle along an axle in a molecular shuttle to access ligand coordination modes not possible with conventional ligand designs.
Introduction
The design of purpose-built multidentate ligands has extensive history in the areas of catalysis,1–5 ion sequestration6–9 and bioinorganic chemistry.10–12 Rotaxanes which involve separate axle and macrocyclic wheel components held together by a mechanical bond offer a way to bring disparate donor groups together that might not be possible, or more difficult to accomplish with a single component molecule.13,14 Although metal ions have been utilised to template rotaxane formation15 – both passively and actively – and used to induce switching between co-conformations,16–19 exploration of the mechanical bond as a fundamental feature of ligand design is limited.13,20 In particular, the rotational and translational dynamics of rotaxanes are features not available with single component designs and their application in transition metal chemistry is rare.15,21
We recently explored the idea of using the rotational motion of a macrocycle about a rigid chelating axle to demonstrate how different binding modes of the rotaxane ligand can be “dialled-up” by rotating the macrocycle and how this affects metal ion binding and selectivity.22,23 Herein, we look at the concept of including large amplitude translation of a macrocycle along a rigid chelating axle into a ligand and investigate how this might affect the metal ion coordination and shuttling dynamics of such a system. The basic design components employed herein, an axle with a bipyridine chelate and a crown ether macrocycle, as well as the resulting interlocked [2]rotaxane ligand are shown in Fig. 1. We were interested in shedding light on the following questions: (i) how would the presence of the bipyridine N-atoms affect shuttling of the macrocycle in the free ligand, (ii) could the crown ether be used as a weak donor to a metal ion bound to the bipy chelate and (iii) was it possible for the macrocyclic wheel unit to undergo shuttling in the presence of a coordinated metal ion, perhaps by utilizing coordination to the metal and concomitant ligand exchange?
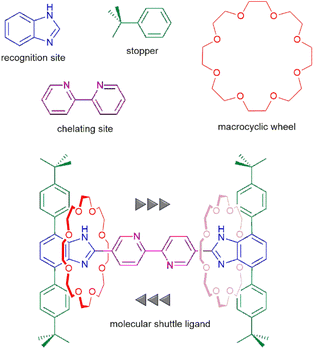 |
| Fig. 1 Design of a translationally active ligand based on a [2]rotaxane molecular shuttle showing the individual components and assembled ligand. | |
Results and discussion
Synthesis, and characterization of the [2]rotaxane ligand
The [2]rotaxane molecular shuttle ligand 4 was prepared as described in Scheme 1. Initially, diamine 1 was condensed with an excess of bipyridine-dialdehyde 2 to yield T-shaped benzimidazole 3 in 68% yield. Protonation of 3 with HBF4 to give [3-H]BF4 provided a benzimidazolium recognition site that, when combined with 24C8 in CHCl3 yielded the [2]pseudorotaxane [3-H⊂24C8]BF4. This was followed by a further condensation between the remaining aldehyde group of [3-H⊂24C8]BF4 and diamine 1 to give [2]rotaxane 4 in 67% yield. 4 was fully characterised by 1H and 13C NMR spectroscopy as well as HR-ESI MS and single crystal X-ray diffraction. Solution data are available in the ESI (see Fig. S8–S10†) and the solid-state structure of 4 is shown in Fig. 2.
 |
| Scheme 1 Synthesis of [2]rotaxane molecular shuttle ligand 4. Labels for 1H NMR peak assignments are shown for 4. | |
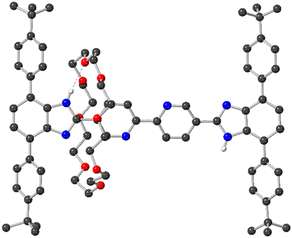 |
| Fig. 2 Single-crystal X-ray structure of [2]rotaxane ligand 4. Colour key: C dark grey, N blue, H white; covalent bonds grey, H-bonds dashed. All H atoms expect those bonded to N atoms were omitted for clarity. | |
The X-ray structure of 4 shows that the crown ether prefers to reside at one of the benzimidazole recognition sites where it is held in place by hydrogen bonding between one of the axle benzimidazole NH groups and crown ether O-atoms. The overall structure is very similar to a related neutral rotaxane in which the central bipyridine unit is a single phenyl group.24 Importantly, it is clear from the structure that the benzimidazole recognition site and accompanying crown ether do not block access to the chelating bipyridine group.
Shuttling of the [2]rotaxane ligand
The 1H NMR spectrum of 4 (see ESI, Fig. S8†) shows a single set of averaged resonances for complexed and uncomplexed axle protons indicating that the 24C8 wheel is shuttling between the two benzimidazole recognition sites at a rate that is faster than the NMR timescale. Variable temperature 1H NMR spectra in CD2Cl2 (see ESI, Fig. S9†) were recorded for 4 and used to determine the rate of translational motion. The observed shuttling rate of 61.2 s−1 (ΔG‡ = 15.1 kcal mol−1) is significantly slower than that observed for a similar system with a biphenyl unit and dibenzo[24]crown-8,24 6.2 × 103 s−1, (ΔG‡ = 9.0 kcal mol−1) indicating that the presence of the bipyridine group on the axle acts as a speed bump for shuttling. This was attributed to the electronic repulsion between the bipy N-atoms and crown ether O-atoms which significantly raises the barrier to translational motion of the wheel along the axle.
Coordination of rotaxane to Pt(II)
Initially, the substitutionally inert PtCl2 fragment was chosen for chelation to the bipy site of the axle as square planar complexes of the type [PtCl2(bipy)] are well known. In addition, it was reasoned that this would be a good base-line structure as it was very unlikely to involve coordination of the crown ether wheel component of the rotaxane. This was accomplished by reacting [PtCl2(DMSO)2] and 4 in CH3CN/CHCl3 (10/1) at 100 °C for 16 h. The solvent was removed from the resulting yellow solution, and the precipitate washed with a basic solution of acetonitrile to yield [PtCl2(4)] in 55% isolated yield.
Fig. 3a shows the 1H NMR spectrum of [PtCl2(4)]. Coordination of PtCl2 to the central bipy chelate site results in desymmetrisation of the [2]rotaxane such that separate sets of resonances are observed for the two different halves of the molecule i.e., with and without crown ether present. Thus, the PtCl2 fragment acts as a steric barrier to translation of the crown ether. The use of metal ion coordination to arrest wheel translation of a molecular shuttle has been accomplished previously but involved bringing axles from two separate rotaxanes together to bind a metal ion and could only be reversed by removal of the metal ion using exchange resin.25 In order to investigate whether the crown ether could participate in binding to the Pt(II) centre, [PtCl2(4)] was reacted with one equivalent of Na[BArF] (BArF = B(3,5-(CF3)2C6H3)4) in CH2Cl2. Fig. 3b shows the 1H NMR spectrum of the complex [PtCl(4)][BArF]. Changes in the spectrum infer removal of a chloride ion and coordination of the 24C8 wheel to the Pt(II) centre (see ESI, Fig. S18†).
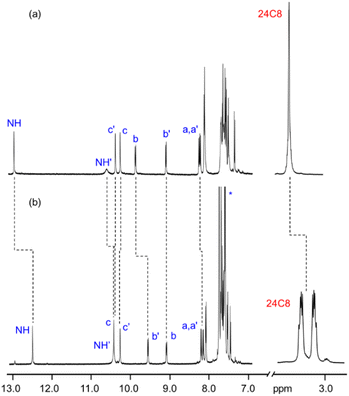 |
| Fig. 3
1H NMR spectra of (a) [PtCl2(4)] and (b) [PtCl(4)][BArF] in CD2Cl2. See Scheme 1 for labels, H-atoms labelled prime (e.g., a′) are from parts of the axle not interacting with 24C8. * indicates peaks due to the BArF anion. | |
Attempts to remove the remaining chloride ion using a further equivalent of Na[BArF] were unsuccessful (see ESI, Fig. S19†). This can be attributed to the fact that the 24C8 macrocycle is not capable of occupying two coordination sites at the square planar Pt(II) centre while it is restricted to an orientation perpendicular to the axle on account of its participation in the mechanical bond.
To further investigate the degree to which the crown ether could coordinate to the Pt(II) centre of [PtCl2(4)], a sample was dissolved in DMSO-d6 and evidence of exchange between the two benzimidazole resonances probed by EXSY (EXchange SpectroscopY) NMR experiments. It was hypothesised that it might be possible for the 24C8 wheel to shuttle from one recognition site to the other using a series of steps involving chloride ion, solvent exchange26 and coordination of the 24C8 macrocycle to the Pt centre. However, no evidence of wheel exchange between the recognition sites was observed even at increased temperatures (see ESI, Fig. S22†). The lack of shuttling was attributed to the awkward combination of a square planar coordination environment parallel to the axle and the restricted orientation of the macrocyclic due to its participation in the mechanical bond.
Coordination of rotaxane to Zn(II)
Since the relative orientation of the square planar geometry dictated by the bipy chelate and the track of the molecular shuttle were not conducive to ligand exchange assisted shuttling, it was reasoned that employing a metal centre without this geometric restriction might alleviate the problem.27 To test this hypothesis, one equivalent of ZnCl2 was added to a solution of 4 in CH2Cl2 at room temperature similar to the approach taken with coordination to Pt(II). Unfortunately, the 1H NMR spectrum of the mixture showed that the resulting Zn(II) complex had been converted to the mono-protonated species in which the crown ether binds solely to the newly created benzimidazolium group (see ESI, Fig. S23†). Such ease of protonation of basic groups when encircled by a crown ether as part of a rotaxane is well known28–31 and is probably promoted by adventitious water in the presence of Lewis acidic Zn(II) ions.32,33 Attempts to deprotonate the complex with moderately strong bases such as proton sponge were unsuccessful, and the use of stronger bases such as hydroxide ion resulted in decomposition of the complex.
After further testing numerous solvents and counterions, it was discovered that the addition of [Zn(NTf2)2] to 4 in DMF produced a neutral Zn(II) complex formulated as [Zn(DMF)4(4)][NTf2]2. The 1H NMR spectra of 4 and the Zn(II) complex are shown in Fig. 4. Similar to the Pt(II) complexes, the 1H NMR spectrum of [Zn(DMF)4(4)][NTf2]2 indicates that the 24C8 macrocycle resides at one of the benzimidazole recognition sites resulting in the observation of complexed and uncomplexed environments for each axle H-atom.
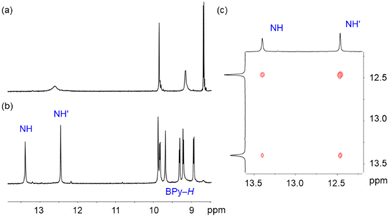 |
| Fig. 4
1H NMR spectra in DMF-d7 of (a) 4, (b) [Zn(DMF)4(4)][NTf2]2 and (c) partial EXSY spectrum showing exchanging NH peaks of [Zn(DMF)4(4)][NTf2]2. | |
Interestingly, in contrast to [PtCl2(4)] in DMSO which showed no evidence of shuttling, the EXSY spectrum (see Fig. 4c and ESI Fig. S33†) of [Zn(DMF)4(4)][NTf2]2 in DMF-d7 exhibited cross peaks indicative of benzimidazole NH exchange and molecular shuttling. Scheme 2 outlines the presumed ligand–solvent–24C8 exchange that occurs to allow the required translational shuttling motion. The key difference is that coordination to Zn(II) allows for an intermediate geometry where the crown ether can bind easily to the Zn(II) ions as it transits from one end of the axle to the other. These measurements produced a shuttling rate of 2.2 s−1 (ΔG‡ = 17.0 kcal mol−1) which is slower than that for the free ligand 4, presumably due to the Zn(II) coordination. Since the rate is dependent upon solvent exchange, it would be reasonable to assume that the rate of this type of shuttling would be dependent upon the solvent used. Unfortunately, this could not be verified with this system as only the DMF solutions studied were amenable to these measurements.
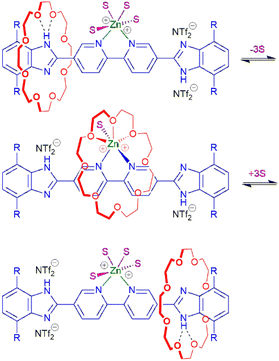 |
| Scheme 2 Proposed mechanism to promote shuttling of rotaxane wheel via ligand and solvent (S) exchange for [Zn(S)4(4)][NTf2]2. | |
DFT calculations were used to provide insight into the interaction between the Zn(II) centre and the rotaxane during the shuttling event. Fig. 5 shows a DFT calculated structure (see ESI† for details) of the exchange intermediate in which three of the crown ether oxygen atoms, the bipyridine chelate and a molecule of DMF are coordinated to the Zn(II) centre in an octahedral geometry. We have previously observed the coordination of three consecutive O-atoms of the macrocycle in this fashion when investigating the coordination of Ag(I) ions to a rotationally active rotaxane containing a bis(benzimidazole) chelate and a 24-membered crown ether.23
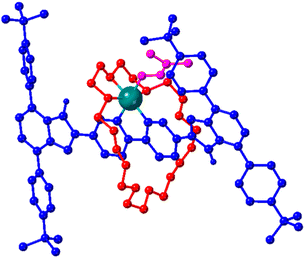 |
| Fig. 5 DFT calculated structure of proposed octahedral intermediate adopted during shuttling of 24C8 macrocycle of [Zn(DMF)4(4)][NTf2]2 in DMF solution; see Scheme 2 (centre). Axle blue, wheel red, DMF pink, Zn teal. All H atoms expect those bonded to imidazole N atoms were omitted for clarity. | |
Finally, slow evaporation of a 1
:
1 mixture of Zn(II) ions and rotaxane 4 afforded single crystals that allowed identification of an ML2 complex, [Zn(4)2(DMF)(H2O)][NTf2]2, in which two bipyridine ligands from two different rotaxane molecules were able to coordinate to a single Zn(II) centre in an octahedral geometry; the single crystal X-ray structure is shown in Fig. 6. It is interesting that two of these bulky rotaxanes are capable of coordinating to the same metal atom and it should be noted this arrangement, in principle, prevents shuttling of the crown ether component. Although it was not possible to directly observe this complex via1H NMR spectroscopy at varying M
:
L ratios or concentrations (see ESI, Fig. S31 and S32†), it is likely that this complex is present in solution with [Zn(DMF)4(4)][NTf2]2 at lower M
:
L ratios.
 |
| Fig. 6 Single-crystal-X-ray structure of the complex formed from a solution with a 1 : 1 Zn to 4 ratio; [Zn(4)2(DMF)(H2O)][NTf2]2. (a) Side view emphasizing the octahedral coordination geometry at Zn(II). (b) Top view showing the relative positions of the crown ether macrocycles on the rotaxane ligands. Rotaxanes are coloured blue axle with red wheel and green axle with orange wheel. Only the O-atoms of the coordinated DMF and water molecules are shown for clarity. Anions are not shown. All H-atoms are omitted for clarity. | |
Conclusions
We have reported the design and synthesis of a new molecular shuttle containing a chelating bipy unit. As previously observed, coordination of a metal centre to a chelate site on a molecular shuttle axle can block the translational motion26 and act as a brake to shuttling as was observed herein with Pt(II). However, it was discovered that the use of a metal centre such as Zn(II) allowed for the shuttling of a macrocycle along the axle to occur via a process involving wheel coordination and ligand exchange. This study is the first to: (i) delineate the concept of a translationally active ligand, (ii) demonstrate molecular shuttling aided by ligand coordination, and (iii) show that shuttling can be controlled (on or off) by tuning the coordination geometry – octahedral versus square planar – of a bound metal. More generally, the ability to transiently involve a weak donor (e.g., ether O-atom) from a mechanically bound macrocycle in the coordination environment of a reactive metal centre might be useful in catalysis and the discovery of a new way to control the translational motion of the wheel in a molecular shuttle expands the scope of utilizing mechanically interlocked molecules as ligands in traditional coordination and organometallic chemistry.
Data availability
All associated data are in ESI† or deposited with CCDC.
Author contributions
Conceptualization – S. J. L.; investigation – A. D. 1, A. S., R. N. H., and B. H. W.; formal snalysis – A. D. 1, and A. D. 2 (crystallography); writing – original draft, S. J. L.; writing – review & editing, A. D. 1, A. D. 2, and S. J. L.; funding acquisition, S. J. L.
Conflicts of interest
There are no conflicts to declare.
Acknowledgements
This research was supported by the Natural Sciences and Engineering Research Council of Canada (Discovery Grant No. 101694 to SJL). A. D. 1 and S. J. L. acknowledge M. Revington for technical assistance with 2D NMR spectroscopy experiments and Prof. J. Rawson for helpful discussions related to our DFT calculations.
Notes and references
- V. T. Annibale and D. Song, Multidentate actor ligands as versatile platforms for small molecule activation and catalysis, RSC Adv., 2013, 3, 11432–11449 RSC.
- E. C. Constable and C. E. Housecroft, The Early Years of 2,2′-Bipyridine—A Ligand in Its Own Lifetime, Molecules, 2019, 24, 3951 CrossRef CAS PubMed.
- M. D. Sampson, A. D. Nguyen, K. A. Grice, C. E. Moore, A. L. Rheingold and C. P. Kubiak, Manganese Catalysts with Bulky Bipyridine Ligands for the Electrocatalytic Reduction of Carbon Dioxide: Eliminating Dimerization and Altering Catalysis, J. Am. Chem. Soc., 2014, 136, 5460–5471 CrossRef CAS PubMed.
- V. A. Malkov and P. Kocovsky, Chiral Bipyridine Derivatives in Asymmetric Catalysis, Curr. Org. Chem., 2003, 7, 1737–1757 CrossRef.
- E. Peris and R. H. Crabtree, Key factors in pincer ligand design, Chem. Soc. Rev., 2018, 47, 1959–1968 RSC.
- A. E. V. Gorden, J. Xu, K. N. Raymond and P. Durbin, Rational Design of Sequestering Agents for Plutonium and Other Actinides, Chem. Rev., 2003, 103, 4207–4282 CrossRef CAS PubMed.
- D. L. White, P. W. Durbin, N. Jeung and K. N. Raymond, Specific sequestering agents for the actinides. 16. Synthesis and initial biological testing of polydentate oxohydroxypyridinecarboxylate ligands, J. Med. Chem., 1988, 31, 11–18 CrossRef CAS PubMed.
- C. J. Carrano and K. N. Raymond, Ferric ion sequestering agents. 2. Kinetics and mechanism of iron removal from transferrin by enterobactin and synthetic tricatechols, J. Am. Chem. Soc., 1979, 101, 5401–5404 CrossRef CAS.
- I. Turcot, A. Stintzi, J. Xu and K. N. Raymond, Fast biological iron chelators: kinetics of iron removal from human diferric transferrin by multidentate hydroxypyridonates, JBIC, J. Biol. Inorg. Chem., 2000, 5, 634–641 CrossRef CAS PubMed.
- K. Degtyarenko, Bioinorganic motifs: towards functional classification of metalloproteins, Bioinformatics, 2000, 16, 851–864 CrossRef CAS PubMed.
-
J. M. Berg, Principles of bioinorganic chemistry, University Science Books, 1994 Search PubMed.
- A. C. Hachey, D. Havrylyuk and E. C. Glazer, Biological activities of polypyridyl-type ligands: implications for bioinorganic chemistry and light-activated metal complexes, Curr. Opin. Chem. Biol., 2021, 61, 191–202 CrossRef CAS PubMed.
- M. Cirulli, A. Kaur, J. E. M. Lewis, Z. Zhang, J. A. Kitchen, S. M. Goldup and M. M. Roessler, Rotaxane-Based Transition Metal Complexes: Effect of the Mechanical Bond on Structure and Electronic Properties, J. Am. Chem. Soc., 2019, 141, 879–889 CrossRef CAS PubMed.
- S. J. Loeb, Rotaxanes as ligands: from molecules to materials, Chem. Soc. Rev., 2007, 36, 226–235 RSC.
- J. E. M. Lewis, P. D. Beer, S. J. Loeb and S. M. Goldup, Metal ions in the synthesis of interlocked molecules and materials, Chem. Soc. Rev., 2017, 46, 2577–2591 RSC.
- C. O. Dietrich-Buchecker, J. P. Sauvage and J. P. Kintzinger, Une nouvelle famille de molecules : les metallo-catenanes, Tetrahedron Lett., 1983, 24, 5095–5098 CrossRef CAS.
- J.-P. Sauvage, Transition metal-complexed catenanes and rotaxanes as molecular machine prototypes, Chem. Commun., 2005, 1507–1510 RSC.
- A. I. Prikhod'ko, F. Durola and J.-P. Sauvage, Iron(II)-Templated Synthesis of [3]Rotaxanes by Passing Two Threads through the Same Ring, J. Am. Chem. Soc., 2008, 130, 448–449 CrossRef PubMed.
- J.-C. Chambron, J.-P. Collin, V. Heitz, D. Jouvenot, J.-M. Kern, P. Mobian, D. Pomeranc and J.-P. Sauvage, Rotaxanes and Catenanes Built around Octahedral Transition Metals, Eur. J. Org. Chem., 2004, 2004, 1627–1638 CrossRef.
- M. Denis and S. M. Goldup, The active template approach to interlocked molecules, Nat. Rev. Chem., 2017, 1, 0061 CrossRef CAS.
- I. Poleschak, J.-M. Kern and J.-P. Sauvage, A copper-complexed rotaxane in motion: pirouetting of the ring on the millisecond timescale, Chem. Commun., 2004, 474–476 RSC.
- G. Baggi and S. J. Loeb, Rotationally Active Ligands: Dialing-Up the Co-conformations of a [2]Rotaxane for Metal Ion Binding, Angew. Chem., Int. Ed., 2016, 55, 12533–12537 CrossRef CAS PubMed.
- G. Baggi and S. J. Loeb, Rotationally Active Ligands: Dialing-Up Multiple Interlocked Co-Conformations for Silver(I) Coordination, Chem.–Eur. J., 2017, 23, 14163–14166 CrossRef CAS PubMed.
- G. Gholami, K. Zhu, G. Baggi, E. Schott, X. Zarate and S. J. Loeb, Influence of axle length on the rate and mechanism of shuttling in rigid H-shaped [2]rotaxanes, Chem. Sci., 2017, 8, 7718–7723 RSC.
- L. Jiang, J. Okano, A. Orita and J. Otera, Intermittent Molecular Shuttle as a Binary Switch, Angew. Chem., Int. Ed., 2004, 43, 2121–2124 CrossRef CAS PubMed.
- R. G. Pearson, H. B. Gray and F. Basolo, Mechanism of Substitution Reactions of Complex Ions. XVI. Exchange Reactions of Platinum(II) in Various Solvents, J. Am. Chem. Soc., 1960, 82, 787–792 CrossRef CAS.
- Other cations were investigated (Li+, Ag+, Cu+) but resulted in complex mixtures which included significant amounts of [4-H]+.
- K. Nakazono and T. Takata, Neutralization of a sec-Ammonium Group Unusually Stabilized by the “Rotaxane Effect”: Synthesis, Structure, and Dynamic Nature of a “Free” sec-Amine/Crown Ether-Type Rotaxane, Chem.–Eur. J., 2010, 16, 13783–13794 CrossRef CAS PubMed.
- K. Zhu, V. N. Vukotic and S. J. Loeb, Molecular Shuttling of a Compact and Rigid H-Shaped [2]Rotaxane, Angew. Chem., Int. Ed., 2012, 51, 2168–2172 CrossRef CAS PubMed.
- K. Zhu, C. A. O'Keefe, V. N. Vukotic, R. W. Schurko and S. J. Loeb, A molecular shuttle that operates inside a metal–organic framework, Nat. Chem., 2015, 7, 514–519 CrossRef CAS PubMed.
- B. H. Wilson, L. M. Abdulla, R. W. Schurko and S. J. Loeb, Translational dynamics of a non-degenerate molecular shuttle imbedded in a zirconium metal–organic framework, Chem. Sci., 2021, 12, 3944–3951 RSC.
- E. Kimura, T. Shiota, T. Koike, M. Shiro and M. Kodama, A zinc(II) complex of 1,5,9-triazacyclododecane ([12]aneN3) as a model for carbonic anhydrase, J. Am. Chem. Soc., 1990, 112, 5805–5811 CrossRef CAS.
- M. H. Salter, J. H. Reibenspies, S. B. Jones and R. D. Hancock, Lewis Acid Properties of Zinc(II) in Its Cyclen Complex. The Structure of [Zn(Cyclen)(SC(NH2)2)](ClO4)2 and the Bonding of Thiourea to Metal Ions. Some Implications for Zinc Metalloenzymes, Inorg. Chem., 2005, 44, 2791–2797 CrossRef CAS PubMed.
Footnote |
† Electronic supplementary information (ESI) available: Experimental details describing the synthesis and characterization of all new compounds including solution NMR assignments, VT and 2D NMR experiments, crystal structures. CCDC 2248267–2248268. For ESI and crystallographic data in CIF or other electronic format see DOI: https://doi.org/10.1039/d3sc01346d |
|
This journal is © The Royal Society of Chemistry 2023 |
Click here to see how this site uses Cookies. View our privacy policy here.