DOI:
10.1039/D3SC02348F
(Edge Article)
Chem. Sci., 2023,
14, 8924-8935
The interplay between hydrogen and halogen bonding: substituent effects and their role in the hydrogen bond enhanced halogen bond†
Received
6th May 2023
, Accepted 20th July 2023
First published on 21st July 2023
Abstract
The hydrogen bond enhanced halogen bond (HBeXB) has recently been used to effectively improve anion binding, organocatalysis, and protein structure/function. In this study, we present the first systematic investigation of substituent effects in the HBeXB. NMR analysis confirmed intramolecular HBing between the amine and the electron-rich belt of the XB donor (N–H⋯I). Gas-phase density functional theory studies showed that the influence of HBing on the halogen atom is more sensitive to substitution on the HB donor ring (R1). The NMR studies revealed that the intramolecular HBing had a significant impact on receptor performance, resulting in a 50-fold improvement. Additionally, linear free energy relationship (LFER) analysis was employed for the first time to study the substituent effect in the HBeXB. The results showed that substituents on the XB donor ring (R2) had a competing effect where electron donating groups strengthened the HB and weakened the XB. Therefore, selecting an appropriate substituent on the adjacent HB donor ring (R1) could be an alternative and effective way to enhance an electron-rich XB donor. X-ray crystallographic analysis demonstrated that intramolecular HBing plays an important role in the receptor adopting the bidentate conformation. Taken together, the findings imply that modifying distal substituents that affect neighboring noncovalent interactions can have a similar impact to conventional para substitution substituent effects.
Introduction
Understanding how to modulate noncovalent interactions that are in close proximity is paramount to engineering functional materials,1 supramolecular assemblies,2 drugs,3 and catalysts.4 Foundational work has detailed the importance of substituent effects5 to tune the electronics of noncovalent interactions.6 Despite this pioneering work, few have looked at substituent effects involving multiple noncovalent interactions that are spatially close. Recently, Cockroft, and coworkers reported rare experimental data quantifying through-space substituent effects on noncovalent interactions and presented the inadequacy of describing substituent effects using classic Hammett parameters when through-space effects dominate.7 Additionally, Zonta, and coworkers utilized similar methods to carry out an experimental survey of aromatic stacking interactions in solution.8 Clearly, understanding how adjacent noncovalent interactions influence each other by substituent effects is critically lacking.
Among the myriad noncovalent interactions, HBs are privileged for their directionality and tunability. Halogen bonds (XBs) share similarities with HBs, yet contain an electron deficient donor (halogen) that forms an attractive noncovalent interaction with an electron rich species. This interaction can be understood in term of electrostatics and covalency.9 An electrostatic description suggests that due to the polarizability of the halogen, its electron density can become anisotropic. In this case, a partial positive potential develops on the halogen, opposite to the C–X σ bond which has been coined the σ-hole. Concurrently, an electron rich belt is generated on the XB donor which is orthogonal to the direction of the σ bond.10 The high directionality, tunability and complementarity with “soft” Lewis bases,11 makes XBing accordant with HBing. Given these unique properties, exciting applications of XBing supramolecule self-assembly,12 molecular recognition,13 anion binders,14 organocatalysts,15 and anion transporters16 are appearing at a rapid pace. Understanding how to modulate both the strength of the XB and the structure of these molecules is of broad importance for the continued development of functional halogenated species. Using substituent effects to alter the electronics of the halogen donors remains a leading strategy. However, experimental substituent effects on XBing are limited (despite numerous computational studies17). Only Taylor,18 Diederich,19 Erdelyi,20 Stilinović21 and Franz22 have explicitly studied XBing substituent effects in solution—with different outcomes for each of their systems. Despite the critical importance of these substituent studies, the influence of a neighbouring noncovalent interaction has not been studied.
We recently introduced a new strategy—the hydrogen bond enhanced halogen bond (HBeXB)—that directs intramolecular HBs to the electron rich belt of XB donors for preorganization and enhanced XB strength (Fig. 1). Recently, the HBeXB interaction has been used to increase anion binding affinity by nearly an order of magnitude and to improve the function of organocatalysts.14b,15a Similarly, Ho and his group have noted the HBeXB in biological settings and employed it to stabilize and improve a T4 lysozyme mutant.23 These studies have been complemented by fundamental studies as well.14b,24 Over the course of these seminal works there are implications of reciprocity between the hydrogen and halogen bond, especially when considering circumstances where augmentation and preorganization are simultaneously operating. In fact, using substituent effects to modulate the HBeXB may not be straightforward as there are potentially competing electronic effects. Making the halogen atom more electron rich may decrease the XB donor ability but will increase the strength of the adjacent HB. The subtle interplay between these two interactions naturally leads one to ask important questions. For example, when optimizing binding, is it more efficient to tune the XB strength or the HB strength with electronic effects? Additionally, how does this influence molecular conformations? To address these questions and more, we present the first HBeXB substituent and LFER studies in solution, gas, and solid phases.
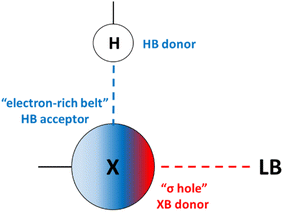 |
| Fig. 1 Cooperativity between HB and XB donors and acceptors in the HBeXB interaction. Blue dashed line: HB interaction, red dashed line: XB interaction, LB: Lewis base. | |
Results and discussion
Receptor design
We previously developed two generations of bis-ethynyl XB receptors that presented two charged pyridinium XB donors in a convergent manner (Fig. 2 top). In the 2nd-generation receptor we introduced an amine substituent to the core that provided intramolecular N–H⋯I HBs to the XB donors. This innovation improved binding by nearly an order of magnitude over a control lacking the amine.14b We coined this effect the HBeXB and showed that the enhancement was due to both preorganization of the receptor into a bidentate binding conformation and strengthening of the XB. The success of the HBeXB and our experience with this scaffold prompted us to evaluate HBeXB substituent effects using a 3rd-generation anion receptor presented here (G3XB, Fig. 2 bottom). This latest iteration replaces the flanking iodopyridinium rings with neutral aromatic rings to improve solubility in organic solvents. The redesign also reduced the number of synthetic steps to produce the diverse range of receptors needed to examine substituent effects. Specifically, we prepared a series of compounds that contained substituents of varying electronic properties that were para to both the amine (R1-G3XB) and the iodine donors (2R2-G3XB). This design permitted systematic modulation of the electron density on both the HB and XB donor rings to test substituent effects. Controls with trifluoromethyl substituents (the strongest electron withdrawing groups in this study) were also prepared without the amine (nHBeXB) and without the iodine XB donors (G3HB).
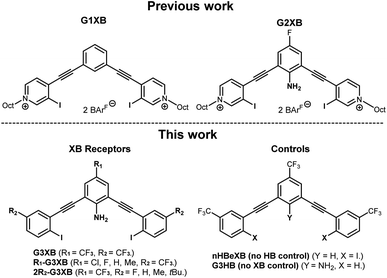 |
| Fig. 2 ChemDraw representation of previous XB receptors and G3XB derivatives in this work. | |
Synthesis and characterization
The synthesis of G3XB derivatives is outlined in Scheme 1. 2,6-Bis(ethynyl)-4-R1-aniline (2) was synthesized by Sonogashira cross-coupling 2,6-dibromo-4-R1-aniline (1) with trimethylsilylacetylene followed by removal of the trimethylsilyl protecting groups with potassium carbonate. Precursor scaffolds containing bromine (R1-3, 2R2-3, and HB receptor G3HB) were synthesized by Sonogashira cross coupling 2 at the iodo-functionality of 4-bromo-3-iodo-R2-benzene or 3-bromotrifluoromethylbenzene, respectively. The iodine containing R1-G3XB and 2R2-G3XB were obtained by microwave assisted halogen exchange of R1-3 or 2R2-3. The complete experimental procedures can be found in the ESI.†
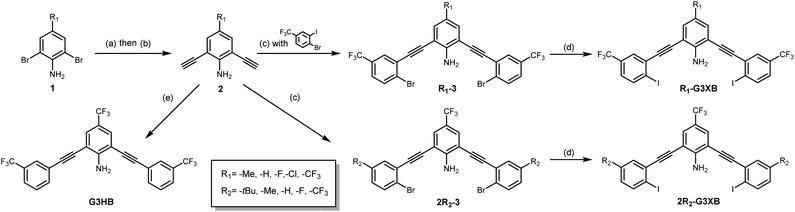 |
| Scheme 1 Synthesis of G3XB derivatives and controls used to study HBeXB substituent effects. Reagents and conditions: (a) TMS-acetylene, Pd(PPh3)2Cl2, Cu(I)I, DIPEA, DMF, overnight, N2, 80 °C; (b) K2CO3, MeOH/DCM (1 : 1 v/v), 4 hours, rt, 45–96%. (c) 4-Bromo-3-iodo-R2-benzene, Pd(PPh3)2Cl2, Cu(I)I, DIPEA, DMF, overnight, N2, rt, 53–87%; (d) NaI, Cu(I)I, trans-N,N-dimethylcyclohexane-1,2-diamine, 1,4-dioxane, microwave reactor, 12–24 hours, 150 °C, 33–84%; (e) 3-bromotrifluoromethylbenzene, Pd(PPh3)2Cl2, Cu(I)I, DIPEA, DMF, overnight, N2, 80 °C, 60%. | |
Experimental evidence of intramolecular hydrogen bonding
Analysis of the amine 1H NMR resonances provided initial indication of intramolecular HBing between the amine and the electron rich belt of the XB donor (N–H⋯I). The analysis provided a preliminary evaluation of substituent effects and provided rare experimental evidence of HBing to larger halogens.24a,25 Control receptor G3HB, lacking XB donors to accept HBs, had an amine 1H chemical shift of 4.64 ppm in C6D6, whereas the chemical shift for G3XB, with iodine acceptors, was 5.53 ppm. This 0.89 ppm downfield shift is indicative of intramolecular HBing. The series of 2R2-G3XB derivatives (Fig. 3, left) showed that as the para substituent on the XB donor ring became more electron donating, the HBing amine proton shifted downfield from 5.53 ppm to 5.80 ppm. This downfield shift occurs despite the expectation that adding electron donating substituents should shield the nuclei and would produce an upfield shift for the proton. However, the electron donating groups in the 2R2-G3XB compounds transfer additional electron density onto the iodine atoms (vide infra) making the iodine a better HB acceptor, resulting in the downfield shift.‡ Overall this NMR analysis suggests that the intramolecular HBing is formed and the strength of this HBing correlates with the electron density of the halogens.
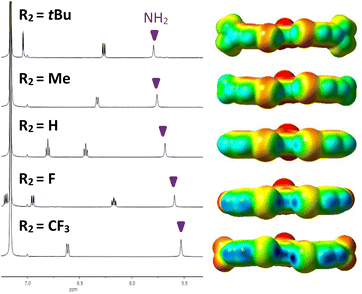 |
| Fig. 3
1H NMR spectra (C6D6, 500 MHz, 5 mM) of 2R2-G3XB derivatives (left) highlighting the HBing downfield shift of the amine as the halogen becomes more electron rich; ESP maps (isovalue = 0.001 a.u.) for 2R2-G3XB derivatives (right) are displayed on the same scale. Electron deficient regions are blue and electron rich regions are red. | |
Computational evaluations
To garner preliminary insight into substituent effects of HBeXBs, in silico studies were performed. All the receptors were evaluated using Gaussian 16 at the M06-2X/def2TZVPP level of theory (see ESI† for further details). A systematic use of conformational analysis and electrostatic potential (ESP) mapping provided early insight into the synergy between the HB and XB and helped to stimulate deeper LFER analysis.
σ-Hole calculations
σ-Hole analysis (maximum/minimum electrostatic potentials, denoted by Vs,max/Vs,min) showed several trends regarding the influence of the intramolecular HB on XB donor strength. Consistent with our previous studies, the HB from the amine to the iodine augments the σ-hole (greater Vs,max). For example, G3XB in the bidentate conformation has a Vs,max that is 4.02 kcal mol−1 greater than nHBeXB—a consequence of the HBeXB (Table 1).
Table 1 Halogen Vs,max of G3XB derivativesa
|
Bidentate |
S conformation (HBed iodine/non-HBed iodine) |
ΔVs,maxb |
V
s,max (kcal mol−1) were calculated at 0.001 Å isoelectric surface.
ΔVs,max = Vs,max HBed iodine − Vs,max, non-HBed iodine.
|
G3XB
|
32.05 |
31.45/24.46 |
6.99 |
nHBeXB
|
28.03 |
26.64/25.39 |
1.25 |
2F-G3XB
|
28.40 |
28.52/20.94 |
7.58 |
2H-G3XB
|
24.98 |
26.05/17.95 |
8.10 |
2Me-G3XB
|
23.03 |
24.69/16.94 |
7.75 |
2tBu-G3XB
|
22.41 |
24.37/16.15 |
8.22 |
Cl-G3XB
|
30.84 |
29.85/24.16 |
5.69 |
F-G3XB
|
30.12 |
29.33/24.61 |
4.72 |
H-G3XB
|
28.72 |
28.08/24.07 |
4.01 |
Me-G3XB
|
28.11 |
27.36/24.04 |
3.31 |
Substitution on the HB donor ring
Modulation of the R1 group helped determine if stronger HBs would correlate to greater XB enhancement. Within the R1 series, the Vs,max values on the XB donors ranged from 32.05 kcal mol−1 for the most electron withdrawing G3XB in the bidentate conformation to 28.11 kcal mol−1 for Me-G3XB (Table 1). The data verify that stronger HB donors correlate with a more positive Vs,max—confirming a notion alluded to in our previous HBeXB systems.14b,24a Our results here highlight that tuning the HB donor with a single remote substituent can influence the XB donor Vs,max by 3.94 kcal mol−1.
Substitution on the XB donor ring
Next, the Vs,max of the 2R2-G3XB derivatives in the bidentate conformation was probed to determine how substituent electronics on the XBing ring influence XB donor strength. As expected, the Vs,max becomes larger as the substituent para to the XB donor increases in electron withdrawing capacity. The G3XB derivative with CF3 substituents represents the upper end in this series with a Vs,max of 32.05 kcal mol−1 whereas, 2tBu-G3XB represents the lower end at 22.41 kcal mol−1. These studies align with previous reports18,19,22 showing that XB donors can be directly influenced by electronic substituent effects. For instance, the Vs,max of the XB donors in Talyor's 4-R-C6F4I studies differ by 6.9 kcal mol−1 when a para fluoro substituent is changed to a piperidyl group.18 However, it is notable that in this bisethynyl system the Vs,max varies by 9.64 kcal mol−1 by direct substitution on the XB ring.
In contrast to the above discussion on XB donor strength (i.e. Vs,max), we also considered the Vs,min of the iodine atoms as a measure of HB acceptor capacity. As the substituent para to the XB donor becomes more electron donating, the iodine species becomes more electron-rich (Fig. 3). The Vs,min of the 2R2-G3XB compounds (−1.11 to −11.14 kcal mol−1, for details see ESI†) trend with the downfield NMR shifting—suggesting that R2 electron donating groups strengthen the intramolecular HBing between the amine and the XB donor.
Conformational effects on the XB donor
R1 – minimal through bond effects on the XB donor.
ESP maps were calculated for the R1-G3XB derivatives in the S conformation (Fig. 4). The S conformation contains one XB donor that accepts a HB and one that does not. This S arrangement was used to determine whether substitution on the HB donor ring has a through bond electronic effect on the XB donor. The S conformation of R1-G3XB derivatives all exhibit similar Vs,max values (≈24 kcal mol−1) for the iodine not accepting a HB. This demonstrates that substituents on the central HB donor ring do not directly inductively alter the electronics of the XB donor.§
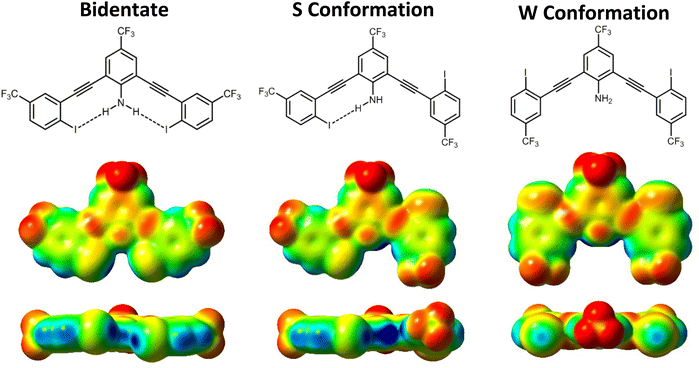 |
| Fig. 4 ChemDraws and ESP maps for G3XB in all three planar conformations. The bidentate conformation (left), where both XB donors are convergent; the S conformation (middle), where the XB donors are on opposite sides of the molecule; and the W conformation (right), where both XBs are directed away from the amine. All ESP maps are displayed on the same scale. Electron deficient regions are blue and electron rich regions are red. | |
R1 – through space effects on the XB donor.
In contrast, the potency of the HB donor does have an influence on the XB donor strength. The Vs,max of the halogen accepting a HB has a greater ESP than the non-HB accepting iodine. The Vs,max values for the iodine in G3XB that accepts a HB was 31.45 kcal mol−1 and 27.36 kcal mol−1 for Me-G3XB which has the most electron donating substituent R1. Thus, strengthening the intramolecular HB donor can modulate the Vs,max of a single XB donor by 4.09 kcal mol−1, a value similar to the bidentate assessment described above.
The through space influence of a HB on the XB donor was analyzed by computing the difference (ΔVs,max) between the two iodine donors in the S conformation (ΔVs,max = Vs,max HBed iodine − Vs,max non-HBed iodine). Here the weakest HB donor Me-G3XB derivative had a ΔVs,max of 3.31 kcal mol−1 while the strongest HB donor G3XB had the largest ΔVs,max of 6.99 kcal mol−1. Collectively the ΔVs,max values of the R1-G3XB derivatives adhere to the trend that increasing the electron withdrawing ability of R1, strengthens the HB which in turn has a larger influence on the XB donor.
R2 – through bond effects on the XB donor ring¶.
V
s,max values for 2R2-G3XB derivatives in the S conformation were used to quantify how changing the electronics of the XB donor ring influences the iodine σ-hole. For example, the Vs,max of the externally directed iodine (non-HBed iodine) generally followed the trends expected from the electronic contributions of the R2 group (i.e. Vs,max values for 2R2-G3XB trended in the order CF3 > F > H > Me > tBu) ranging from 24.46 to 16.15 kcal mol−1. The internally directed iodine atoms (accepting a HB) all had larger Vs,max values ranging from 31.45 to 24.37 kcal mol−1 and generally followed the same trend.
R2 – through space effects on the XB donor†.
The strength of the HB donor in the 2R2-G3XB derivatives is constant (i.e. a CF3 group para to the central amine). Thus, the ΔVs,max values here are a measure of how the R2 electronics impact the halogen as both a XB donor and a HB acceptor. The ΔVs,max values of 6.99, 7.58, 8.10, 7.75 and 8.22 kcal mol−1 were obtained for G3XB, 2F-G3XB, 2H-G3XB, 2Me-G3XB and 2tBu-G3XB, respectively. The trend parallels previous evaluations and shows that generally, more electron rich iodines experience a greater augmentation.
Comparing 2R2-G3XB and R1-G3XB ΔVs,max values provides a measure of which substituent position impacts XBing the most by a through space effect. The smaller range of values for the 2R2-G3XB series (1.23 kcal mol−1), as compared to the R1-G3XB derivatives (3.68 kcal mol−1), suggests that the influence of HBing on the halogen atom is more sensitive to substitution on the HB donor ring. For example, altering one R1 substituent from CF3 to Me results in a 3.68 kcal mol−1 ΔVs,max difference. However, altering two R2 substituents from CF3 to Me results in a 0.76 kcal mol−1 ΔVs,max difference.
Influence of R1 and R2 on conformation.
To assess the role of preorganization in G3XB derivatives, their relative stabilities were assessed based on electronic energies from DFT. The difference between the S and bidentate conformation energy illustrates that intramolecular HBeXBs stabilize the bidentate conformation of the receptors (Table 2). The bidentate conformation of G3XB contains two intramolecular HBs (N–H⋯I) and is more stable than the S conformation by 1.53 kcal mol−1. The W form, lacking intramolecular HBs is 3.11 kcal mol−1 higher in energy than the bidentate conformation. For the 2R2-G3XB series, as R2 becomes more electron donating, the energy differences between the bidentate and S conformation increases from 1.76 kcal mol−1 for 2F-G3XB to 2.15 kcal mol−1 for 2tBu-G3XB. This suggests a greater stabilization when the iodine HB acceptor is more electron rich. These results track with the amine 1H NMR chemical shift analysis for 2R2-G3XB. In contrast, the difference between the bidentate and S conformation for the R1-G3XB series is comparatively attenuated; however, only a single substituent group is modified. The ΔE is 1.42 kcal mol−1, 1.33 kcal mol−1, 1.43 kcal mol−1, and 1.36 kcal mol−1 for Cl-G3XB, F-G3XB, H-G3XB and Me-G3XB, respectively. These data indicate that conformational preference is sensitive to the electronics of both the XB and HB donor.
Table 2 Electronic energy difference (ΔE) between S and bidentate conformations of G3XB derivatives
|
ΔE (kcal mol−1) |
G3XB
|
1.53 |
nHBeXB
|
−0.01 |
2F-G3XB
|
1.76 |
2H-G3XB
|
1.83 |
2Me-G3XB
|
1.90 |
2tBu-G3XB
|
2.15 |
Cl-G3XB
|
1.42 |
F-G3XB
|
1.33 |
H-G3XB
|
1.43 |
Me-G3XB
|
1.36 |
Solution studies
NMR titrations and association constants
1H NMR anion binding titrations were performed to quantify HBeXB substituent effects in solution. Titrations were conducted in C6D6 with tetra-n-hexylammonium iodide (THAI) as the guest to ensure all complexes remained in solution. The addition of THAI resulted in downfield shifts for nearly all of the 1H NMR signals on the receptors, (except for a center core singlet of nHBeXB). Bindfit27 was used to fit the changes in the 1H NMR signals to a 1
:
1 binding model. Iterative and simultaneous refinement of multiple isotherms provided association constants (Ka) for all scaffolds (Table 3).
Table 3 Measured association constants and binding energies for G3XB
a
Host |
K
a (M−1) |
ΔGbinding (kcal mol−1) |
The Ka values are reported as the average of three titration experiments. All titrations were performed in C6D6; two significant figures are reported and errors are estimated at 10%. Tetra-n-hexylammonium iodide was used and titrations were performed at 25 °C. Bindfit was used to fit changes in chemical shift to a stepwise 1 : 1 host–guest binding model. The free energy of binding (ΔGbinding) was calculated from the association constant.
|
G3XB
|
420 |
−3.6 |
G3HB
|
30 |
−2.1 |
nHBeXB
|
10 |
−1.5 |
2F-G3XB
|
170 |
−3.0 |
2H-G3XB
|
70 |
−2.5 |
2Me-G3XB
|
50 |
−2.3 |
2tBu-G3XB
|
50 |
−2.3 |
Cl-G3XB
|
330 |
−3.4 |
F-G3XB
|
250 |
−3.3 |
H-G3XB
|
190 |
−3.3 |
Me-G3XB
|
170 |
−3.1 |
Role of intramolecular HBing on anion binding
G3XB (all R groups –CF3) had the strongest binding (420 M−1) which was nearly 14 times greater than the isostructural no XB control (G3HB) (30 M−1). The substantially lower binding affinity of G3HB suggests that the amine doesn't significantly HB to the iodide guest; the amine of the G3XB derivatives largely forms intramolecular HBs with the iodine XB donors. The considerable influence of the intramolecular N–H⋯I HBs is evident when comparing G3XB to the control lacking an amine (nHBeXB). nHBeXB exhibited very weak binding in solution with a Ka = 10 M−1. The nearly 50-fold difference in Ka between G3XB and nHBeXB demonstrates the striking impact that a weak intramolecular N–H⋯I HB can have on receptor performance. The HBeXB enhancement is far greater than our original HBeXB study using a dicationic receptor where only a 9-fold increase was observed.14b The greater HBeXB influence in this study could be due to the iodine XB donors of G3XB being more electron rich (i.e. neutral receptor) than the iodopyridinium donors previously evaluated—allowing for stronger HBeXB and greater preorganization. It could also be attributed to solvent effects as the two studies were conducted in significantly different media (C6D6vs. 60% CD3NO2/40% CDCl3). These binding studies highlight that the central amine interacts minimally with the iodide and largely operates as an intramolecular HB donor to the iodine XB donor atoms.
2R2-G3XB substituent effects on anion binding
The 2R2-G3XB (R2
CF3, F, H, Me, tBu) series of molecules were used to quantify how substituents para to the XB donor influence the HBeXB. Varying these substituents resulted in association constants for THAI ranging from 50 M−1 to 420 M−1 (Table 3). Having a stronger electron withdrawing group para to the XB donor increases the XB strength and for this series of compounds this generally holds true. G3XB (R2
CF3) maintained the greatest affinity followed by 2F-G3XB with a Ka = 170 M−1, which is 60% less than G3XB. 2H-G3XB binding was further diminished to a Ka of 70 M−1. The most electron rich 2tBu-G3XB exhibited similar iodide binding with the 2Me-G3XB derivative (50 vs. 50 M−1, respectively). Nevertheless, the general trend in substituent effects matches previous studies for XB derivatives.18–22
R1-G3XB substituent effects on anion binding
While studies have evaluated the influence of functional groups on the XB and HB independently none have considered their interplay. Here binding studies of R1-G3XB derivatives represent the first experimental consideration of this.
The binding data for R1-G3XB derivatives (R1
CF3, Cl, F, H, Me) highlights that stronger intramolecular HBing enhances the XB receptor binding affinity. By increasing the electron withdrawing capacity of the substituent para to the amine (strengthening the HB donor) from a Me group to a CF3 group the binding increased 2.5-fold. Me-G3XB, the most electron rich of the R1-G3XB series, had the lowest association constant (170 M−1), while the most electron deficient G3XB had the highest (420 M−1). This result reveals an effective way to increase the overall XB binding ability in a system which includes an intramolecular HB to XB donor—electronically tuning the HB with substituents rather than the XB.
R1 and R2 interplay: receptor performance
As noted above, the receptor performance can be modulated by either changing the substituents para to the XB donor or para to the HB donor. To further quantify the substituent effects on binding ΔGbinding was calculated for each receptor. The binding energy for 2R2-G3XB derivatives can be tuned by 1.3 kcal mol−1 simply by changing out the two CF3 groups to Me groups on the XBing rings. Intriguingly, altering only one substituent (from CF3 to Me) on the center ring can elicit a 0.5 kcal mol−1 change. This suggests that binding can be modified by a comparable amount with a smaller structural change to the receptor. These small energetic changes can have large implications, as previously demonstrated in a study of XB catalyst transition state binding.28
R1 and R2 interplay: linear free energy relationships
Linear free energy relationships (LFERs) are gaining importance in understanding substituent effects on noncovalent interactions like HBing, XBing, chalcogen bonding, cation–π and π–π.17,29 There are surprisingly few studies that have experimentally examined substituent effects by evaluating LFERs on the XB.18–22 Taylor and coworkers adeptly used this approach to evaluate the XB between para-substituted tetrafluoro-iodobenzene and tributylphosphine oxide (Fig. 5a). These studies showed the best correlation of association constants with the σmeta parameter (R2(σm) = 0.94 vs. σparaR2(σp) = 0.82) which they attributed to inductive/field effects being more dominant.18 In contrast, Diederich19 and Franz22 interestingly reported strong correlation for the σpara parameters. Diederich evaluated XBing between 4-R-iodoethynylbenzene and quinuclidine (R2(σp) = 0.97 vs. R2(σm) = 0.82, Fig. 5b). The strong correlation with the σpara parameter in this case indicated that substituents largely influence the halogen donor through resonance in this conjugated system. Finally, Erdélyi20 and Stilinović21 investigated substituent effects on XB acceptors. In Erdélyi's case, the three-center [N–I–N]+ XB (Fig. 5c) exhibited linear correlation (R2 = 0.97) between the calculated natural atomic populations with σpara parameters. In all cases, substituent effects were shown to have a significant influence on XBing. Nevertheless, each of these examples showed great correlation with different parameters for their LFERs. Despite these important studies, there are no experimental examples analyzing substituent effects on adjacent noncovalent interactions. Supramolecular contacts don't occur in an isolated environment and as such, it is essential to understand whether traditional substituent effects hold true in these situations. Herein, we evaluated substituent effects on the HBeXB and used LFERs to establish whether changing the electronics of the HB donor has the same influence on the overall binding as changing the electronics of the XB donor.
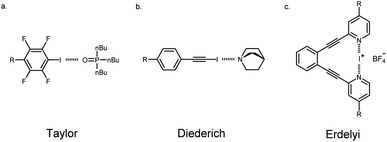 |
| Fig. 5 Selected examples of systems that were used to study halogen bonding LFER. | |
Our initial evaluation of LFERs compared the bidentate ESP values (Vs,max) of receptors with experimental ΔG values from titration studies. While XBing is known to encompass both covalent and electrostatic components,9 plots of ESP vs. ΔG can establish the degree of electrostatic contribution in these particular HBeXB complexes. The following results demonstrate that in this system, the substituent influence is largely electrostatic in nature.
The 2R2-G3XB derivatives show strong correlation between the electrostatics (Vs,max) of the XB donor and the iodide binding in solution. The non-normalized plots (Fig. 6, top) are linear for the 2R2-G3XB derivatives (R2 = 0.99). Since the modification was directly on the XBing ring, this finding was expected given previous LFER studies on the XB.18
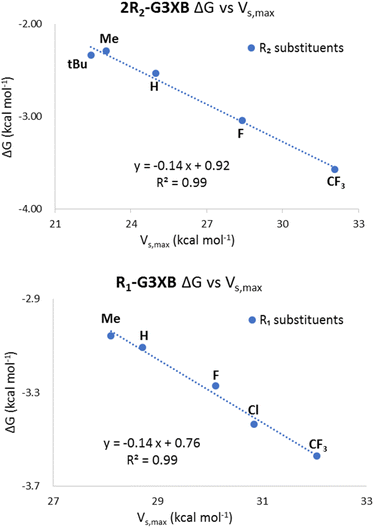 |
| Fig. 6 Non-normalized plots of the ESPs and binding energies for 2R2-G3XB (top) and R1-G3XB (bottom). | |
In contrast, the R1-G3XB derivatives provided original insight into how the amine HB donor augments the XB donor strength. The LFER analysis for the R1-G3XB series certainly suggests an electrostatic origin (Fig. 6, bottom) as the plots are again linear (R2 = 0.99). Collectively the ESP vs. ΔG plots indicates that the HBeXB iodide binding of the G3XB derivatives is largely governed by electrostatics and that ESP maps accurately model the influence of intramolecular HBs on the XBs in this system.
We extended our LFER analysis by evaluating the correlation between our experimental association constants and Hammett parameters (σmeta and σpara). While Hammett parameters were originally used to model the ionization reaction of benzoic acid, they have been increasingly used to study noncovalent interactions.6–8 We used Hammett parameters to analyze possible inductive (or field effects as proposed by Wheeler and Houk30) and resonance effects on the HBeXB receptor binding—an approach recently used by Hunter to effectively assess HB cooperativity.31
Normalized association constants (log(Kr/KH)) and the corresponding substituent parameters (σ) were fit using the Hammett equation shown below. ρ represents the slope.
The R1-G3XB derivatives show a more linear correlation with the σpara parameter (R2(σp) = 0.93) than with the σmeta (R2(σm) = 0.87), indicating resonance effects are more important than inductive effects on the HB donor of the HBeXB (Fig. 7, top). Electron withdrawing R1 substituents enhance the amine donor strength, thus leading to stronger intramolecular N–H⋯I HBing. A stronger HB in turn makes the XB donor more electron deficient. Thus, the resonance of the R1 substituent work in concert with both the HB and XB donors, resulting in a linear correlation with the σpara parameters.
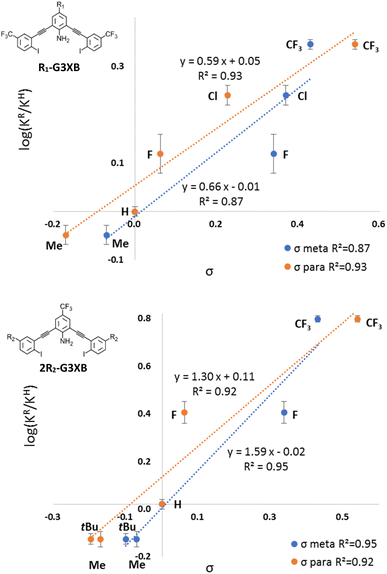 |
| Fig. 7 Normalized Hammett plots of K values of R1-G3XB (top) and 2R2-G3XB (bottom) with σmeta (blue) and σpara (orange). | |
Curiously, normalized Hammett plots of Ka values for 2R2-G3XB resulted in similar linear fits with both the σmeta and σpara parameters (Fig. 7, bottom). The fit with the σmeta parameters is R2(σmeta) = 0.95 while the fit with the σpara parameters is R2(σpara) = 0.92, implying that inductive effects may play a modestly more important role on substituent effects. As noted above, substituent effects on the XB have been found to be attributed to either inductive18 or π-resonance effects.19,20 It is atypical in LFER studies to obtain linear fits for both the σpara and the σmeta parameters simultaneously.32 Unlike previous LFER studies, in this case there are two noncovalent interactions involved (N–H⋯I HBing and C–I⋯I− XBing). The halogen here functions as both a XB donor and a HB acceptor. The electronics of the R2 substituents can have competing influences on the noncovalent interactions. Thus, increasing the electron density on the halogen should weaken the XB donor but strengthen the intramolecular HBs with the amine. This competing effect produces good correlation with both the σpara and the σmeta parameters. Thus, when competing electronic influences are present, selecting an appropriate para substituent for a XB donor could be tricky if relying on Hammett parameters. We also looked for linear correlations between the association constants and other parameters including Taft's σI, σR,6d sEDA and pEDA.33 However, no linear correlations were obtained (see ESI†). The combined LFERs herein, suggest that choosing an appropriate substituent (R1) to tune adjacent noncovalent interactions could complement the common strategy of directly altering the electronics of the XB donor (R2) to modulate binding.
Classically, the slope ρ in the Hammett equation describes the susceptibility of the reaction to substituents. While studying noncovalent interactions, ρ provides a measure of how sensitive the interaction is to substituent effects as compared to the ionization of benzoic acid. The ρ value of the R1-G3XB Hammett plot (ρR1 = 0.59) is between 0 and 1 indicating that the binding is sensitive to electronics (although not as much as the ionization of benzoic acid). More importantly, the ρ value for R1-G3XB can be compared to 2R2-G3XB (statistically taking into consideration the number of substituents); thereby, determining quantitatively which substituent position has a greater influence on receptor performance. There are two R2 groups affecting the anion binding per receptor, so half the ρ value was used for comparison. The 1/2ρ value for R2-G3XB (1/2ρR2 = 0.79) is only modestly higher than the one obtained for the R1-G3XB series (ρR1 = 0.59). This is notable as the R1 substituents are much further from the binding site and maintain minimal through bond electronic influence on the XB. The data here indicate that altering the electronics of an HB donor within the context of the HBeXB can have a similar effect on XB strength as traditional substituent effects.
For a more thorough picture of how resonance contributes to the interaction, multivariable linear regression of the normalized association constants (log(Kr/KH)) with the field (F) and resonance (R) parameters in the Swain–Lupton equation5b were conducted in Matlab. The percent resonance contribution (%R) in this equation affords a simple and meaningful way to assess the relative importance of field (F) and resonance (R) effects.
log(KX/KH) = ρfF + ρrR + i |
| %R = ρr/(ρf + ρr) × 100 |
(1)
|
As shown in Table 4, %R = 49% for 2R2-G3XB which suggests that the binding is slightly more governed by inductive/field effects from the substituents. The %R for 2R2-G3XB reveals the similarity in the impacts of resonance and inductive/field effects on the XB donor ring's R2 substituents. This similarity aligns with the 2R2-G3XB Hammett plots, which exhibit linear relationships with both the σpara and σmeta parameters due to the competing influences from the electronics of the R2. In contrast, the substituent effects in R1-G3XB are more dependent on resonance where %R = 54%. However, it should be noted that the Hammett studies employed used relatively few parameters which limits the statistical strength of this Swain Lupton analysis.
Table 4 Field and resonance fitting parameters
|
ρ
f
|
ρ
r
|
R
2
|
%R |
2R2-G3XB
|
0.95 |
0.90 |
0.99 |
49% |
R1-G3XB
|
0.37 |
0.44 |
0.99 |
54% |
Crystal structures
HBeXB impact on solid-state features.
Crystal structures of several receptors were obtained to further evaluate substituent effects. While previous studies have evaluated the HBeXB in the solid-state,11a,14b,23a,24a the HBeXB bidentate receptors reported here are the first structures considered the structures within the context of substituent effects.
The initial solid-state assessment of the neutral receptors focused on three species (G3XB, G3HB, nHBeXB) to identify the influence of the HBeXB. Previous generations were charged and thus in the solid state could be more influenced by induced fit binding. This series further confirms that the amine HBs promote a bidentate conformation.
As shown in Fig. 8 (left), the G3XB structure displays convergent bidentate XBing conformations promoted by the intramolecular HBing with N–H⋯I distances and angles of 3.12(4) Å, 163(3)° and 3.20(3) Å, 165(4)°. G3XB crystalized in the monoclinic space group P21/c with a single molecule in the asymmetric unit. The XB donors are directed towards an iodine atom of an adjacent molecule. One of the iodine atoms forms a XB with C–I⋯I distances and angles of 3.9379(6) and 167.38(11)° (RII = 0.97).34 The other halogen while directed at an iodine, is too far to XB with a C–I⋯I distance of 4.2007(11) and 173.56(9)°.
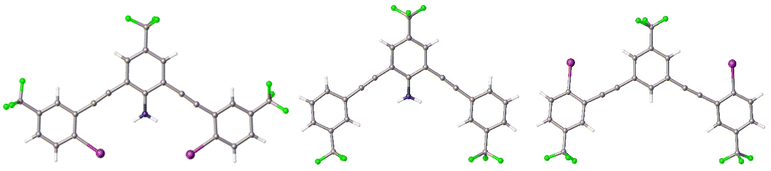 |
| Fig. 8 Crystal structures of G3XB molecule (left), G3HB (middle)*, and nHBeXB (right) highlighting the importance of the intramolecular HB to produce the bidentate conformation. * Representative example from the orthorhombic polymorph. | |
Notably, the exchange of the central amine group for a CH proton results in a molecule that adopts the W conformation (Fig. 8 right), the form energetically favoured in the computational analysis. nHBeXB crystalizes in P21/c with a single molecule in the asymmetric unit. One of the iodine atoms forms a XB with a symmetrically equivalent species on an adjacent molecule (C–I⋯I of 160.91(16)° and 3.8065(6) Å (RII = 0.93)). The other iodine has type II halogen contacts35 with disordered fluorine atoms of an adjacent molecule, with contacts that are less than the sum of the van der Waals radii.
Two crystal structures were obtained of G3HB, a triclinic and orthorhombic polymorph. Neither structure adopted a bidentate conformation. The triclinic (P
) form has a single molecule in the asymmetric unit, and does not adopt one of the planar forms (i.e. bidentate, S, or W). The central amine does not play a significant role in the packing. The orthorhombic (Fdd2) structure of G3HB has two molecules in the asymmetric unit. While each molecule adopts a W conformation, one molecule is much less planar than the other. The distortion of one species in the asymmetric unit may come from the arms having to deflect to maintain a head-to-tail HBing chain.
The systematic changes of XB donors and HB donors in the G3XB, G3HB, nHBeXB series highlights that the N–H⋯I intramolecular HBing plays a key role in the receptor adopting the bidentate conformation. This is further demonstrated in the structures of derivatives containing different substituents on the flanking iodine containing arms.
2R2-G3XB in the solid state.
To further consider the interplay between the XB and HB we obtained crystal structures of species that modulate the electron density on the XB donating arms. Altering the functional group para to the iodine donor permitted us to evaluate two different features related to HBeXB within this system. First, the ability of the N–H⋯I HBing to bias the bidentate conformation was evaluated. Second, the first solid state evaluation of substituent effects with relation to the HB acceptor capacity of iodine donors was investigated.
Despite great efforts, only G3XB and 2H-G3XB were crystallized from the 2R2-G3XB series. As expected, each of the molecules in this series maintains intramolecular N–H⋯I HBs and adopt bidentate conformations. 2H-G3XB crystalizes in the monoclinic P21 space group with two molecules in the unit cell. The 2H-G3XB molecules have HB parameters of 3.16(4) Å 164(4)°, 3.00(7) Å, 170(7)°, 3.16(7) Å 165(5)°, and 2.99(6) Å 169(6)°, that are shorter than G3XB.
The planarity of the molecules did not follow the expected trend. For example, despite having shorter HB contacts 2H-G3XB was more distorted than G3XB. Angles between flanking rings and central rings of the two unique 2H-G3XB species were 1.68° and 15.54° for one receptor and for the other was 4.66° and 17.53°. A partial explanation for this is that the two structures have different long range packing features (see ESI Fig. S90†).
The angle formed by the centroids of the three rings (i.e. flanking-core-flanking angle) provides another structural measurement that indicates HBing strength between the amine hydrogen and more electron rich iodine atoms. For example, a smaller angle would suggest a stronger attraction distorting the alkyne bonds linking the core to the arms. Comparing G3XB, and 2H-G3XB, the increasing electron density on the iodine led to greater distortion with angles of 118.94° for G3XBvs. 117.18° for 2H-G3XB.
R1-G3XB in the solid state.
Several R1-G3XB derivatives (Me-G3XB, F-G3XB, and G3XB) that modulate the electron density of the central amine were also crystalized to evaluate the effects that these substituents have on the solid-state structures.
Similar to the 2R2-G3XB series, initial analysis compared the HB distances. G3XB maintained the shortest N–H⋯I HB contacts (see above for distances and angles) as suggested from the electron withdrawing nature of the CF3 group. In contrast, the longest N–H⋯I HB contacts came from the Me-G3XB derivative. This methyl species crystalized in the monoclinic space group P21/c with a single receptor in the asymmetric unit that adopts the bidentate conformation. The N–H⋯I HB contacts were 3.16(6) Å, 154(7)°, and 3.48(5) Å, 148(5)°. The longer HB contacts are attributed to the electron donating nature of the methyl group.
Unfortunately, F-G3XB adopted the S conformation making comparisons across the series irrelevant. However, comparison of the bidendate G3XB and Me-G3XB suggests that the electronics of the aniline core may have some structural impact on the receptor. For example, the weaker HBs of Me-G3XB resulted in a less planar receptor. For Me-G3XB the angles between the planes of the core and flanking arms were 5.29° and 8.75° as compared to G3XB which was 1.06° and 8.59°. Another parameter evaluated was the angle formed by the centroids of the three rings. For the electron rich Me-G3XB this angle is 123.16° whereas for G3XB the angle is 118.94°. This suggests that the amine donors are stronger in G3XB causing a slight distortion due to the stronger HBs between the amine hydrogen atoms and the iodine atoms.
G3XB and derivatives as cocrystal-salts.
To probe receptor binding in the solid-state the various derivatives were crystalized with tetraalkylammonium chloride salts. Despite our efforts only four successful cocrystal-salts were obtained (G3XB·Cl−, 2H-G3XB·Cl−, 2Me-G3XB·Cl−, and H-G3XB·Cl−). Unfortunately, the different tetraalkylammonium salts present and the crystal packing differences, made comparisons difficult (see ESI†). Despite this, all the structures maintain a bidentate conformation. Additionally, the XB contacts are quite strong with reduction ratios ≤0.85.
Conclusions
In this work, we reported the first LFER studies for substituent effects on the HBeXB interaction. Electrostatic surface potentials (ESP) were used to assess the electrostatic contribution to the interaction. A strong correlation between computational ESP values and solution binding data illustrated the electrostatic nature of this cooperative interaction. Hammett plots constructed with iodide association constants for R1-G3XB and 2R2-G3XB showed that the electronics of both the HB and XB are critically important to the binding. Resonance effects of electron withdrawing R1 substituents strengthened both the HB and XB and enhanced the overall HBeXB binding. Electron withdrawing groups of R1 substituents generated a more potent HB donor which better polarized the XB and further promoted the bidentate conformation. In contrast, the electronics of the R2 substituents had competing effects on the HB and XB. Specifically, electron donating groups para to the iodine atoms (R2) decreased the XB donor ability but made the halogen a better HB acceptor. From a design standpoint, this implies that when modulating electron density on the halogen one can enhance the preorganization of a receptor by increasing electron density on the halogen (improved HB acceptor capacity) at the expense of a slightly weakened XB. Our X-ray crystallography studies further demonstrated the role of the HBeXB on preorganizing molecular structure. Combined, the solution experiments, computations and crystallography provided a rare example of how substituents affect proximal noncovalent interactions. The results from this study also provides important insights for the design of receptors or catalysts—altering remote substituents which electronically influence adjacent noncovalent interactions (instead of direct para substitution) can have similar impact to traditional substituent effects and should be considered for molecular design.
Data availability
The corresponding data can be found in the ESI.†
Author contributions
JS and OBB conceptualized the project. JS and EAJ conducted synthesis and characterization. VSB conducted computational studies. JS conducted the solution studies. DAD conducted solid-state studies. JS and DAD wrote the paper. OBB supervised the investigation and provided editorial assistance during manuscript preparation. All authors examined the data, results, and conclusions presented here.
Conflicts of interest
There are no conflicts to declare.
Acknowledgements
This work was funded by the National Science Foundation (NSF) CHE-2004213, the Center for Biomolecular Structure and Dynamics CoBRE (NIH NIGMS grant P30GM140963), Montana University System MREDI 51030-MUSRI2015-02, and the University of Montana (UM). The X-ray crystallographic data were collected using a Bruker D8 Venture, principally supported by NSF MRI CHE-1337908. The work at the Oak Ridge National Laboratory was supported by U.S. Department of Energy, Office of Science, Basic Energy Sciences, Chemical Sciences, Geosciences, and Biosciences Division. This research used resources of the Compute and Data Environment for Science (CADES) at the Oak Ridge National Laboratory and the National Energy Research Scientific Computing Center (NERSC), which are supported by the Office of Science of the U.S. Department of Energy under Contracts No. DE-AC05-00OR22725 and No. DE-AC02-05CH11231, respectively.
References
- K. Liu, Y. Kang, Z. Wang and X. Zhang, Adv. Mater., 2013, 25, 5530–5548 CrossRef CAS PubMed.
- C. Rest, R. Kandanelli and G. Fernández, Chem. Soc. Rev., 2015, 44, 2543–2572 RSC.
- S. U. Rehman, T. Sarwar, M. A. Husain, H. M. Ishqi and M. Tabish, Arch. Biochem. Biophys., 2015, 576, 49–60 CrossRef PubMed.
- M. Raynal, P. Ballester, A. Vidal-Ferran and P. W. van Leeuwen, Chem. Soc. Rev., 2014, 43, 1660–1733 RSC.
-
(a) C. Hansch, A. Leo and R. Taft, Chem. Rev., 1991, 91, 165–195 CrossRef CAS;
(b) C. G. Swain and E. C. Lupton, J. Am. Chem. Soc., 1968, 90, 4328–4337 CrossRef CAS.
-
(a) S. E. Wheeler, Acc. Chem. Res., 2013, 46, 1029–1038 CrossRef CAS PubMed;
(b) C. S. Wilcox, E.-i. Kim, D. Romano, L. H. Kuo, A. L. Burt and D. P. Curran, Tetrahedron, 1995, 51, 621–634 CrossRef CAS;
(c) B. W. Tresca, R. J. Hansen, C. V. Chau, B. P. Hay, L. N. Zakharov, M. M. Haley and D. W. Johnson, J. Am. Chem. Soc., 2015, 137, 14959–14967 CrossRef CAS PubMed;
(d) M. Charton, Prog. Phys. Organ. Chem., 1981, 119–251 CAS.
- R. J. Burns, I. K. Mati, K. B. Muchowska, C. Adam and S. L. Cockroft, Angew. Chem., 2020, 132, 16860–16867 CrossRef.
- C. Bravin, J. A. Piękoś, G. Licini, C. A. Hunter and C. Zonta, Angew. Chem., Int. Ed., 2021, 60, 23871–23877 CrossRef CAS PubMed.
-
(a) L. P. Wolters and F. M. Bickelhaupt, ChemistryOpen, 2012, 1, 96–105 CrossRef CAS PubMed;
(b) B. Pinter, N. Nagels, W. A. Herrebout and F. De Proft, Chem.–Eur. J., 2013, 19, 519–530 CrossRef CAS PubMed;
(c) C. W. Kellett, P. Kennepohl and C. P. Berlinguette, Nat. Commun., 2020, 11, 1–8 CrossRef PubMed;
(d) S. W. Robinson, C. L. Mustoe, N. G. White, A. Brown, A. L. Thompson, P. Kennepohl and P. D. Beer, J. Am. Chem. Soc., 2015, 137, 499–507 CrossRef CAS PubMed;
(e) H. Wang, W. Wang and W. J. Jin, Chem. Rev., 2016, 116, 5072–5104 CrossRef CAS PubMed;
(f) V. Oliveira, E. Kraka and D. Cremer, Phys. Chem. Chem. Phys., 2016, 18, 33031–33046 RSC;
(g) J. Thirman, E. Engelage, S. M. Huber and M. Head-Gordon, Phys. Chem. Chem. Phys., 2018, 20, 905–915 RSC;
(h) C. Loy, J. M. Holthoff, R. Weiss, S. M. Huber and S. V. Rosokha, Chem. Sci., 2021, 12, 8246–8251 RSC.
-
(a) J. S. Murray, P. Lane and P. Politzer, J. Mol. Model., 2009, 15, 723–729 CrossRef CAS PubMed;
(b) P. Politzer, J. S. Murray and T. Clark, Phys. Chem. Chem. Phys., 2013, 15, 11178–11189 RSC;
(c) G. Cavallo, P. Metrangolo, R. Milani, T. Pilati, A. Priimagi, G. Resnati and G. Terraneo, Chem. Rev., 2016, 116, 2478–2601 CrossRef CAS PubMed.
-
(a) A. M. S. Riel, M. J. Jessop, D. A. Decato, C. J. Massena, V. R. Nascimento and O. B. Berryman, Acta Crystallogr., Sect. B: Struct. Sci., Cryst. Eng. Mater., 2017, 73, 203–209 CrossRef CAS PubMed;
(b) C. C. Robertson, R. N. Perutz, L. Brammer and C. A. Hunter, Chem. Sci., 2014, 5, 4179–4183 RSC;
(c) M. G. Chudzinski and M. S. Taylor, J. Organ. Chem., 2012, 77, 3483–3491 CrossRef CAS PubMed.
- C. J. Massena, N. B. Wageling, D. A. Decato, E. Martin Rodriguez, A. M. Rose and O. B. Berryman, Angew. Chem., 2016, 128, 12586–12590 CrossRef.
-
(a) G. Berger, P. Frangville and F. Meyer, Chem. Commun., 2020, 56, 4970–4981 RSC;
(b) J. Pancholi and P. D. Beer, Coord. Chem. Rev., 2020, 416, 213281 CrossRef CAS.
-
(a) C. J. Massena, A. M. S. Riel, G. F. Neuhaus, D. A. Decato and O. B. Berryman, Chem. Commun., 2015, 51, 1417–1420 RSC;
(b) A. M. S. Riel, D. A. Decato, J. Sun, C. J. Massena, M. J. Jessop and O. B. Berryman, Chem. Sci., 2018, 9, 5828–5836 RSC;
(c) J. Sun, A. M. S. Riel and O. B. Berryman, New J. Chem., 2018, 42, 10489–10492 RSC.
-
(a) A. M. S. Riel, D. A. Decato, J. Sun and O. B. Berryman, Chem. Commun., 2022, 58, 1378–1381 RSC;
(b) R. L. Sutar and S. M. Huber, ACS Catal., 2019, 9, 9622–9639 CrossRef CAS;
(c) D. Bulfield and S. M. Huber, Chem.–Eur. J., 2016, 22, 14434–14450 CrossRef CAS PubMed.
- A. V. Jentzsch and S. Matile, Top. Curr. Chem., 2014, 205–239 CrossRef PubMed.
-
(a) I. Alkorta, G. Sánchez-Sanz and J. Elguero, CrystEngComm, 2013, 15, 3178–3186 RSC;
(b) M. Solimannejad, M. Malekani and I. Alkorta, J. Phys. Chem. A, 2013, 117, 5551–5557 CrossRef CAS PubMed;
(c) B. Nepal and S. Scheiner, J. Phys. Chem. A, 2015, 119, 13064–13073 CrossRef CAS PubMed;
(d) M. Domagała and M. Palusiak, Comput. Theor. Chem., 2014, 1027, 173–178 CrossRef;
(e) S. T. Nguyen, T. L. Ellington, K. E. Allen, J. D. Gorden, A. L. Rheingold, G. S. Tschumper, N. I. Hammer and D. L. Watkins, Cryst. Growth Des., 2018, 18, 3244–3254 CrossRef CAS;
(f) F. Adasme-Carreno, C. Munoz-Gutierrez and J. H. Alzate-Morales, RSC Adv., 2016, 6, 61837–61847 RSC.
- M. G. Sarwar, B. Dragisic, L. J. Salsberg, C. Gouliaras and M. S. Taylor, J. Am. Chem. Soc., 2010, 132, 1646–1653 CrossRef CAS PubMed.
- O. Dumele, D. Wu, N. Trapp, N. Goroff and F. Diederich, Org. Lett., 2014, 16, 4722–4725 CrossRef CAS PubMed.
- A.-C. C. Carlsson, K. Mehmeti, M. Uhrbom, A. Karim, M. Bedin, R. Puttreddy, R. Kleinmaier, A. A. Neverov, B. Nekoueishahraki and M. Erdélyi, J. Am. Chem. Soc., 2016, 138, 9853–9863 CrossRef CAS PubMed.
- V. Stilinović, G. Horvat, T. Hrenar, V. Nemec and D. Cinčić, Chem.–Eur. J., 2017, 23, 5244–5257 CrossRef PubMed.
- Y.-P. Chang, T. Tang, J. R. Jagannathan, N. Hirbawi, S. Sun, J. Brown and A. K. Franz, Org. Lett., 2020, 22, 6647–6652 CrossRef CAS PubMed.
-
(a) A. M. S. Riel, R. K. Rowe, E. N. Ho, A.-C. C. Carlsson, A. K. Rappé, O. B. Berryman and P. S. Ho, Acc. Chem. Res., 2019, 52, 2870–2880 CrossRef CAS PubMed;
(b) M. R. Scholfield, M. C. Ford, A.-C. C. Carlsson, H. Butta, R. A. Mehl and P. S. Ho, Biochemistry, 2017, 56, 2794–2802 CrossRef CAS PubMed;
(c) A.-C. C. Carlsson, M. R. Scholfield, R. K. Rowe, M. C. Ford, A. T. Alexander, R. A. Mehl and P. S. Ho, Biochemistry, 2018, 57, 4135–4147 CrossRef CAS PubMed;
(d) R. K. Rowe and P. S. Ho, Acta Crystallogr., Sect. B: Struct. Sci., Cryst. Eng. Mater., 2017, 73, 255–264 CrossRef CAS PubMed.
-
(a) D. A. Decato, A. M. S. Riel, J. H. May, V. S. Bryantsev and O. B. Berryman, Angew. Chem., Int. Ed., 2021, 60, 3685–3692 CrossRef CAS PubMed;
(b) S. Portela and I. Fernández, Molecules, 2021, 26, 1885 CrossRef CAS PubMed;
(c) S. Scheiner, J. Phys. Chem. A, 2022, 126(51), 9691–9698 CrossRef CAS PubMed;
(d) R. P. Orenha, S. S. P. Furtado, G. F. Caramori, M. J. Piotrowski, A. Muñoz-Castro and R. L. T. Parreira, New J. Chem., 2023, 47, 4439–4447 RSC.
-
(a) L. Brammer, E. A. Bruton and P. Sherwood, Cryst. Growth Des., 2001, 1, 277–290 CrossRef CAS;
(b) Y.-Y. Zhu, H.-P. Yi, C. Li, X.-K. Jiang and Z.-T. Li, Cryst. Growth Des., 2008, 8, 1294–1300 CrossRef CAS;
(c) J. Shang, N. M. Gallagher, F. Bie, Q. Li, Y. Che, Y. Wang and H. Jiang, J. Org. Chem., 2014, 79, 5134–5144 CrossRef CAS PubMed;
(d) Y.-H. Liu, X.-N. Xu, X. Zhao and Z.-T. Li, Supramol. Chem., 2015, 27, 310–320 CrossRef CAS.
- J. Lapp and S. Scheiner, J. Phys. Chem. A, 2021, 125, 5069–5077 CrossRef CAS PubMed.
-
http://supramolecular.org
.
- J.-P. Gliese, S. H. Jungbauer and S. M. Huber, Chem. Commun., 2017, 53, 12052–12055 RSC.
-
(a) J. M. McGrath and M. D. Pluth, J. Org. Chem., 2014, 79, 11797–11801 CrossRef CAS PubMed;
(b) A. Docker, C. H. Guthrie, H. Kuhn and P. D. Beer, Angew. Chem., Int. Ed., 2021, 60, 21973–21978 CrossRef CAS PubMed;
(c) C. A. Hunter, C. M. Low, C. Rotger, J. G. Vinter and C. Zonta, Proc. Natl. Acad. Sci. U. S. A., 2002, 99, 4873–4876 CrossRef CAS PubMed;
(d) Z. Chen, B. Fimmel and F. Würthner, Org. Biomol. Chem., 2012, 10, 5845–5855 RSC;
(e) S. E. Wheeler, A. J. McNeil, P. Müller, T. M. Swager and K. Houk, J. Am. Chem. Soc., 2010, 132, 3304–3311 CrossRef CAS PubMed;
(f) J. Hwang, P. Li, W. R. Carroll, M. D. Smith, P. J. Pellechia and K. D. Shimizu, J. Am. Chem. Soc., 2014, 136, 14060–14067 CrossRef CAS PubMed.
-
(a) S. E. Wheeler and K. Houk, J. Chem. Theory Comput., 2009, 5, 2301–2312 CrossRef CAS PubMed;
(b) S. E. Wheeler and K. Houk, J. Am. Chem. Soc., 2008, 130, 10854–10855 CrossRef CAS PubMed.
- D. O. Soloviev, F. E. Hanna, M. C. Misuraca and C. A. Hunter, Chem. Sci., 2022, 13, 11863–11868 RSC.
- Q. Teng, P. S. Ng, J. N. Leung and H. V. Huynh, Chem.–Eur. J., 2019, 25(61), 13956–13963 CrossRef CAS PubMed.
- W. P. Ozimiński and J. C. Dobrowolski, J. Phys. Org. Chem., 2009, 22, 769–778 CrossRef.
- S. Alvarez, Dalton Trans., 2013, 42, 8617–8636 RSC.
- G. R. Desiraju and R. Parthasarathy, J. Am. Chem. Soc., 1989, 111, 8725–8726 CrossRef CAS.
Footnotes |
† Electronic supplementary information (ESI) available: Gas-phase DFT calculations and coordinates, NMR spectroscopic data, crystallographic refinement details. CCDC 2023091–2023093 and 2238833–2238840. For ESI and crystallographic data in CIF or other electronic format see DOI: https://doi.org/10.1039/d3sc02348f |
‡ No clear trend was observed among R1-G3XB derivatives. The amine proton peak is 5.53 ppm for R1 CF3, 5.25 ppm R1 Cl, 5.17 ppm R1 F, 5.40 ppm R1 H and 5.31 ppm R1 = Me. The deviation for the fluorine and chlorine substituents might be a result of their π electron donating property affecting the amine. We rationalize the trend observed of 2R2-G3XB derivatives based on how the substituents influence the electronics on the HB acceptor (in this case the XB donor) rather than the HB donor. |
§ This finding also correlates with computational data from Scheiner26 illustrating that the through bond influence of the electron withdrawing group on the XB properties diminishes with its distance from the halogen atom. |
¶ Comparing the entire 2R2-G3XB series, we noted that the Vs,max of 2Me-G3XB is smaller than 2H-G3XB which deviates from the expected electronic trend. This is likely due to small differences in the planarity of the structures during the calculations that affect the electronic environment of the halogens (see ESI†). For 2R2-G3XB derivatives which have more electron donating substituents (R2 F, H, Me, tBu), it was observed that the iodine in the S conformation which accepts a HB has a 0.1 to 2.0 kcal mol−1 higher Vs,max than the iodines in the bidentate conformation (which also accept HBs). We hypothesized that the S conformation provides more flexibility for the only N–H⋯I HB thus producing a stronger HB. However, when considering receptor design, this small difference would likely be compensated by allowing two convergent XBs to be available in the bidentate conformation. |
|
This journal is © The Royal Society of Chemistry 2023 |
Click here to see how this site uses Cookies. View our privacy policy here.