DOI:
10.1039/D3SD00194F
(Tutorial Review)
Sens. Diagn., 2023,
2, 1414-1436
The technology of wearable flexible textile-based strain sensors for monitoring multiple human motions: construction, patterning and performance
Received
23rd July 2023
, Accepted 14th August 2023
First published on 28th August 2023
Abstract
This paper discusses the development of wearable flexible textile-based strain sensors for monitoring multiple human motions. With the growing interest in wearable technology for health and wellness, these sensors offer a non-invasive and comfortable solution for tracking movements during daily activities. The paper outlines the technology behind the sensors, including their fabrication, material selection, and sensing mechanisms. It also discusses the potential applications of these sensors in various fields such as sports, rehabilitation, and medical monitoring. The results of several studies on the performance of these sensors are presented, including their accuracy and durability. Overall, the paper highlights the potential of wearable flexible textile-based strain sensors as valuable tools for monitoring human motions and improving health outcomes. However, overall while there are many obstacles such as accuracy and reliability, calibration, durability, interference, cost, and compatibility to the widespread adoption of textile-based strain sensors, researchers and engineers are working hard to overcome these challenges and realize the full potential of this exciting technology.
1. Introduction
Wearable technology has become increasingly popular in recent years due to its ability to provide real-time monitoring of various physiological and environmental parameters. Among various types of wearable sensors, textile-based strain sensors have gained significant attention due to their high sensitivity, flexibility, and stretchability. These sensors can be seamlessly integrated into various types of clothing and can provide accurate monitoring of multiple human motions such as breathing, body movement, and muscle activity. With advances in materials science and fabrication techniques, these sensors have shown tremendous potential for healthcare, sports, and rehabilitation applications. In this context, this technology review will discuss the recent developments in the field of wearable flexible textile-based strain sensors for monitoring multiple human motions. Sensors embedded in fabrics are discussed here. Textile-based detectors can be identified through their distinctive textile design which is consistently composed of textile materials. The textile framework has four sections. Several technologies may be used to produce textile sensors. Mechanical, chemical and thermal effects in sensors embedded in textiles must not compromise the sensing function. Therefore, it is reasonable to use various approaches for sensors which can depend upon various chemical, physical and thermal reactions to stimuli.1 In the early stages, commercial thin film electronic components were simply sewn onto textile substrates to create useful textiles with sensory capabilities.2 Piezoresistive, capacitive, piezoelectric and other physical transduction principles can be utilized to categorize pressure sensors in general. Capacitive sensors stand out in this regard due to their quick dynamic response, modest power consumption and low detection limits. They have proven ability to track a diversity of human physiologies including finger touching, blood pulse monitoring and muscular activity. Despite these capabilities, conventional capacitive sensors have drawbacks because of their unwieldy design and reduced sensitivity in low-pressure ranges.3 The benefit of capacitive technologies is that they enable object distance detection in low-light situations.4 A current generation of capacitive strain sensors that recognize strains have outstanding durability and continue to function even after tens of thousands of cycles.5 Due to their straightforward designs and great sensitivity, piezoresistive materials have demonstrable qualities for the beneficial improvement of strain detectors. However, there is an urgent need to minimize the cross-sensitivity and vulnerability of piezoresistive sensors to hostile conditions, such as high temperatures and humidity. Inorganic piezoelectric materials and piezoelectric polymers are two different categories of piezoelectric materials. Currently, the two piezoelectric materials most often utilized allow a high level of intrinsic piezoelectric properties.6,7 For applications in structural health monitoring, the bending and piezoelectric capabilities have shown considerable promise in providing flexible reinforcement in composite materials.8 Due to their highly sensitive, technical adaptability, piezoelectric haptic detectors composed of polyvinylidene fluoride (PVDF), offering numerous techniques, compatibility, reliability and low cost, are employed for measuring movements.9 Piezoelectric pressure devices are types of autonomous device systems using piezoelectric phenomena that can produce electronic pulses when pressure is applied.10 By controlling the compositions of such materials, assembly structure, surface shape and other factors, it has proved feasible to improve the turboelectric pressure sensor's performance.11 Polymer films in textiles are frequently employed as substrates for flexible wearable sensors due to their flexibility, scalability, lightness and low-cost benefits. Conductive polymer composites have been created using a variety of manufacturing methods that include conductive additives like copper, aluminium, graphite, carbon nanotubes, carbon fibres, and graphene.12,13 Wearable sensors for health monitoring depend on the physiological, biochemical, and biological information that is transmitted through the human skin. For instance, the components of human sweat, such as glucose, lactate, and urea, are valuable indicators for physiological measures (Table 1).14 In wearable technology, textile materials including fabrics, yarns and fibres are regarded as viable modern alternatives to silicon chips. Recently, electronic textiles (e-textiles), or smart fabrics, often referred to as perceptive substances and structures, also respond to external stimuli and are often considered fashionable. These clothes are modern and sophisticated products or systems which can perceive and react predictably to their surroundings and measure such parameters as strain, pressure, temperature, and humidity.15 In this report, classification of strain sensors and textile-based strain sensors, their fabrication, process, structure, characterization, merits, demerits and applications are discussed in depth. The text concludes with a description of challenges and future continuous improvement.
Table 1 Summary of findings from recent research papers, based on resistive-type sensors
Types of materials |
Strain sensitivity |
GF |
Response time |
Modulus |
Merits |
Application |
Ref. |
Silicone elastomer/MWCNTs |
High |
100% elongation −4.9 |
1 ms |
100% 46 kPa |
Feasible for enhancing electrical properties |
Disease evaluation in actual time as well as allowing ongoing medical supervision |
14
|
PB/AgNF-PU |
High |
32.08 |
— |
— |
Excellent mechanical and electrical reversibility, 30% strain |
Monitoring human detection during movement such as sitting, moving around, and running |
29
|
mNW/elastomer composite |
High |
GF: ≈81 at >130% strain |
Response too large |
— |
The fabrication technique is straightforward, economical as well as repeatable |
A variety of human actions, including significant joint bending, breathing, blinking, open and closing of the mouth and drinking |
23
|
MXenes |
Sensing range up to 70% |
— |
— |
— |
— |
Smart wearable devices |
20
|
Graphite nanoflake/rebound silicone elastomer |
Large strain sensitivity |
96.73 |
160 ms |
— |
Significant longevity with extreme linearity |
Human joint movements, biomedical engineering |
21
|
Gelatine/DATNFC/Fe3+ hydrogel GDIH |
— |
2.24 |
— |
1.14 kPa−1 |
Long-lasting self-healing capabilities, ecological degradation, and full recyclable status |
Healthcare monitoring |
22
|
2. Taxonomy of stretchable and wearable strain sensors and their performance analysis
Electronic skin and flexible wearable technology have advanced in recent years as a result of them finding extensive applications in human detection therapy, intelligent robotics, and health monitoring. The most recent developments have been in piezoelectricity, piezo resistivity, capacitance, and triboelectricity for e-skin-based flexible pressure sensors.16 Concurrently, the most extensively studied highly stretchable strain sensors are those made of resistive, capacitive, piezoelectric, turboelectric, and optical materials.17 With flexible sensors for electronic, healthcare, textiles, skin-related, electronic control technologies, frictional touch, capacitance contact, turbo-electric contact and piezoelectric tap, the technology is advancing markedly. Researchers worldwide are focusing on these technologies to deliver components for flexible wearable sensors.18 Wearable multifunctional sensing technology is a crucial tool for keeping track of human health since it allows for flexibility and stretchability in the sensors. To attain high sensitivity, several innovative architectures and manufacturing techniques are being explored.19 E-textile detectors that utilize a range of resistive, capacitive and inductive sensing techniques have been developed as a result of improvements in device materials and their construction. To retain appropriate performance under large and repetitive deformations, these sensors must mix materials with radically different mechanical characteristics, electrical behaviour, and processing capabilities (Table 1).20
2.1 Understanding resistive strain sensors: advantages, limitations, and real-world applications
The early 1940s saw the creation of resistive-based strain detectors using metal foils. These first sensors could only measure strain over a maximum range of 0 to 5%. The drive to achieve portable electronics has changed how resistive-type strain sensors are built and resulted in them being readily available in a stretchable form rather than a brittle one (Table 1).21 These sensors are generally made of flexible substrates and electrically conductive sensing layers (Table 1).22 The most difficult strains to measure are those of high sensitivity and stretchability. Consequently, several research programmes are taking place to develop highly sensitive and stretchable sensors. According to Kim et al., the sensors exhibit both a high standard of sensitivity, high stretchability and long-term performance stability (Table 1).23 Resistive tactile sensors provide various benefits, including high sensitivity with a simple device structure, controllable spatial resolution and affordable manufacture.24 A thin-film resistive strain sensor with a scalable sensing sheet allows for the manufacture of low-cost, high-volume sensors having a minimal deviation of around 1.5.25 In ‘as-prepared’ flexible sensors, PMXene, CNTs and doped Ni ions interact to form successful 1D, and 2D double conductive with a notable specificity of 9.25 and a detection limit of 100% strain.26 The most important characterisation of strain sensors are gauge factors (GF) and response time. The gauge factor is defined as the ratio of the percentage change in electrical resistance of a material to the percentage change in strain or deformation that the material experiences. The gauge factor is an important parameter for the design and optimization of strain sensing devices, such as strain gauges, which are commonly used in applications ranging from structural health monitoring to biomedical sensing. In the context of sensors, response time refers to the time it takes for a sensor to detect and respond to a change in the physical quantity it is designed to measure. However, sensors displaying resistive behaviour based on eco-flex silicone and multi-walled carbon nanotubes have been described in a recent article. The proposed sensor exhibits very sensitive properties, including gauge factors of −4.9 at 100% elongation, −0.9% at 90 degrees of bending, 725% stretchability, 0.7 MPa tensile strength, and 46 kPa at 100% modulus of elasticity.27 Elastomeric fibre-based strain sensors that incorporate graphene (Gr) and carbon nanotubes (CNTs) were created by Liu et al. to recreate the conductive connections of the fibres, the solution etching technique was used to create a 1D@2D@1D hierarchical structure including pores and microcracks. This resulted in the fibres' capability of functioning over a very large strain range (0–500% – almost 31 times larger than that for the non-etched fibre) is shown in Fig. 1(a) and for extremely high gauge factors in the region of 1667. For up to 10
000 tensile strain cycles, the sensor exhibited exceptional stability. The fibre-based strain detector was also utilized to rapidly and precisely track biological motions including finger, elbow, and knee bending.28 As reported by Kim et al., rubber bands, silver nano particles and polyurethane PB/AgNF-PU which has excellent mechanical and electrical reversibility, 30% strain capability, high resistivity of 6328 S cm−1 and a gauge factor of 32.08 was employed. Strain sensors with exceptional sensing capabilities have several potential applications (Table 1).29 These include motion tracking, structural health and damage monitoring, smart robotics, man–machine interfaces, personal healthcare and other uses. The most crucial technologies for creating the textile-based strain sensor are capacitive and resistive systems.30–32 Another article discussed the development of a stretchable strain sensor using a combination of electrospinning and electrostatic spray deposition techniques. The sensor is made of a composite material consisting of MXene and thermoplastic polyurethane, which allows for both high sensitivity and stretchability. The fabrication process involves electrospinning the thermoplastic polyurethane onto a substrate, followed by electrostatic spray deposition of the MXene layer. The resulting sensor exhibits excellent mechanical properties, with a high sensitivity to strain and a wide range of detectable strains. The sensor also shows good stability and repeatability over multiple cycles of stretching and releasing Fig. 1(b). The authors suggest that this new approach to fabricating stretchable sensors could have applications in a variety of fields, including wearable electronics, robotics, and biomedical devices.33
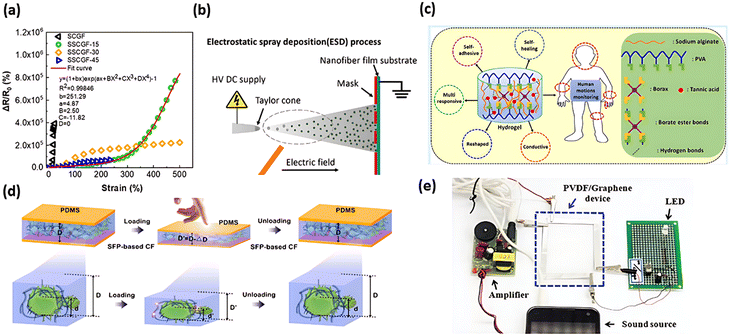 |
| Fig. 1 (a) A wide strain range (b) schematic diagram of electrostatic spray deposition (Copyright © 2023, Elsevier). (c) Graphene-based ultrasensitive strain sensors (Copyright © 2020, American Chemical Society). (d) Schematic of the pressure-induced empty shape of a sensor caused by microspheres of sunflower pollen (Copyright © 2019, Springer). (e) Flexible strain sensor using compound hydrogel (Copyright © 2021, American Chemical Society). | |
Silicone elastomer/graphite nano flake is a nano-composite strain detector using a rebounding elastic silicon layer and was developed by R. Madhavan et al. The sensor exhibited potential for accurate measurements of every human movement, including small mechanical deflections in the face, eyes, blood flow, throat, and large-strain movements of the wrist, elbow, and fingers.34 Based on the MMDI approach, the hydrogel-based sensors' as-fabricated showed excellent mechanical qualities, outstanding sensitivity, exceptional sensing stability, significant self-healing, and full recyclability.35 Zhou et al. proposed a method for making graphene or eco-flex compound materials and devices. The sensors produced exhibited extremely high sensitivity. They were able to recognize a variety of physiological signals, including active body movements, voice and pulse monitoring as well as utilizing cloud platforms to track human respiration in real-time, showing that complex motions and sign languages could be accurately identified.36
2.2 Overview of capacitive strain sensors with performance
Features of capacitive strain gauges include high sensitivity, high accuracy, and low drift. They can be used to measure both static and dynamic strain, and they are highly durable and reliable. In the sensor industry, flexible pressure sensors are considered by far the most important products when compared with other flexible devices.37 Capacitive flexible pressure sensors stand out among this category due to their uncomplicated device architecture, unique qualities such as energy efficiency and affordable manufacturing costs.38 There are several materials used for high sensitivity including barium titanate (BaTiO3), multi-walled carbon nanotubes (MWCNTs) and polydimethylsiloxane (PDMS). BaTiO3/MWCNTs/PDMS flexible capacitive pressure sensor demonstrated a rapid reaction time and a low detection limit (∼7 Pa).39 The first class of capacitive strain sensors with super-stretch capability and sensitivity have recently been developed by researchers. The sensors reached a 300% strain GF of 2.07 while demonstrating outstanding linear minimal distortion, and high mechanical durability over 1000 tensile cycles with rapid signalling.40 Zhao et al. were able to create highly stretchable, flexible hydrogel-based strain sensors that were wearable, self-adhesive, sensitive, demonstrated self-recovery and provided a wide range of possible responses. This strain sensor had a high gauge factor of 15.98 and could sustain up to 780% strain as well as monitor different human motions Fig. 1(c).41,42
2.3 Overview of piezoelectric strain sensors with performance
Piezoelectric-based strain sensors are commonly used in structural health monitoring of bridges, buildings, and aircraft, where they can detect small changes in strain that may indicate the onset of structural damage or failure. They are also used in vibration analysis, where they can measure the vibrations of rotating machinery and other mechanical systems. Piezoelectric materials are useful for quickly and accurately evaluating high-repetition dynamic signals and for converting external pressures into equivalent potential differences Fig. 1(d).43 Another statement made for them is that flexible piezoelectric sensors made of PVDF fibre arrays can be electro-spun before use. The most important sensor characteristics of sensors of this type are stretch ability, sensitivity, and tenability.44,45 In conclusion, piezoelectric-based strain sensors are a reliable and accurate method for measuring mechanical strain and deformation. Their high sensitivity and frequency response make them ideal for a wide range of applications, including structural health monitoring and vibration analysis.
2.4 Overview of piezoresistive based strain sensors with performance
Capacitive, piezoelectric, piezoresistive and optical sensors are the four basic detecting methods and are particularly important for strain sensing Fig. 1(e).46 Among them, piezoresistive sensors have generated considerable curiosity, owing to their advantages of production efficiency and simple data interpretation Fig. 2(a).47 Commonly, electronic textiles, sometimes referred to as smart textiles, denote unique products or technologies that are capable of perceiving and reacting to circumstances in an uncomplicated manner.48 Piezoresistive sensors had very little mechanical flexibility a decade ago as they were made of rigid, non-organic, and brittle materials.15 However, piezoresistive sensors recently achieved enhanced sensitivity, detection ranges, reaction times, and durability and achieved heightened performance for flexible pressure sensors after a substantial amount of research.49,50
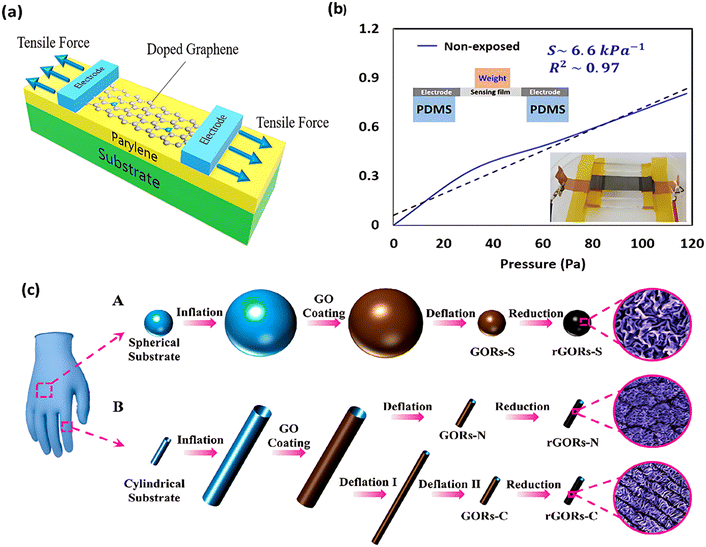 |
| Fig. 2 (a) Piezoelectric polymer-based sensor connected to a circuit (Copyright © 2016, Wiley), (b) parallel ultrasensitive and extremely flexible strain sensors based on micro cracks (Copyright © 2016, American Chemical Society), and (c) photographic recordings with illustrations (Copyright © 2019, American Chemical Society). | |
Advances in graphene-based flexible and wearable strain sensors have opened up a wide range of applications, including smart textiles for sports and healthcare, biomedical devices for monitoring and treating diseases, and human–machine interfaces for virtual and augmented reality. These sensors offer high sensitivity, flexibility, and durability, making them ideal for use in wearable and portable devices.51 The sensors demonstrated fast response times of less than 2 seconds, good stability over a long period, and selectivity towards different gases. Additionally, the sensitivity of the sensors was found to increase with higher concentrations of carbon nanofibers in the composite.52
2.5 Overview of optical-based strain sensor with performance
An optical sensor refers to a type of sensor that uses optical fibres to detect changes in strain or deformation in a material or structure. The sensor works by transmitting light through the fibre, which is sensitive to changes in the material or structure's deformation.53 There are some potential characteristics of the optical sensor such as multi-responsive, self-healing, air-stable, ionogel-based, and vertically integrated.54 Sagnac interferometer-based optical fibre strain sensors are a type of fibre optic sensors that utilize the Sagnac effect to measure strain or other physical quantities. These sensors have the advantage of high sensitivity, high accuracy, and immunity to electromagnetic interference Fig. 2(b).55 The sensor consists of a photonic crystal fibre with a small air cavity that acts as a strain sensor. This type of sensor has several advantages, including high sensitivity, fast response time, and the ability to measure strain in multiple directions. It has potential applications in fields such as structural health monitoring, aerospace engineering, and medical diagnostics.56 Functionalized fibre-based strain sensors are a type of optical strain sensor that utilizes specialized fibres coated with functional materials to detect changes in strain. Additionally, they offer several advantages over traditional strain sensors, including their ability to measure strain in multiple directions and their immunity to electromagnetic interference. Overall, functionalized fibre-based strain sensors represent a promising pathway to the development of next-generation wearable electronics.57
Overall, each type of sensor has its advantages and disadvantages. Resistive sensors are simple, inexpensive and offer a wide range of sensing applications. Capacitive sensors are sensitive and have a high signal-to-noise ratio. Piezoresistive sensors are highly sensitive and have a wide dynamic range. Piezoelectric sensors are highly sensitive, offer a fast response, and are suitable for high-frequency applications. Optical sensors are non-contact, offer high accuracy, and can be used in harsh environments. Resistive strain sensors work by changing their resistance as the material undergoes strain. Capacitive strain sensors use a change in capacitance due to deformation. Piezoresistive sensors use the resistance change of a material under stress. Piezoelectric sensors use the voltage generated by the deformation of a crystal or polymer. Optical strain sensors measure the change in the refractive index or light transmission through a material. The challenges in the development of strain sensors are improving sensitivity, stability, reliability, and reducing noise. Additionally, future challenges include the development of sensors with smaller sizes, lower power consumption, and the ability to withstand harsh conditions. Moreover, integration with wireless communication systems and the Internet of Things (IoT) will lead to significant advancements in the field of strain sensors representing a promising pathway to the development of next-generation wearable electronics.
3. Overview of textile based flexible conductive materials with consideration of construction, patterning and performance
Globally, the textile industry is taking a proactive approach to developing new and innovative textile systems that meet the technological needs of modern society in the 21st century. This forward-thinking approach is aimed at creating conceptual textiles that are both innovative and practical Fig. 2(c).58 The new approach to fabricating flexible conductive materials could have applications in a variety of fields, including wearable electronics, robotics, and biomedical devices.59 The processing of the material requires specific treatments such as chemical processes and external metallization using metals like silver (Ag), copper (Cu), aluminium, nickel, or silver.60 Up to the present, many different methods have been suggested to create productive textiles, including coating or printing polymer materials and colourants on surfaces, hand loom and knitting conductive yarns through into textile, stitching and embroidering loops on exteriors, ultrasonic cleaning metal deposition, integrating metal wires into a fabric and others.61 Due to identifying several applications in interface design for machines, strain devices, pressure sensors, temperature monitoring, moisture sensors, etc. are among numerous examples of flexible sensing devices based on sensing the various inputs from objects and surroundings. The application of homogeneous parallel microcrack thin films in micro- and nano fabrication, biology, photonics and electronics has also become possible. A variety of conductive substances, such as carbon nanotubes, graphene, silver nanoparticles and nanowires, organic polymers, transition metal complexes etc. make up the active components.15,62–64 Different processes have been investigated to adjust the strain detectors' resistivity to exterior strains, including the consideration of geometric effect, unplugging of nearby components, tunnelling impact and most significantly, understanding the development of fracture in nanoparticles. Composites made of carbon nanotubes, nanowires, graphene, and hybrid materials and structures have proven to be very flexible strain sensors.65 In particular, polymer matrix functional features of CNT-reinforced nano composites can be provided by improving properties, which are mostly imparted by the CNT fillers.66
The technology needs to preserve the connection of conductive pathways in a large strain zone to prevent a substantial radical transformation at low strains.67 Therefore, a variety of strain sensing materials have been investigated for advanced strain sensors with stretchability, including silicon nano membrane/polymers (Eco-ex), ZnO nanowire/polystyrene nanofiber hybrid structures, carbon nanotube/polymers (PDMS), carbon black/thermoplastic elastomers, graphene/epoxy and carbon black/thermoplastic elastomers.68 Although carbon nanotubes and graphene composites may be used in strain sensing applications where there is low loading, their high price, and lack of large-scale industrial manufacturing restrict their use when compared with conductive carbon black (CB), which is more affordable.69 Two-dimensional (2D) substance graphene has outstanding chemical properties and optimal electric and mechanical properties for e-textiles. Researchers have recently experimented with a variety of approaches to create complicated buckling patterns in the material. Recently, there has been rapid advancement in the fabrication of stretchable or elastic strain-sensing properties in nanotube and nano wire polymer structures Fig. 3(a) and (b).70–75
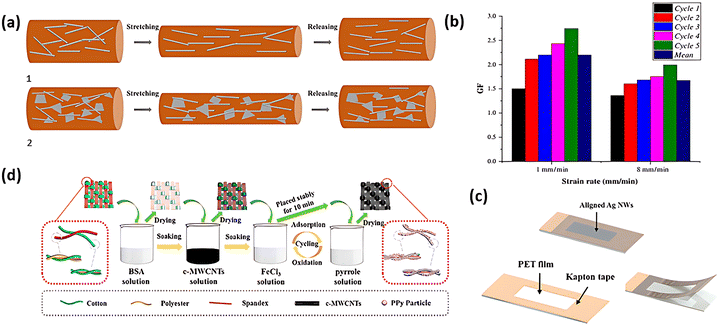 |
| Fig. 3 Diagram showing the development of (a) (1) S-AgNW/PU and (2) applied cyclic load testing, S–AgMix/PU fibres (Copyright © 2018, American Chemical Society). (b) A comparison of GF readings for the PDMS2 detector following repeated cyclic loading using two separate applied loads (copyright@2020, from Wiley). (c) Diagrams of the DEF/c-MWCNTs/PPy strain sensor production process (Copyright © 2018, American Chemical Society) (d) employing Kapton tape to create a design on a polyethene terephthalate (PET) film (copyright@2020, MDPI). | |
3.1 Metallic materials construction, patterning with performance
Metal-based strain sensors are devices that detect and measure the deformation or strain of a metallic material when it is subjected to external forces or loads. These sensors are widely used in a variety of industrial applications, such as structural health monitoring, aerospace, automotive, and biomedical engineering. Characterization of metal-based strain sensors is a critical step in understanding their performance, reliability, and sensitivity.76 The characterization process involves measuring the electrical and mechanical properties of the sensor, such as resistance, sensitivity, linearity, hysteresis, and stability. These properties are influenced by several factors, including the metallic material used, the sensor geometry, the manufacturing process, and the applied strain.77 Another important aspect of characterization is determining the sensor's linearity, which refers to the relationship between the applied strain and the corresponding change in resistance. A linear response is desirable because it simplifies the calibration process and ensures accurate measurement.78 Hysteresis is another factor that needs to be characterized. Hysteresis refers to the difference in the sensor's output when the strain is applied and removed. This effect can cause inaccuracies in the measurement and needs to be accounted for during calibration Fig. 3(c).79 Flexibility is needed for effective sensing and metal is often used for manufacturing sensing devices. Metals such as silver, gold, base metals, aluminium and zinc are used to manufacture metallic films.80 The best conductive material is silver; it has special qualities in respect of high conductivity and low electrical conductivity, stretchability, durability and use over wide temperature ranges (Table 2).81 Their advantages include flexibility and light transmittance.82 Gold nanowires are expensive, while copper nanowires are quickly oxidized. Consequently, silver nanowires are generally used. They have high conductivity and antibacterial properties.83 Unsurprisingly, due to its remarkable properties and applications, the best metallic material to be used in sensing so far has been silver nanowire (Ag NW).84 A maximum AgNW mass fraction fibre with a 14
205 S cm−1 electrical conductivity, exceptional stretchability and the ability to be stretched up to 200% was presented by Lu et al. A solvent/non-solvent wet-spinning process was used to create conductive composite fibres.85 Hashemi, et al. experimented with polymer matrices of thermoplastic polyurethane (TPU), polydimethylsiloxane (PDMS) and conductive nanomaterials of silver nanowires (AgNWs) were generated based on strain sensors using the solvent/non-solvent wet-spinning process. The resistivity and stability of the TPU-based sensors were superior to those based on PDMS.86 Liu et al. (2022) indicated that a cellulose nano fibril/silver nanowire (CNF/AgNW) nano paper, was made by a staged process (SBS) self-assembly method.87 Further new uses included wearable devices, smart clothes and other applications. With potential in electrical and chemical studies and numerous other research disciplines, micro- and nanomaterial ion-sensitive metal oxides (MOx) have attracted a lot of attention. In this regard, Manjakkal et al. presented a detailed examination of the wide-ranging array of MOx materials used to make pH sensors using various fabrication techniques Fig. 3(d).88 Yang et al. developed a technique for producing AgNW where designs were created using a special three-dimensional mask and a vacuum filtering mechanism. The characterization of metal-based strain sensors involves measuring various electrical and mechanical properties to understand their performance, reliability, and sensitivity. The characterization process helps ensure that the sensor meets the required specifications for the intended application and provides accurate and reliable measurements over time Fig. 4(a).89 Sensors implemented using this method were capable of measuring high strains and sensitivities.90 According to Choi et al., strain sensors were constructed using the temperature-controlled dip coating method and employing Kapton tape to create designs on a polyethene terephthalate (PET) film. They provided advantages for commercialization because they can be produced at a minimal cost in huge volumes (Table 2).91 It is noteworthy that the AgNW-based strain sensors have a planar thin film geometry previously reported by Kong et al. who developed a cost-efficient coating plus rolling-up technique to manufacture an AgNW/PDMS-based fibrous strain sensors. The 100% strain detection response was remarkably linear at a GF of 3.0. Also, excellent durability was demonstrated by the fibre strain sensor (Table 2).92 Yu et al. using AgNws demonstrated a superior electromagnetic interference filtering capability over AgNPs whenever the SE was <20 dB (>99%) at all frequency ranges between 3 and 17 GHz. This is crucial for the possibility of silver-based materials increasing electrical properties as well as electromagnetic interference (EMI).93 Liu et al. presented a flexible strain sensor constructed from carbon nanotubes. The strain sensor can measure hand movements. A glove can recognize a patient's finger bending and display the user's responses properly on a computer or a mobile device APP.
Table 2 A summary of recent research into the performance of metal-based strain sensors
Materials |
Fabrication method |
Stretchability |
Sensitivity (GF) |
Other properties |
Ref. |
AgNW and CNT/PDMS |
Coating with a sprayer spin coating |
50% |
6.7 |
Results can be demonstrated with an APP on a smartphone or a central computer after detecting the wearer's finger bend |
92
|
AgNW/PDMS |
Spray coating plus rolling |
100% |
3 |
Durable for 6000 cycles. Excellent reliability, and outstanding linearity |
91
|
AgNW/PDMS |
Dip coating |
60% |
89.99 |
Produced at low cost in large quantities, relatively low-cost materials, and flexibility |
90
|
AgNW and graphene/PDMS |
Drop coating |
60% |
9156 |
Resistance to electricity; the electrical conductivity of 1.2 10 2 U cm was outstanding |
81
|
AgNW/TPU |
Spray coating |
100% |
4.4 × 107 |
Exhibited high sensitivity and was applied to human body motion monitoring |
93
|
AgNW and MXene/a self-healing elastomer |
Spray |
96% |
>29.4 |
Pressure sensing ability |
94
|
AuNPs/tetra ethylene glycol dithiol |
Seeded growth method |
— |
≈126 |
Outstanding sensing characteristics include a wide dynamic range, a rapid response time (16.1 ms), and high cycle reliability |
96
|
Gold nanoparticles/PDMS |
Stop-and-go convective self-assembly |
21% |
— |
These single-wire strain gauges have shown remarkable sensitivity, reproducibility, and resilience |
97
|
Polyethene terephthalate/palladium nanoparticle |
— |
— |
Up to 1000 |
Withstand the long-term bending–unbending cyclic test with heightened performance |
98
|
Super aligned carbon nanotubes SACNT/platinum (Pt) |
— |
ε = 100% |
≈12 274 |
Superb mechanical flexibility, long-term operation, a relatively quick response time (257.7 ms) |
99
|
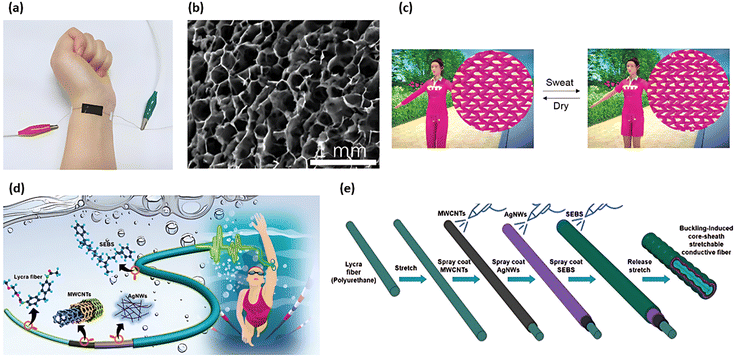 |
| Fig. 4 (a) A cross-section of NTSm@rubber@fiber is schematically illustrated and it depicts a buckling profile throughout both exhaling and inhaling (Copyright@2020, from Wiley). (b) Wrist pulse indication (Copyright © 2020, American Chemical Society) (c) biological tissue and STFMs with self-healing properties are shown schematically (Copyright@2022, from Wiley). (d) The CSCF's framework (Copyright © 2020, Wiley), (e) the fabrication process for CSCF (Copyright © 2020, Wiley). | |
The device is wireless and usually completely flexible (Table 2).94
Another research group identified a simple technique for constructing electrospun thermoplastic polyurethane (TPU) based flexible strain detectors linking silver nanowires (AgNWs) with a manufacturing technique using rGO/AgNWs/TPU strain sensors which demonstrated great strain sensitivity and achieved exceptional results under maximum deformation (Table 2).95 Moreover, Zhang et al. fabricated an alternative simple material with a novel multiscale conductive layer structure by merely applying conducting chemicals to the elastomer with dynamic Diels–Alder bonding. The developed sensor was capable of concurrently accomplishing extreme sensitivity (sensitivity range of 0.5% to 96%), Additionally, the sensors demonstrated stable responses to a range of frequencies, strains, and long-term stretching (Table 2).96 A gold-based strain sensor is a type of pressure sensor that uses ultrathin gold nanowires to measure mechanical strain. The sensor can be designed as a wearable device that is highly sensitive to pressure changes and can be used in a variety of applications, including biomedical monitoring, electronic skin, and robotics.97 The sensor is made up of a network of gold nanoparticles linked together by molecules that act as “stretchable bridges”. The gold strain sensor offers high sensitivity and resolution, making it a promising material for applications in healthcare and wearable devices (Table 2).98 Overall, metal-based strain sensors are versatile and reliable devices that play an important role in monitoring and controlling the behaviour of mechanical systems. With advancements in materials science and electronics, these sensors are becoming more sensitive, durable, and cost-effective, making them an attractive option for a wide range of applications. The method involves using a stop-and-go convective self-assembly process to create a single wire of nanoparticles. The resulting strain gauge was found to have high sensitivity to strain, making it a promising tool for various sensing applications (Table 2).99
The sensor's resistance changes with applied strain due to the movement of the nanoparticles, and the sensitivity can be adjusted by varying the size and concentration of the nanoparticles. The Mott variable-range hopping regime enables the sensor to operate over a wide range of temperatures and strain rates. The sensor has potential applications in areas such as structural health monitoring and wearable electronics (Table 2), and Fig. 4(b).100 The sensor's resistance changes with applied strain due to the movement of the nanoparticles, and the sensitivity can be adjusted by varying the size and concentration of the nanoparticles. The Mott variable-range hopping regime enables the sensor to operate over a wide range of temperatures and strain rates. The sensor has potential applications in areas such as structural health monitoring and wearable electronics (Table 2).101
3.2 Polymer-based strain sensor construction, patterning and their performance
In the discipline of sensing, polymer-based components are the most critical for achieving improved performance. These materials exhibit biocompatibility, biodegradability, flexibility and other positive qualities.102 Both natural and artificial, these two polymer-based materials are easily accessible. Animals and plants can produce natural polymers that perform in numerous sectors, for example in treating common ailments, pharmaceutical industries, cosmetology and science.103 Conventional strain sensors to date have had limited sensitivity, detecting ranges, and stability. However, Seyedin et al. revealed that highly conductive polyurethane/PEDOT PSS composite fibres may withstand up to 160% strain during construction.104 According to Eom et al., the PEDOT/PS fibre shows the simple integration of textile-based multifunctional sensors and UI devices into a wireless system that can communicate using specified hand movements to represent American Sign Language. Strain sensors, body-motion tracking sensors, and contact sensors can all be categorised as strain devices (Table 3).105 Shen et al. used incorporated carboxylic-multi-walled carbon nanotubes and adsorbent pyrrole procedures to build a stretchable biaxial strain sensor to perform actual tracking of complicated human body movements. This method has exceptional performance, showing excellent stretchability of over 80% and a rapid reaction time of 100 s (Table 3).106 Due to their inherent conductivity, there is a lot of research being done on regulating polymers in the fields of catalysis, sensors, actuators, capacitors and compounds, etc. The encouraging outcome for tests on the material demonstrated an increased strain limit of 309.5%, a tensile strength of 32.8 MPa, and outstanding sensitivity. The sensor can identify a variety of physiological signals. Depicts the sensor put on a healthy wrist of a 23-year-old female of 161 cm height and this allowed non-invasive pulse tracking, heartbeat and vocal cord vibrations to be measured (Table 3).107 The construction of a continuous cPPy/BCNF active layer utilizing customized BCNF to create NR latex-based sensing material represents the most exciting innovation discussed in this study. The consequent interest led to Xu et al. conducting research that produced a natural rubber (NR)-based sensing system with a highly configurable and responsive polypyrrole-redesign bacterial cellulose nanocomposite (cPPy/BCNF) construction. Outstanding sensitivity and long-term dependability are features of this strain sensor.108 Another way to use materials based on polymers employs a polyaniline PANI/reduced graphene oxide rGO/polydopamine PDA/lycra cotton LC strain sensor, which was fabricated by Jin et al. by dip coating and polymerization. The GF of the strain sensor in the wide strain range was (0.2–50%). The material exhibited excellent sensitivity, stability, stretchability and durability. This technology provides sensing that can track both physical human movements and medical parameters (Table 3).109 Sensing structures were created by Rashida et al. The structures showed exceptional mechanical resilience, extended fatigue life under various loading and repeatable piezoresistive behaviour for both subtle and obvious body motions. The washability, wearability, as well as other textile-related properties that have been evaluated for use in real-world situations, are reflected in this study's publications.110 Mechanically strong, high-conductivity strain detectors that can operate under different circumstances, offering outstanding repeatability and quick reaction times are in high demand. In another study, Lam et al. produced strain sensors which showed high stretchability, a wide sensing range and high linearity of 1000%, a rapid reaction time of 63 ms and 0.05% for the full resolution.
Table 3 Summary of polymer-based strain sensors' performance
Materials |
Method/process |
Sensitivity |
Stretchability |
Advantage with application |
Ref. |
PEDOT/PS |
— |
— |
3% of strain |
Biaxial strain sensors, skin tracking detectors, touch devices, or identical stress–strain characteristics |
105
|
MWCNTs/PPy |
Scalable soaking and adsorption–oxidizing |
5.2 |
The good stretchability of over 80% |
Movements of the human skin's joint capsule, such as those of the shoulder and neck |
106
|
PPy@PVA |
Cross-linking |
5.07 |
309.5% |
Physiological signals, detecting pulse beats |
107
|
PANI/rGO/PDA/LC |
Dip coating |
24 |
50% |
Excellent mechanical durability application: actual human activity information and both computer and healthcare tracking |
109
|
PEDOT:PSS with the CNTs |
Using elastomer |
— |
Sensing range of 1275% |
Application in smart clothing |
111
|
PEDOT:PSS/dimethyl sulfoxide |
Charge transport transition process |
— |
— |
Displayed outstanding temperature anti-interference |
112
|
Electrodes/PVDF/silicone rubber |
Kirigami-cut PVDF film |
— |
400% |
Applications in posture monitoring and athlete rehabilitation |
113
|
The strain sensor also displayed good real-time detection of a variety of human actions, including walking, leaping, and phonation. Also, the sensor could be easily stitched onto the cloth (Table 3).111 For a wearable strain detector created by utilising the improved PEDOT with a gauge factor of 2, the PSS thin film responded steadily to the interference (Table 3), and Fig. 4(c).112 The Kirigami-cut PVDF film sensor exhibits excellent stretchability, high sensitivity, and fast response time to strain and strain rate due to its unique geometric design. The sensor's performance was characterized using various testing methods, including tensile tests, bending tests, and strain rate tests. The authors envisage that the Kirigami-cut PVDF film sensor will find applications in various fields, including wearable devices, robotics, and human–machine interfaces (Table 3).113
3.3 Carbon-based materials construction, patterning and performance
Since there first discovery in 1991, carbon nanotubes have received a lot of interest. Superior mechanical, thermal, optical and electrical conductivity capabilities are found in carbon-based materials Fig. 4(d) and (e).114 Low density, great strength, high conductivity, remarkable flexibility and flexible integration with materials and sensors are just a few of the distinctive advantageous properties of carbon-based materials Fig. 5(a).115 Compared to other carbon-based products, carbon black is the least expensive. However, carbon-based materials (such as carbon black, carbon nanotubes, and graphene) are a superior option for the large-scale production of strain sensors (Table 4), Fig. 5(b) and (c).116,117 Zheng et al. prepared flexible composites by using thermoplastics as well as carbon nanomaterials and carbon black in a one-dimensional configuration. Materials with high sensitivity and GFs of 10.8 and 6.8 resulted from processing. A CB/TPU composite was capable of a 90% strain (Table 4), Fig. 5(d) and 6(a),118 In further research, Li et al. reported the fabrication of CNT and PVA-coated yarns with core–sheath structures. The strain sensor exhibits features including improved mechanical behaviour, high linear piezo resistivity, and electro-mechanical stability under monotonic as well as cyclic loads (Table 4), and Fig. 6(b).119 Graphene-based strain sensors can be created on substrates consisting of PDMS, TPU and certain rubbers, as well as other materials that are equivalent to CNT-based strain sensors. The GA/NRL-0.5 exhibited a 100–300 μm diameter sphere-like, high porosity morphology having a cross-section image of GA/NRL-0.5.120,121 The combination of spandex and nylon fabric with graphene was demonstrated by Lee et al. It was fabricated by a simple procedure. Even so, this strain sensor exhibited a strain of about 18.5% at a 40.6%-gauge factor (Table 4). Sun et al. reported on multi-walled carbon nanotubes and concluded that nanomaterials have outstanding tensile strain and high sensing capability.122 A sensor of this type was used to achieve exceptional strain rates of up to 720% (GF = 1768.7, 90–100% strain) and good endurance (1050 cycles at 10% strain). These critical qualities allowed MAPCN to reliably measure human parameters (Table 4), and Fig. 6(c).123 Through customizing the segregated conductive networks (SCNs) of CPCs, a new, straightforward, yet highly successful technique was devised to dramatically improve the electrical and sensing capabilities of flexible strain sensors. The post-processing method presented offers a global technological foundation for improving the electrical and sensing capabilities of CPCs for real-world uses (Table 4).124 Sun et al. demonstrated how to drop-coat graphene, carbon black, and polydimethylsiloxane (PDMS) into a 3D structure. The G/CB/Ni strain sensor demonstrated excellent flexibility, sensitivity, and long-lasting stability with a gauge factor of 138 at 16% strain. The G/CB/Ni sensor provided measurement capabilities of muscle strength as well as accurately tracking small human actions like blinking, pulse, and swallowing.125 The most frequently used types of graphene piezoresistive sensors comprise an alternative graphene composite hydrogel, that not only detects physiological parameters including heart rhythm, skin warmth, bloodstream content and exercise intensity, as well as significant physiological indications of inflammatory response and even glucose levels.126
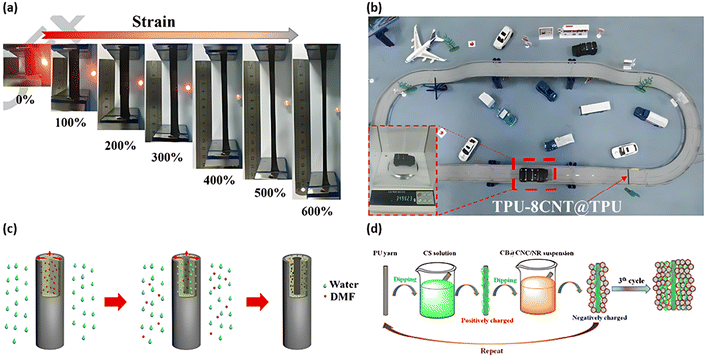 |
| Fig. 5 (a) While conducting, V-CNTs/TPU a strain detector was extended from 0 to 600% and the illumination of the LED was seen to change (Copyright © 2018, Wiley). (b) A graphical image demonstrating the construction of a smart transport network using TPU-8CNT@TPU (Copyright © 2018, American Chemical Society). (c) Diagram illustrating the creation of the stacked fibre's hollow-monolith structure (Copyright © 2018, American Chemical Society). (d) Illustration of the CPC@PU yarn strain device's structural design. (Copyright © 2016, American Chemical Society). | |
Table 4 Summary of carbon-based strain sensor's outcomes
Materials |
Method/process/structure |
Sensitivity |
Stretchability |
Application/other properties |
Ref |
CNTs/CB |
Through a layer-by-layer assembly method |
45.4 |
150% |
Excellent linearity application: monitoring human movement |
117
|
CB/TPU |
— |
10.8 |
20% |
High sensitivity |
118
|
CNT/PVA |
Core–sheath structure |
High |
100% |
Flexible intelligent devices |
119
|
Spandex/nylon/graphene |
Oxide dip-reduce |
18.5 |
40.6% |
Healthcare monitoring, body motion detection |
122
|
MWCNTs |
Anisotropic structure |
1768.7 |
90–100% |
Monitor human physiological activities |
122
|
CNT/Eco flex |
Segregated conductive networks (SCNs) |
176 |
130% |
Human motion monitoring and soft robotics |
124
|
Carbon/PDMS |
Wrinkled and cracked structure |
2300 |
Up to 50% |
Good linearity, rapid response |
125
|
PA6 and PA66/CB |
— |
15 to 25 |
— |
Structural health monitoring, wearable electronics, and smart textiles |
126
|
SBS/Gr/CNTs |
1D@2D@1D hierarchical structure |
1667 |
0–500%, |
Tracks physiological actions like bending of the finger, elbow, or knee with quick and precise reactions |
129
|
LM/CNTs |
— |
4.8 and 12.7 |
2200% |
A significant level of transparency, sufficient adherence, and long-lasting |
130
|
AgNPs/CNTs/PDA/TPU |
— |
High |
640% |
Possible uses for stretchable and flexible electronics by monitoring human motion |
131
|
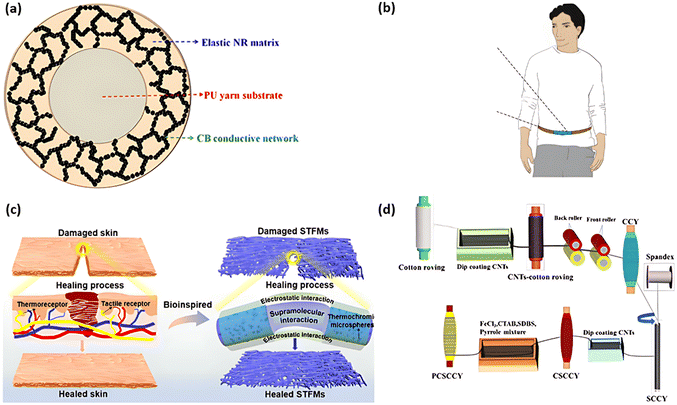 |
| Fig. 6 (a) Diagram illustrating the creation of the stacked fibre's hollow-monolith structure (Copyright © 2018, American Chemical Society). (b) Illustration of the CPC@PU yarn strain device's structural design (Copyright © 2016, American Chemical Society). (c) A representation of the structural layout of the CPC@PU yarn strain sensor (Copyright © 2016, American Chemical Society). (d) Schematic of the PCSCCY core-spun yarn electrolyte manufacturing operation (Copyright © 2020, American Chemical Society). | |
Wrinkled and cracked amorphous carbon film for high-performance flexible strain sensors presents a novel approach to fabricating a flexible strain sensor using amorphous carbon films in the wrinkled and cracked state. A sensor of this type exhibits high sensitivity, low detection limit, and excellent stability due to its unique surface morphology and material properties. The authors envision the potential applications of the wrinkled and cracked amorphous carbon film sensor in wearable electronics, healthcare monitoring, and structural health monitoring (Table 4).127 Another article explores the effect of adding carbon black to polyamides to enhance their mechanical and electrical properties for strain-sensing applications. The study found that the addition of carbon black improved the mechanical properties, such as stiffness and strength as well as the electrical conductivity of the polyamides. These multifunctional materials can potentially be used as strain sensors in various applications (Table 4).128 Another study presents a novel strain sensor made of highly stretchable and sensitive fibres composed of styrene–butadiene–styrene copolymer (SBS), graphene (Gr), and carbon nanotubes (CNTs). The hierarchical structure leads to high sensitivity and stretchability, allowing the fibres to withstand large strains without damage. The authors believe that their work can contribute to the development of high-performance strain sensors for various applications, such as wearable electronics and structural health monitoring. Highly stretchable and sensitive SBS/Gr/CNTs fibres with hierarchical structure for strain sensors (Table 4).129 Furthermore, researchers have developed a transparent strain sensor using a hydrogel-based material infused with liquid metal and carbon nanotubes. The sensor is suitable for monitoring the health of aquatic animals without interfering with their natural behaviour. The wireless sensor can detect small movements and changes in the body shape of animals and transmit the data to a receiver for analysis. The new technology has the potential to revolutionize the way marine biologists and researchers monitor the health of aquatic animals in their natural environment (Table 4), and Fig. 6(d).130 Wang et al. developed a strain sensor having properties that were characterized through various tests and the results showed promising potential for practical applications (Table 4).131
3.4 Overview of fibre-based strain sensor with construction, patterning and performance
Since ancient times, fibres have been a common material for clothing and other items used in daily life.132 Fibre-based materials are quite popular since they are both flexible and strong. At present have been initiatives to produce high-performance fibres. Nevertheless, it is still difficult for multi-functional large fibres to fulfil a variety of applications. Modern synthetic fibres, including textile fibres, are typically not influenced by environmental conditions such as stress, light, heat, humidity, etc., that place restrictions on the possible uses of fibre-based technologies in clothing warmers, robotics and people monitoring systems. Through very easy adjustments to the fabrication and processing of fibre materials can be made, sophisticated thread or yarn manufacturing and textile-making offers a reasonably cheap path to realising innovative thread-based wearable technologies Fig. 7(a).133–135 Cotton is the natural fibre that makes up the largest portion of the textile market, followed by silk, wool, flax, hemp, and others. However, there are difficulties in obtaining enough cotton because it can only be grown in sub-tropical regions and requires a lot of water and agricultural chemicals to produce large yields. In this context, various chemical compounds have a detrimental effect on the environment. It is important to create parallel markets for alternative natural fibres suited for textiles, such as hemp and jute, to minimize this risk.136 However, due to various advantages in terms of affordability and convenience, organic textile materials are much more appealing for smart textiles. Silk is a very comfortable fabric. The article discusses how the development of moisture-sensitive smart yarns and textiles can lead to the creation of advanced clothing that can adapt to changing environments. This technology is made possible by self-balanced silk fiber muscles, which react to moisture levels in the environment. The potential applications for this technology include athletic performance wear that can adapt to sweaty conditions, medical devices that can sense changes in the body, and even environmental sensors that can detect changes in moisture levels in the air. Overall, the development of these materials and technologies represents an exciting advancement in the field of smart textiles.137 Recent research on conductive fibres has produced results that have shown improvements in terms of the conductive fibre's strong electrical performance.138 Expandable conductive fibres with outstanding electrical conductivity and physical toughness are needed to create such smart electronic textiles (E-textiles), which will allow for washable textiles with lifespans comparable to those of traditional textiles. The fabrication process of CSCF Fig. 7(b).139 The production of nanotubes (CNTs)/thermoplastic polyurethane (TPU) fibre strain sensors using an electro spinning approach was described by Ren et al. The linked CNTs/TPU fibre materials with parallel direction showed extraordinarily high stability over 10
000 cycles at a 200 per cent strain and a stretch capacity of 900%, a rapid response time of only 70 ms and an ultra-low detection limit of 0.5%, indicating good sensitivity. Thereafter, a strain detector made from CNTs and TPU materials was constructed to observe significant human movements, including cheek bulging and vocalization. The final variant of the active V-CNTs/TPU stain sensor was extensible between 0% and 600% and the luminance of the LED changed, (Table 5).140 Fibres needed exceptional electrical conductivity, stretchability and wearability. Utilizing a parallel pattern consisting of thermoplastic elastomer-enveloped carbon nanotube fibres, coaxially wet spun were described by Jian Zhou et al. The researchers were able to create extremely stretchable and sensitive strain sensors. The sensors exhibited greater linearity, high stretchability, and high sensitivity with a gauge factor of 425 at 100% strain (Table 5), and Fig. 7(c).141 A simple coaxial wet-spun construction method is used to create a multi-layered, hollow, monolithic, electrically conductive CNT/TPU fibre with good stretchability and low density. This method produces high-performance wearable electronics. Digital images demonstrating the construction of an intelligent traffic system using TPU-8CNT@TPU (Table 5).142 Wu et al. showed that by covering polyurethane (PU) yarn using a very light, flexible, and durable conductive polymer composite (CPC) coating made of carbon black and organic rubber, it was possible to develop a straightforward, economical, and highly compliant methodology for making extremely sensitive strain sensors. The cost-effective manufacture of highly efficient strain monitoring systems possessing good selectivity and washing endurance, economic, convenience, and environmental acceptability is thus made possible. Demonstrates the manufacturing method for the CPC@PU yarn. The NR matrix includes the carbon-black electrical connection and the microstructure of the cross-section of CPC@PU is shown in Fig. 7(d), and (Table 5).143 Wang, et al. investigated a bi-sheath design of buckling rubber on rubber fibres and sheets of buckled carbon nanotubes. With this strain sensor, excellent linearity, quick response times, good resolution, exceptional stability and almost no hysteresis were achieved via sheath–core construction (Table 5).144 Dopamine was polymerized in situ to form an adhering polydopamine coating at the interface of polyurethane filaments (PFs). The full surface of the PFPF was silver-plated. The approach described for synthesizing SPPFs has lots of advantages including procedure simplicity, low cost, good durability (Table 5).145 Another research group, Cao et al., fabricated a technique for creating sheath–core silverware. It proved possible to create conductive fibres that revealed an enormous opportunity for the implementation of smart technologies as well as sensors with flexible carrying links (Table 5).146
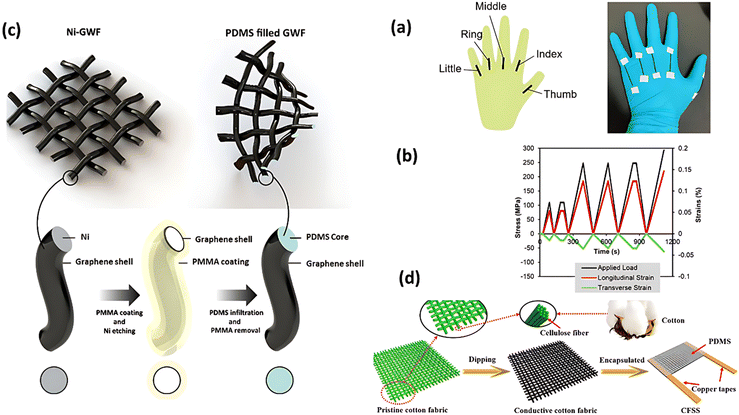 |
| Fig. 7 (a) NMS fabric's electrical heating characteristics, temperature fluctuations and infrared images (Copyright © 2019, American Chemical Society). (b) The longitudinal, transversal and functional strain of the metal plate (Copyright © 2019, American Chemical Society). (c) Stand-alone GWF's features and vapid GT GWF (E-GWF) (Copyright © 2019, American Chemical Society). (d) A graphical illustration of the CFSS process and the creation of pure cotton fibres (Copyright © 2020, American Chemical Society). | |
Table 5 Summary of the performance of fibre-based strain sensors
Substrate and sensitive materials |
Structure |
Technology |
Strain |
GF |
Repeatability |
Response time |
Others benefit |
Ref. |
TPU/CB |
Porous |
Spinning |
380% |
28 084 (204%) |
1000 (60%) |
200 ms |
Breaking stress and strain: 2.15 MPa |
140
|
TPE/SWCNTs |
Coaxial |
Spinning |
100% |
425 (100%) |
11 000 (60%) |
— |
High stretch ability |
141
|
TPU CNTs |
Hollow |
Spinning |
152% |
238 (50%) |
1000 (50%) |
— |
— |
142
|
PU CS |
Core–sheath PU |
Coating |
1% |
38.9 (1%) |
10 000 (1%) |
— |
Conductivity: 4.1 MΩ cm−1 |
143
|
SBS/CNTS |
Double sheath buckle |
Coating |
600% |
0.14 (200–600%) |
5000 (100%) |
80 ms |
— |
144
|
AgNPs/PF |
Core–sheath |
Coating |
— |
— |
— |
— |
Breaking strength and breaking elongation are 5% and 11.9% |
145
|
PU/AgNWs |
Core–sheath |
Coating |
60% |
5–9557 |
10 000 (1%) |
120 ms |
— |
146
|
Ag NPs substrate: Pu fibre |
Multifilament |
Embedding |
200% |
35 (0–100%), 659 (150–200%) |
10 000 (10%) |
— |
High sensitivity excellent sensing range |
148
|
For diagnosis as well as control of diabetes, flexible, non-invasive electromechanical sensors are preferred in glucose testing. According to Cai et al., this reversed iontophoresis (RI) technique was employed to apply the graphene fibre fabric utilized as dry sensor patches to skin surfaces as in vivo non-invasive diabetes tracking.147 Zhu et al. proposed a revolutionary contact protection technique. This technique was built into a fibre-based design of electronic skin by contact covalent bonds. This implementation of an e-skin in sophisticated smart sensor technologies is very attractive to manufacturers and users. Biological tissue and STFMs with self-healing properties.148 Additionally, Lee et al. revealed a simple way for producing extremely flexible and sensitive fibre strain sensors by inserting silver nanoparticles into a flexible fibre with a multi-thread design. The fibre strain sensors were able to be used in interactive textiles, wearable technology, and bioengineering. The fibre strain detectors can also be commonly incorporated into a glove to regulate the actions of a touch robot (Table 5).149
3.5 Overview of yarn-based wearable strain sensor with construction, patterning and performance
Yarns that are employed in producing fabric should be thin and flexible to assure the participant's comfort. Direct incorporation of electrical features into the garment environment has certain benefits, including improved comfort, movement, use and aesthetic qualities. However, to achieve its widespread use, some challenges must be solved.150 The following are numerous benefits of using yarn strain sensors: (a) the sensor is simple to utilize incorporating sensing strands in the textile construction. This allows innovations to present a wide range of detecting processes; (b) they can possess greater internal flexibility; (c) they have effortless performance and (d) they are non-invasive and create minimal skin inflammation.151
Another researcher revealed in their study a simple, flexible structure where wool yarns are used as an abundant, light and flexible natural resource in an environmentally friendly manufacturing process for wearable strain sensors. Using conductive ink and an uncomplicated coating technique, conductive woollen strands were created. Strain sensors can track the motion of design and interactivity to determine the degree of strain sensor response, and these sensors showed excellent agreement with the results of the experiments when obtaining status as manufactured products. Curcumin was used to encourage adherence on the surface of the fibre by hydrogen bonds via layering on a nesting catalyst, which effectively metallized the wrapped threads with Cu or Ni using ELD at ambient temperature. Even, Cai et al. demonstrated that the sheath–core yarn, which has an extremely high strain sensing range (0–350%) and exceptional stability, can be employed as a wearable strain sensor. Wearable strain sensing CNTs and polypyrrole PPy produced by spinning technology makes it suitable for use. Human muscles and joints Tables 6 and 7.152–154
Table 6 Summary of yarn-based strain sensor's optimal capabilities
Substrate and sensitive materials |
Structure |
Method/technology |
Strain |
GF |
Repeatability |
Response time |
Ref. |
Pu/CU |
Wrapped yarn |
ELD |
50% |
— |
5000 (50%) |
— |
153
|
PU/cotton and CNT/PPy |
Core-spun yarn |
Dip coating and in situ polymerization |
350% |
— |
— |
— |
155
|
TPU SWCNT/rGO |
Helical layer |
Spraying |
620% |
2160.4 |
1000 (50%) |
— |
155
|
Rubber/PET CNT |
Braided yarn |
Dip coating |
44% |
980 |
1000% |
200 ms |
157
|
Ag-nanoparticles/graphene-micro sheets(PU) |
1D coaxial |
Dip coating |
0–50% |
500 |
±1.56% |
— |
158
|
CNTs ink/polyurethane yarn (PU yarn) |
Crack microstructure |
Coating |
200% |
1344.1 |
1000% |
80 ms |
159
|
AgNWs and MXene |
0D–1D–2D |
Situ HF method |
200% |
872.79 |
1000 cycles |
0.1 mm s−1 |
160
|
Cotton/spandex core-spun |
Ribbon |
— |
Up to 100% |
1799 |
1000 cycles |
2 m |
161
|
Wool yarn |
Sandwich |
Simple coating |
200% |
5 and 7.75 |
— |
— |
152
|
Table 7 Summary of fabric-based strain sensors' performance
Substrate and sensitive materials |
Structure |
Method/technology |
Strain |
GF |
Repeatability |
Response time |
Ref. |
TPU nonwoven/rGO |
Micro-cracked structure |
Dip-coating |
98% |
180 (15%) |
1000 (10%) |
33 ms |
154
|
Silk knitted fabric/rGO |
Chemical structure |
Hot press |
10% |
124.5 (10%) |
1000 (2%) |
— |
165
|
PDMS woven fabric/rGO |
Hierarchical structure |
— |
30% |
70 (10%), 282 (20%) |
— |
∼0.07 s |
166
|
PDA/PANI/PPy/PDMS |
— |
Dip coating |
— |
— |
10 000 times |
500 ms |
167
|
Cotton/PDMS |
Woven structure |
Coating |
75% |
30% |
10 000 cycles |
∼90 ms |
168
|
CNT/non-woven |
Non-woven |
Coating |
0.4% |
1.9 and 4.0 |
A high degree of repeatability |
Real-time response |
164
|
TPU/CNTs |
— |
Dip-coating |
400% |
1571 |
10 000 cycles |
— |
169
|
Nanoscale silica dioxide/PDMS |
Knitted |
Dip-coating |
— |
— |
4000 cycles |
22 ms |
170
|
GNSs/MWCNTs |
— |
One-step screen-printing method |
30% |
40 |
— |
— |
171
|
It is still difficult to combine several functionalities in a textile matrix in a way that is both structurally stable and useful. Through the optimization of several spinning processes the core-spun yarn electrode fabrication process, the textile structure was utilised to manufacture extremely flexible sheath–core yarn to solve this problem (Table 6).155 According to research by Xie et al., the intensity, permeability and sensibility of the sensor can be considerably improved by adding a limited quantity of 2D rGO to a 1D CNT system (with a gauge factor up to 2160.4). The sensor has a large working strain of 620% and great durability because of its number of layers (Table 6).156 Pan et al. described a straightforward method for mass-producing sensor devices made of core–sheath nanomaterials yarn (CSCY) with great sensitivity and improved stability. Finally, BYs-CNT and CSCY were wound onto a motor collector. It was shown that the CSCY strain sensor could track both delicate and active human activity in real-time (Table 6).157 The majority of reported flexible and wearable sensors use two-dimensional (2D) planar strip topologies that are ineffective for integrating into textile structures and have qualities incorporating comfort, air permeability and smoothness. For this reason, Li et al. developed a novel one-dimensional wearable strain detector yarn with an elastic polyurethane core, a channel composite sheath made of graphene micro sheets, Ag nanoparticles and a silicone encapsulating layer. The fabricated strain sensor had an approximate gauge factor of 500, excellent linearity of 0.98, exceptional stretchability and extremely reproducible mechanical durability (a repeatable error of 1.56%) (Table 6).158 It has been found that the stretching vibration peak of C–O–C occurred at 1100 cm−1 in the spectra of pure polyurethane PU yarn and CNTs/PU yarn. The hydrogen bond is believed to be the most powerful bond between both the electrical conductor and the elastic substrates (Table 6).159 Li et al. reported the fabrication of 0D–1D–2D multidimensional silver Ag/MXene nanoparticles as strain sensors, which had extreme sensitivity and flexible performance. This technique greatly improved sensitive and stretchable performance with a high gauge factor even at very high strains (350%) for AgNPs. A bridge was constructed between AgNWs and MXene and the fabric was knit using NMS yarns Table 6.160 Under continuous strain loading, deformation fracturing of the electrical pathways provided by the fibres/yarn breaks was avoided, resulting in good operational longevity. According to Feng et al. even within the 0 to 100% strains range, the strain sensor's resistance rose proportionately with strain, and reproducible data could be obtained for more than 1000 tensile cycles (Table 6).161
4. Overview of fabric-based strain sensors with construction, patterning and their performance
Wearable devices can easily incorporate fabric-based strain sensors to monitor parameters. Several techniques have been used to capture physiological data. Even though a variety of methods have been suggested to improve the fabric-based strain sensor's sensing capabilities, solving the associated problems is still difficult.
Wearable sensors need to be lightweight for portability, very sensitive to detect minute movements in people, flexible and stretchable to handle significant deformations, durable with lower bounce risks, and biocompatible for complex systems to achieve linearity and stability over large stress ranges.162,163 Considering non-woven composite sensors fabricated using carbon nanotubes (CNTs), the nanotubes are coated onto non-woven transmission fabrics using an easily scalable technique. Linearity up to 0.4% strain is achieved, also the sensors offer repeatability. The longitudinal and transverse elastic strain gauge factors are given Table 7.164 Liu et al. constructed a micro-cracked non-woven textile (NWF) strain sensor for adjustable garments through dipping layers inside the hydrophobic silicon dioxide (Hf-SiO2)/ethanol solution. The strain sensor, fabricated with a G content of 25 wt%, is capable of a broad working range at 98% and GF values up to 2.36. Additionally, the detector had remarkable self-cleaning properties, uniformity, waterproofness (WCA = 154°), anti-corrosion properties, detecting capabilities for strains as small as 0.1% and rapid reaction times (33 ms). Using the hot press approach, Wang et al. created a strain sensor from a Graphene-silk fabric. A maximum GF of 124 was achieved in the 10% strain range and the sensor exhibited reasonable linearity and had acceptable mechanical properties. This graphene–silk fabric also had certain multifunctional qualities, including UV protection and hydrophobicity (Table 7).165 To make E-GWFs that resemble spider webs, a flexible PDMS elastomer was poured into hollowed GTs of CVD-grown GWFs. Liu et al. reported that the EGWF/PDMS sensing simultaneously showed high gauge factors at 10% strain which ascends to 282 at 20% and outstanding linearity of 0.981 for strains in the range of 0 to 10%. It has been proven that a spider web's excellent qualities result directly from the cooperative synergy of its hierarchically arranged parts (Table 7).166 To sequentially cover the fabric, in situ polymerization and dip-coating have been used with polydopamine (PDA), polyaniline (PANI), polypyrrole (PPy) and polydimethylsiloxane (PDMS). The resulting sample had a rapid electrical reaction (500 ms) and was able to survive 10
000 tensile strain cycles. It also performed satisfactorily in terms of electro-thermal and photo-thermal processes (Table 7).167 Two varieties of cotton fabric-based strain sensors (CFSS), known as CFSS-90° and CFSS-45 cotton fabrics are coated with graphene nano sheets via a repeated dipping process and sealed inside polydimethylsiloxane (PDMS). By comparison with CFSS-90° (1.75 MPa and 30% strain), CFSS-45° had a higher stress level and strain of around 4.5 MPa and 75% respectively. The cotton fabric-based strain sensors CFSSs exhibit impressive consistency and endurance CFSS-45° 10
000 cycles at 30% strain (Table 7).168 From another research project, the thermoplastic polyurethane and carbon nanotube TPU/CNTs strain detector display a superb array of qualities, including high sensitivity, exceptional tensile strength and toughness, and stable cycling durability (Table 7).169 Using chitosan (CS), a reduced graphene oxide (rGO) conductive fabric was first created electrostatically. The sensor demonstrated superior stability while cycling, extreme hydrophobicity, and rapid response. For sportsmen to detect their movements while being affected by water and as a warmer to assure the wearer's support, this sensor might be employed as winter sportswear in winter-time (Table 7).170
Another article presents a new type of flexible and self-adhesive strain sensor that is based on graphene nanosheets (GNSs) and multi-walled carbon nanotubes (MWCNTs). The sensor can detect and monitor different types of hand gestures, including finger bending and wrist rotation, by measuring the changes in electrical resistance caused by the stretching of the fabric. The authors demonstrated the effectiveness of the sensor in controlling a robotic arm and a prosthetic hand. The new sensor has the potential to be used in various applications such as wearable electronics, human–machine interfaces, and rehabilitation devices (Table 7).171
5. Applications of textile-based strain sensors
The development of wearable sensors has attracted significant attention in recent years, as they offer a non-invasive and convenient method for monitoring human physiological signals. However, textile-based strain sensors have gained significant attention in recent years due to their potential applications in various fields, including human health monitoring, physiotherapy, and injury prevention. These sensors are lightweight, flexible, and comfortable to wear, making them suitable for continuous monitoring of human body movements and activities. In particular, strain sensors that can be integrated into clothing have received burgeoning attention due to their potential applications in human motion detection. Traditional strain sensors, such as metal foil or semiconductor-based sensors, are rigid and bulky, which limits their use in wearable applications. Therefore, there is a need for developing flexible and comfortable strain sensors that can be integrated into clothing.172,173
5.1 Discussion of human motion monitoring
Human motion monitoring by strain sensors involves the use of sensors to measure the deformation or strain in a person's body during movement. This approach has the potential to provide valuable information about movement patterns, gait analysis, and biomechanics, which can be useful in applications such as sports performance monitoring, physiotherapy, and injury prevention.174 The biodegradable Eco flex encapsulated bacterial cellulose/polypyrrole strain sensor is a novel technology that allows for the detection of motion with high sensitivity, flexibility, and scalability. The strain sensor can detect a range of motions, including bending, stretching, and twisting, with high accuracy and sensitivity. Overall, the biodegradable Eco flex encapsulated bacterial cellulose/polypyrrole strain sensor represents a significant advancement in sensor technology with promising applications in various fields.175
Waterproof conductive fibre with a microcracked synergistic conductive layer has emerged as a promising technology for high-performance tunable wearable strain sensors. These sensors have a wide range of potential applications in fields such as healthcare, sports, and the military.176 The multifunctional hydrophobic fabric-based strain sensor is a wearable device that can detect human motion and provide personal thermal management. The sensor is made of a hydrophobic fabric that can repel water and sweat, making it suitable for use during physical activities. The strain sensor is integrated into the fabric and can detect movements of the wearer, such as walking or running. The data collected by the sensor can be used for a variety of applications, including fitness tracking, medical monitoring, and virtual reality gaming. Overall, the multifunctional hydrophobic fabric-based strain sensor offers a promising solution for enhancing human performance and well-being Fig. 8(a).177 A flexible capacitive strain sensor to monitor hand movements and a deep learning algorithm were used to classify the different hand gestures performed in Fig. 8(d).178
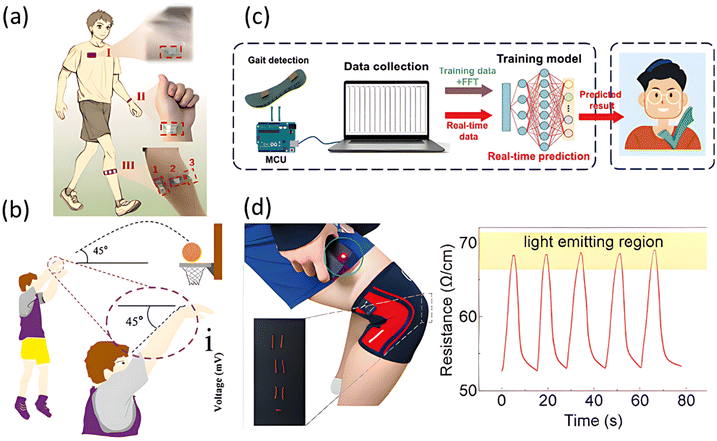 |
| Fig. 8 (a) Depiction of an elastic knee band (Copyright © 2021, American Chemical Society). (b) An illustration of the self-powered sensors that basketball players wear to track their shooting form and increase their hitting accuracy (Copyright © 2022, Chemical Engineering Journal). (c) Physical therapy (Copyright © 2021, Advanced Science). (d) Hand gesture movement (Copyright © 2022, Biosensors and Bioelectronics). | |
5.1.1 Sports performance monitoring.
In terms of practical applications, textile-based strain sensors have been used in a variety of fields, including sports and athletics, healthcare, and industrial manufacturing. For example, in the field of sports, these sensors have been used to monitor muscle activity and fatigue during exercise Fig. 8(b).179 Yarn-based strain sensors are a new class of sensors that have the potential to revolutionize the field of wearable technologies. Yarn-based strain sensors have been used in various applications, such as monitoring breathing, heart rate, and body motion. The sensor can be integrated into a wearable device that can be worn by athletes to monitor their performance. The sensor can also be used in healthcare settings to monitor patients' movements during rehabilitation. Additionally, the sensor can be used to develop smart clothing that can track body movements and provide feedback to the wearer.180 One recent application of textile-based strain sensors can be found in the article “Flexible textile strain sensor based on carbon nanotube-coated nylon yarns for human motion detection” by Liu et al. In this study, the researchers developed a flexible textile-based strain sensor using carbon nanotube-coated nylon yarns as described. The sensor was designed to detect and monitor human motion, specifically for use in a smart garment. The sensor was able to measure strain in multiple directions and was highly sensitive, with a gauge factor of 2.2. The sensor was integrated into a wearable sleeve and tested on human subjects during various exercises. The results showed that the sensor was able to accurately detect and measure the strain caused by different types of movements, including bending and stretching.181 The use of self-powered sensors based on triboelectric nanogenerators (TENGs) for sports monitoring applications is explored. TENGs are described as power sensors that can be worn by athletes to monitor various parameters related to sports performance, such as body motion, muscle activity, and temperature. One of the key advantages of TENG-based sensors is that they are self-powered, which makes them ideal for sports monitoring applications where the user needs to wear the sensors for extended periods. However, the authors propose several solutions to these challenges, such as using materials with high triboelectric properties and developing algorithms to filter out unwanted motion signals. Overall, the paper highlights the potential of TENG-based sensors for sports monitoring applications Fig. 8(c) and provides a roadmap for future research in this area.182
5.1.2 Physiotherapy.
In physical therapy, textile-based strain sensors can be used to track the progress of patients undergoing rehabilitation programs. These sensors can be incorporated into garments, such as compression sleeves, to monitor muscle activity and joint movements during exercises. This information can then be used to adjust the rehabilitation program to suit the patient's needs and improve their recovery outcomes physiotherapy, also known as physiotherapy, is a healthcare profession that focuses on the prevention and treatment of physical impairments, disabilities, and pain. The main goal of physiotherapy is to improve a patient's quality of life through various techniques such as exercise, manual therapy, and education. Another study by Zhang et al. (2022) developed a wearable textile-based sensor for monitoring and analyzing the gait patterns of stroke patients. The sensor was integrated into a sock and could accurately measure the foot's strain during walking. This information could be used to analyze the patient's gait pattern and identify any abnormal patterns that need to be corrected during rehabilitation.183 One application of physiotherapy using textile-based strain sensors is in the monitoring of muscle activity during exercise. Researchers developed a wearable textile-based strain sensor that could be used to monitor muscle activity during resistance training exercises. The sensor was integrated into a compression sleeve worn on the upper arm and was able to detect changes in muscle length and tension.184 Strain gauge integration is a useful tool in the assessment and rehabilitation of knee osteoarthritis. It involves placing a small device called a strain gauge on the skin over the knee joint to measure the strain or deformation of the joint during movement. This information can be used to monitor the progress of rehabilitation, evaluate the effectiveness of different interventions, and customize treatment plans for individual patients. The use of strain gauge integration has shown promising results in improving knee function and reducing pain in patients with knee osteoarthritis.185 Overall, physiotherapy continues to evolve and improve with new research and advancements in technology. Physiotherapy remains an important healthcare profession that helps millions of patients each year and hence the role of sensing in this discipline gains in importance.
5.1.3 Injury prevention.
In injury prevention, textile-based strain sensors can be used to monitor the movements of athletes and prevent injuries caused by overexertion or using an incorrect technique. By tracking joint movements and muscle activity, coaches and trainers can identify potential injury risks and adjust training programs accordingly. Moreover, these sensors can also be used to monitor the physical activity of workers in physically demanding jobs, reducing the risk of work-related injuries and improving workplace safety. Injury prevention refers to the various measures and strategies that are put in place to reduce the risk of injuries occurring. It involves identifying potential hazards and implementing strategies to eliminate or minimize the risk of injury. The fibre-based strain sensor was able to track knee joint motion with a high degree of accuracy. The sensor was able to detect changes in knee joint angle with a resolution of 0.5 degrees. The sensor readings were highly correlated with those obtained using the motion capture system (r = 0.95, p < 0.001).186 The fibre-based strain sensor has potential applications in the monitoring and rehabilitation of knee injuries. The sensor is non-invasive and can be easily attached to the skin above the knee joint. The sensor can be used to monitor knee joint motion during rehabilitation exercises, allowing clinicians to track progress and adjust treatment plans accordingly. “Development of a textile-based strain sensor for monitoring muscle activity in athletes” was published by Kim et al. (2021). This paper describes the development of a textile-based strain sensor system that can monitor muscle activity in athletes. The authors demonstrate the feasibility of using the system to detect changes in muscle activity during physical activity and it is suggested that it could be used to prevent injuries and optimize training Fig. 8(d).187 “Wearable strain sensors for injury prevention in sports” by Zhang (2017) is a paper that discusses the development of wearable strain sensors that can be used for injury prevention in sports. The authors propose a sensor design that is both comfortable and reliable, and they demonstrate the feasibility of using the sensors to detect muscle strain and joint movement.188
Overall, the application of textile-based strain sensors for human health monitoring, physiotherapy, and injury prevention shows great potential in improving human performance and overall well-being. Further research and development are required to optimize the design and functionality of these sensors, making them more suitable for practical applications in various fields.
6. Present challenges for flexible, wearable textile-based strain sensors
Flexible, wearable textile-based strain sensors are a promising technology for various applications, including health monitoring, sports and fitness, and human–machine interfaces. However, there are several obstacles to overcome to fully realize their potential. Here are some of the obstacles:
Accuracy and reliability: one of the main challenges of textile-based strain sensors is ensuring accurate and reliable measurements. The sensors need to be sensitive enough to detect small changes in strain, but also robust enough to withstand repeated use and washing.
Calibration: textile-based strain sensors require careful calibration to ensure accurate measurements. Calibration can be time-consuming and complex, and calibration errors will inevitably lead to inaccurate measurements.
Durability: textile-based strain sensors need to be durable enough to withstand the wear and tear of everyday use, including washing and stretching. They also need to be resistant to environmental factors such as heat, moisture, and UV radiation.
Interference: textile-based strain sensors can be susceptible to interference from other electronic devices, such as smartphones or Wi-Fi signals. This interference can lead to inaccurate measurements or even complete failure of a sensor.
Cost: developing and manufacturing textile-based strain sensors can be expensive, which can limit their widespread adoption. Finding cost-effective materials and manufacturing processes is crucial for making these sensors more accessible.
Compatibility: textile-based strain sensors need to be compatible with a variety of devices and platforms, including smartphones, wearable devices, and medical monitoring systems. Ensuring compatibility can be a challenge, especially given the rapidly evolving nature of these technologies. Overall, while there are many obstacles to the widespread adoption of textile-based strain sensors, researchers and engineers are working hard to overcome these challenges and realize the full potential of this exciting technology.
7. Conclusions and future developments
Textile-based wearable flexible strain sensors have been comprehensively researched and analysed here. Capacitive, resistive, piezoelectric and other strain sensors are now available, as are turboelectric strain sensors. In this review, textile-based conductive materials are discussed, including strain sensors which are made of carbon, metal and polymeric materials. Mechanical, thermal and electrical properties of conductive materials used for sensors are also investigated, along with performance indicators e.g. sensing range, responsiveness, recovery time, linearity, repeatability and durability. Advantages and disadvantages were evaluated. The methodologies used and the properties of several metals, including gold, copper, aluminium, silver, and zinc, have been documented. Modification and production processes have been described and images are shown including photographs which clarify these processes. Another consideration is the availability of these essentially two polymer-based materials, both natural and man-made for example, this review considers the implications for human, animal and plant vulnerability, concerning the pharmaceutical industry, cosmetics, science and technology. Finally, the performance of various materials, including their sensitivity and stretchability, has been summarized. Several methods, including the use of Woven and knitted conductive strands with textiles, as well as interface stitching and embroidery of conductive threads, have been proposed to create conductive textiles. Although textile-based strain sensors have shown advancements in raw materials, processing methods and structural developments, there are still numerous issues and obstacles that need to be resolved for strain sensors in fabrics to be used on a large scale over a range of industries, including universal health care, confidentiality, infotainment, the technology sectors, in the military and also for sport. It is reasonable to believe that many other spheres of life will eventually use these technologies. More research is needed to diversify and improve strain sensors. These sensors need to have large detecting ranges and high sensitivity. In addition, further features should be added to the platform for fabric, fibre, and yarn-based sensors to create wearable systems that are intelligent and responsive. For such technology to become ubiquitous, autonomous and intelligent, two or more functions need to be addressed. These include the nature of displays possibly incorporating structural colouring and photoluminescence that will depend on fibre performance. There is great potential but also perceived obstacles for designing textile-based strain sensors and these issues should be essential topics for further cross-disciplinary research.
Author contributions
Conceptualization, Liza; methodology, Liza and Md Homaune Kabir; formal analysis, Liza and Md Homaune Kabir; investigation, Liza and Liang Jiang; resources, Shaojuan Chen; writing-original draft, Liza; writing-review & editing, Liang Jiang and Stephen Jerrams; supervision, Liang Jiang and Shaojuan Chen; project administration, Shaojuan Chen; funding acquisition, Liang Jiang and Shaojuan Chen. All authors have read and agreed to the published version of the manuscript.
Conflicts of interest
The author declares no conflicts of interest in this study.
Acknowledgements
The authors gratefully acknowledge the National Natural Science Foundation of China (Grant no. 51703108 and Grant no. 52003130), the Postdoctoral Science Foundation of China (Grant no. 2019M652318) and the Taishan Scholar Foundation of Shandong, China (Grant no. tsqn201909100) for financial support.
References
-
P. Bosowski, M. Hoerr, V. Mecnika, T. Gries and S. Jockenhövel, in Electronic Textiles, Elsevier, 2015, pp. 75–107 Search PubMed.
- T. Lee, W. Lee, S. W. Kim, J. J. Kim and B. S. Kim, Adv. Funct. Mater., 2016, 26, 6206–6214 CrossRef CAS.
- P. Yu, X. Li, H. Li, Y. Fan, J. Cao, H. Wang, Z. Guo, X. Zhao, Z. Wang and G. Zhu, ACS Appl. Mater. Interfaces, 2021, 13, 24062–24069 CrossRef CAS PubMed.
-
P. Kozyr, A. Saveliev and L. Kuznetsov, in 2021 International Siberian Conference on Control and Communications (SIBCON), IEEE, 2021, pp. 1–5 Search PubMed.
- L. Cai, L. Song, P. Luan, Q. Zhang, N. Zhang, Q. Gao, D. Zhao, X. Zhang, M. Tu and F. Yang, Sci. Rep., 2013, 3, 1–9 Search PubMed.
- C. Zhao, J. Niu, Y. Zhang, C. Li and P. Hu, Composites, Part B, 2019, 178, 107447 CrossRef CAS.
- C. Hu, L. Cheng, Z. Wang, Y. Zheng, S. Bai and Y. Qin, Small, 2016, 12, 1315–1321 CrossRef CAS PubMed.
- L. Lu, N. Zhao, J. Liu and B. Yang, J. Mater. Chem. C, 2021, 9, 9309–9318 RSC.
- N. Turdakyn, A. Medeubayev, I. Abay, D. Adair and G. Kalimuldina, Mater. Today: Proc., 2022, 49, 2478–2481 CrossRef CAS.
- H. Lei, Y. Chen, Z. Gao, Z. Wen and X. Sun, J. Mater. Chem. A, 2021, 9, 20100–20130 RSC.
- H. M. Lee, S. Y. Choi, A. Jung and S. H. Ko, Angew. Chem., 2013, 125, 7872–7877 CrossRef.
- M. A. Saleh, R. Kempers and G. W. Melenka, Sens. Actuators, A, 2021, 328, 112764 CrossRef CAS.
- S. Chen, J. Qi, S. Fan, Z. Qiao, J. C. Yeo and C. T. Lim, Adv. Healthcare Mater., 2021, 10, 2100116 CrossRef CAS PubMed.
- Y. Gao, L. Yu, J. C. Yeo and C. T. Lim, Adv. Mater. Technol., 2020, 32, 1902133 CAS.
- J. Wang, C. Lu and K. Zhang, Energy Environ. Mater., 2020, 3, 80–100 CrossRef.
- U. Pierre Claver and G. Zhao, Adv. Eng. Mater., 2021, 23, 2001187 CrossRef.
- H. Souri, H. Banerjee, A. Jusufi, N. Radacsi, A. A. Stokes, I. Park, M. Sitti and M. Amjadi, Adv. Intell. Syst., 2020, 2, 2000039 CrossRef.
- A. H. Anwer, N. Khan, M. Z. Ansari, S.-S. Baek, H. Yi, S. Kim, S. M. Noh and C. Jeong, Sensors, 2022, 22, 4460 CrossRef CAS PubMed.
- X. Zeng, H.-T. Deng, D.-L. Wen, Y.-Y. Li, L. Xu and X.-S. Zhang, Micromachines, 2022, 13, 254 CrossRef PubMed.
- A. Leber, B. Cholst, J. Sandt, N. Vogel and M. Kolle, Adv. Funct. Mater., 2019, 29, 1802629 CrossRef.
- C. Wang, K. Xia, H. Wang, X. Liang, Z. Yin and Y. Zhang, Adv. Mater. Technol., 2019, 31, 1801072 Search PubMed.
- M. Amjadi, K. U. Kyung, I. Park and M. Sitti, Adv. Funct. Mater., 2016, 26, 1678–1698 CrossRef CAS.
- K. H. Kim, N. S. Jang, S. H. Ha, J. H. Cho and J. M. Kim, Small, 2018, 14, 1704232 CrossRef PubMed.
- J. Park, R. Ghosh, M. S. Song, Y. Hwang, Y. Tchoe, R. K. Saroj, A. Ali, P. Guha, B. Kim and S.-W. Kim, NPG Asia Mater., 2022, 14, 1–13 CrossRef.
- L. E. Aygun, V. Kumar, C. Weaver, M. Gerber, S. Wagner, N. Verma, B. Glisic and J. C. Sturm, Sensors, 2020, 20, 1386 CrossRef CAS PubMed.
- Y. Chen, Y. Jiang, W. Feng, W. Wang and D. Yu, Colloids Surf., A, 2022, 635, 128055 CrossRef CAS.
- N. A. Demidenko, A. V. Kuksin, V. V. Molodykh, E. S. Pyankov, L. P. Ichkitidze, V. A. Zaborova, A. A. Tsymbal, S. A. Tkachenko, H. Shafaei and E. Diachkova, Bioengineering, 2022, 9, 36 CrossRef CAS PubMed.
- M. Liu, Y. Sheng, C. Huang, Y. Zhou, L. Jiang, M. Tian, S. Chen, S. Jerrams, F. Zhou and J. Yu, Composites, Part A, 2023, 164, 107296 CrossRef CAS.
- Y. Kim, K. Faseela, S. Y. Yang, K. Kim, H. J. Yu, J. Y. Lim, J. G. Do, H. R. Choi, J. H. Hwang and S. Baik, Compos. Sci. Technol., 2022, 221, 109305 CrossRef CAS.
- Z. Wang, K. Zhang, Y. Liu, H. Zhao, C. Gao and Y. Wu, Compos. Struct., 2022, 282, 115071 CrossRef CAS.
- O. Atalay, Materials, 2018, 11, 768 CrossRef PubMed.
- T. Yan, Y. Wu, W. Yi and Z. Pan, Sens. Actuators, A, 2021, 327, 112755 CrossRef CAS.
- F. Fang, H. Wang, H. Wang, X. Gu, J. Zeng, Z. Wang, X. Chen, X. Chen and M. Chen, Micromachines, 2021, 12, 252 CrossRef PubMed.
- R. Madhavan, New J. Chem., 2022, 46, 17596–17609 RSC.
- H. Fu, B. Wang, J. Li, J. Xu, J. Li, J. Zeng, W. Gao and K. Chen, Mater. Horiz., 2022, 9, 1412–1421 RSC.
- J. Zhou, X. Long, J. Huang, C. Jiang, F. Zhuo, C. Guo, H. Li, Y. Fu and H. Duan, npj Flexible Electron., 2022, 6, 1–11 CrossRef.
- R. B. Mishra, N. El-Atab, A. M. Hussain and M. M. Hussain, Adv. Mater. Technol., 2021, 6, 2001023 CrossRef.
- H. Wang, Z. Li, Z. Liu, J. Fu, T. Shan, X. Yang, Q. Lei, Y. Yang and D. Li, J. Mater. Chem. C, 2022, 10, 1594–1605 RSC.
- F. Wang, Y. Tan, H. Peng, F. Meng and X. Yao, Mater. Lett., 2021, 303, 130512 CrossRef CAS.
- X. Hu, F. Yang, M. Wu, Y. Sui, D. Guo, M. Li, Z. Kang, J. Sun and J. Liu, Adv. Mater. Technol., 2022, 7, 2100769 CrossRef CAS.
- L. Zhao, Z. Ren, X. Liu, Q. Ling, Z. Li and H. Gu, ACS Appl. Mater. Interfaces, 2021, 13, 11344–11355 CrossRef CAS PubMed.
- S. Hosseini, H. Hajghassem and M. F. Ghazani, Mater. Res. Express, 2022, 9, 065605 CrossRef.
- Y. Gu, T. Zhang, H. Chen, F. Wang, Y. Pu, C. Gao and S. Li, Nanoscale Res. Lett., 2019, 14, 1–15 CrossRef PubMed.
- H. S. Wang, S. K. Hong, J. H. Han, Y. H. Jung, H. K. Jeong, T. H. Im, C. K. Jeong, B.-Y. Lee, G. Kim and C. D. Yoo, Sci. Adv., 2021, 7, 5683 CrossRef PubMed.
- Y.-G. Kim, J.-H. Song, S. Hong and S.-H. Ahn, npj Flexible Electron., 2022, 6, 1–8 CrossRef.
- Y. S. Rim, S. H. Bae, H. Chen, N. De Marco and Y. Yang, Adv. Mater. Technol., 2016, 28, 4415–4440 CAS.
- A. Singh, S. Lee, H. Watanabe and H. Lee, ACS Appl. Electron. Mater., 2020, 2, 523–528 CrossRef CAS.
- Y. Wang, Y. Yue, F. Cheng, Y. Cheng, B. Ge, N. Liu and Y. Gao, ACS Nano, 2022, 16, 1734–1758 CrossRef CAS PubMed.
- J. Li, L. Fang, B. Sun, X. Li and S. H. Kang, J. Electrochem. Soc., 2020, 167, 037561 CrossRef CAS.
- B. Niu, S. Yang, T. Hua, X. Tian and M. Koo, Nano Res., 2021, 14, 1043–1052 CrossRef CAS.
- H. Chen, F. Zhuo, J. zhou, Y. Liu, J. Zhang, S. Dong, X. Liu, A. Elmarakbi and H. Duan, Chem. Eng. J., 2023, 464, 142576 CrossRef CAS.
- M. C. Karlapudi, M. Vahdani, S. M. Bandari, S. Peng and S. Wu, Sensors, 2023, 23, 3245 CrossRef CAS PubMed.
- D.-Y. Wang, H.-H. Zhu, J. Wang, Y.-J. Sun, L. Schenato, A. Pauto and B. Shi, Eng. Geol., 2023, 314, 107011 CrossRef.
- J. Kim, J. W. Kim, K. Keum, H. Lee, S. Kim and J. S. Ha, Chem. Eng. J., 2023, 141278 CrossRef CAS.
- C. Liu, H. Chen, Q. Chen, Z. Gao, B. Wu, X. Fan and M. Ma, Opt. Laser Technol., 2023, 159, 108935 CrossRef.
- P. Gao, Y. Liu, X. Zheng, Z. Wang, C.-M. Yang, N.-H. You and B. Yang, Opt. Commun., 2023, 526, 128942 CrossRef CAS.
- Z. Liu, T. Zhu, J. Wang, Z. Zheng, Y. Li, J. Li and Y. Lai, Nano-Micro Lett., 2022, 14, 61 CrossRef CAS PubMed.
- J. Song, Y. Tan, Z. Chu, M. Xiao, G. Li, Z. Jiang, J. Wang and T. Hu, ACS Appl. Mater. Interfaces, 2018, 11, 1283–1293 CrossRef PubMed.
- W. Root, T. Wright, B. Caven, T. Bechtold and T. Pham, Polymers, 2019, 11, 784 CrossRef PubMed.
- A. Ali, V. baheti, M. Vik and J. Militky, J. Phys. Chem. Solids, 2020, 12, 109181 CrossRef.
- A. Ojstršek and S. Gorgieva, Polymers, 2020, 12, 109181 Search PubMed.
- Y. Lu, M. Tian, X. Sun, N. Pan, F. Chen, S. Zhu, X. Zhang and S. Chen, Composites, Part A, 2019, 117, 202–210 CrossRef CAS.
- H. Souri, J. Yu, H. Jeon, J. W. Kim, N.-H. You and B. Yang, Carbon, 2017, 120, 427–437 CrossRef CAS.
- Y. Yang, L. Shi, Z. Cao, R. Wang and J. Sun, Adv. Funct. Mater., 2019, 29, 1807882 CrossRef.
- J. Song, Y. Tan, Z. Chu, M. Xiao, G. Li, Z. Jiang, J. Wang and T. Hu, ACS Appl. Mater. Interfaces, 2018, 11, 1283–1293 CrossRef PubMed.
- X. Xiao, L. Yuan, J. Zhong, T. Ding, Y. Liu, Z. Cai, Y. Rong, H. Han, J. Zhou and Z. L. Wang, Adv. Mater., 2011, 23, 5440–5444 CrossRef CAS PubMed.
- J. Lee, S. Kim, J. Lee, D. Yang, B. C. Park, S. Ryu and I. Park, Nanoscale Res. Lett., 2014, 6, 11932–11939 RSC.
- W. Yi, Y. Wang, G. Wang and X. Tao, Polym. Test., 2012, 31, 677–684 CrossRef CAS.
- Y. Lu, M. Tian, X. Sun, N. Pan, F. Chen, S. Zhu, X. Zhang and S. Chen, Composites, Part A, 2019, 117, 202–210 CrossRef CAS.
- A. Atalay, V. Sanchez, O. Atalay, D. M. Vogt, F. Haufe, R. J. Wood and C. J. Walsh, Adv. Mater. Technol., 2017, 2, 1700136–1700340 CrossRef.
- T. Yang, X. Jiang, Y. Zhong, X. Zhao, S. Lin, J. Li, X. Li, J. Xu, Z. Li and H. Zhu, ACS Sens., 2017, 2, 1440–1450 Search PubMed.
- P. Jiang, Z. Ji, X. zhang, Z. Liu and X. Wang, Progress in Additive Manufacturing, 2018, 3, 65–86 CrossRef.
- W. Li, Y. Zhou, Y. Wang, L. Jiang, J. Ma, S. Chen and F. L. Zhou, Adv. Electron. Mater., 2021, 7, 2000865–2000899 CrossRef CAS.
- X. Zhang, X. Wang, Z. Lei, L. Wang, M. Tian, S. Zhu, H. Xiao, X. Tang and L. Qu, ACS Appl. Mater. Interfaces, 2020, 12, 14459–14467 CrossRef CAS PubMed.
- J. Cai, M. Yang, Z. Xu, J. Liu, B. Tang and X. Wang, Chem. Eng. J., 2017, 325, 396–403 CrossRef.
- N. Afsarimanesh, A. nag, S. Sarkar, G. S. Sabet, T. Han and S. C. Mukhopadhyay, Sens. Actuators, A, 2020, 315, 112355 CrossRef CAS.
- U. Stren, Nature, 2021, 591, 685 CrossRef PubMed.
- X. Huang, W. Go, S. Liu, Y. Li, Y. Qiu, H. Fang, G. Yang, K. Zhu, Z. Yin and Z. Li, Adv. Funct. Mater., 2022, 32, 2109109 CrossRef CAS.
- X. Wang, Y. Deng, P. Jiang, X. Chen and H. Yu, Microsyst. Nanoeng., 2022, 8, 113 CrossRef CAS PubMed.
- I. Hogas, C. Fosalau and C. Z, IEEE, 2016, 576–580 Search PubMed.
- Y.-H. Yu, C.-C. M. Ma, C.-C. Teng, Y.-L. Huang, S.-H. Lee, I. Wang and M.-H. Wei, Mater. Chem. Phys., 2012, 136, 334–340 CrossRef CAS.
- Q. Zhao, M. Zhao, J. Qiu, W. Y. Lai, H. Pang and W. Huang, Small, 2017, 13, 1701091–1704048 CrossRef PubMed.
- X. Dong, Y. Wei, S. Chen, Y. Lin, L. Liu and J. Li, Compos. Sci. Technol., 2018, 155, 108–116 CrossRef CAS.
- G. Wang, L. Hao, X. Zhang, S. Tan, M. Zhou, W. Go and G. Ji, J. Colloid Interface Sci., 2022, 607, 89–99 CrossRef CAS PubMed.
- Y. Lu, J. Jiang, S. Yoon, K.-S. Kim, J.-H. Kim, S. Park, S.-H. Kim and L. Piao, ACS Appl. Mater. Interfaces, 2018, 10, 2093–2104 CrossRef CAS PubMed.
- P. Hashemi, M. Mehranpour and I. Ghasemi, Polym. Compos., 2021, 42, 1440–1450 CrossRef CAS.
- K. Liu, W. Liu, W. Li, Y. Duan, K. Zhou, S. Zhang, S. Ni, T. Xu, H. Du and C. Si, Adv. Compos. Hybrid Mater., 2022, 1–12 Search PubMed.
- L. Manjakkal, D. Szwagierczak and R. Dahiya, Prog. Mater. Sci., 2020, 109, 100635 CrossRef CAS.
- J. An, Y. Ma, M. He, J. Yan, C. Zhang, X. Li, P. Shen, S. Luo and Y. Gao, Sens. Actuators, A, 2020, 311, 112081 CrossRef CAS.
- S. W. Kim, S. Zhang, D. Y. Park, C.-W. Lee, Y.-H. Ko, H. Yang, Y. Xiao, G. Chen and M. Li, J. Mater. Chem. C, 2018, 6, 7207–7218 RSC.
- J. H. Choi, M. G. Shin, Y. Jung, D. H. Kim and J. S. Ko, Micromachines, 2020, 11, 156 CrossRef PubMed.
- W.-W. Kong, C.-G. Zhou, K. Dai, L.-C. Jia, D.-X. Yan and Z.-M. Li, Composites, Part B, 2021, 225, 109275 CrossRef CAS.
- Y.-H. Yu, C.-C. M. Ma, C.-C. Teng, Y.-L. Huang, S.-H. Lee, I. Wang and M.-H. Wei, Mater. Chem. Phys., 2012, 136, 334–340 CrossRef CAS.
- M.-Y. Liu, C.-Z. Hang, X.-Y. Wu, L. Y. Zhu, X.-H. Wen, Y. Wang, X.-F. Zhao and H.-L. Lu, Nanotechnolgy, 2022, 33, 255501 CrossRef PubMed.
- Y. Li, S. Wang, C.-c. Xiao, Y. Yang, B.-w. Deng, B. Yin, K. Ke and M.-b. Yang, J. Mater. Chem. C, 2020, 8, 4040–4048 RSC.
- L. Zhang, X. Zhang, L. Xu, D. Wang, X. Lu and A. Zhang, Chem. Eng. J., 2022, 434, 134751 CrossRef CAS.
- C. b. Huang, Y. Yao, V. Montes-García, M. A. Stoeckel, M. Von Holst, A. Ciesielski and P. Samori, Small, 2021, 17, 2007593 CrossRef CAS PubMed.
- Y. Li, L. Shi, Y. Cheng, R. Wang and J. Sun, Chem. Eng. J., 2023, 455, 140763 CrossRef CAS.
- K. Singh, S. Sharma, B. Singh, M. Gupta and C. Tripathi, Thin Solid Films, 2022, 761, 139540 CrossRef CAS.
- A. Erdem, E. Yildiz, H. Senturk and M. Maral, J. Pharm. Biomed. Anal., 2023, 115385 CrossRef CAS PubMed.
- Y. K. Choi, T. K. Kim, J. H. Song, B. K. Jung, W. Kim, J. H. Bae, H. J. Choi, J. Kwak, J. W. Shim and S. J. Oh, Nanoscale, 2023, 15, 7980–7990 RSC.
- G. Alberti, C. Zanoni, V. Losi, L. R. Magnaghi and R. Biesuz, Chemosensors, 2021, 9, 108 CrossRef CAS.
- S. Kulkarni Vishakha, D. Butte Kishor and S. Rathod Sudha, Int. J. Res. Pharm. Biomed. Sci., 2012, 3, 1597–1613 CAS.
- S. Seyedin, J. M. Razal, P. C. Innis, A. Jeiranikhameneh, S. Beirne and G. G. Wallace, ACS Appl. Mater. Interfaces, 2015, 7, 21150–21158 CrossRef CAS PubMed.
- V. Eom, R. Jaisutti, H. Lee, W. Lee, J.-S. Heo, J.-Y. Lee, S. K. Park, Y.-H. Kim, L. Guo and Y. Gao, ACS Appl. Mater. Interfaces, 2017, 9, 10190–10197 CrossRef PubMed.
- H. Shen, H. Ke, J. Feng, C. Jiang, Q. Wei and Q. Wang, Nanomaterials, 2021, 11, 2333 CrossRef CAS PubMed.
- W. Shi, G. Han, Y. Chang, H. Song, W. Hou and Q. Chen, ACS Appl. Mater. Interfaces, 2020, 12, 45373–45382 CrossRef CAS PubMed.
- X. Xu, S. Wu, J. Cui, L. Yang, K. Wu, X. Chen and D. Sun, Composites, Part B, 2021, 211, 108665 CrossRef CAS.
- F. Jin, D. Lv, W. Shen, W. Song and R. Tan, Sens. Actuators, A, 2022, 337, 113412 CrossRef CAS.
- I. A. Rashid, I. Saif, M. Usama, M. Umer, A. Javid, Z. A. Rehan and U. Zubair, Sens. Actuators, A, 2022, 346, 113836 CrossRef CAS.
- T. N. Lam, G. S. Lee, B. Kim, H. D. Xuan, D. Kim, S. I. Yoo and J. Yoon, Compos. Sci. Technol., 2021, 210, 108811 CrossRef CAS.
- J. H. Song, Y. G. Kim, Y. Cho, S. Hong, J. Y. Choi, M. S. Kim and S. H. Ahn, Adv. Mater. Technol., 2023, 2201112 CrossRef CAS.
- A. Ciampaglia, R. Ciardiello, F. Cesano, G. Belingardi and V. Brunella, Compos. Struct., 2023, 304, 116373 CrossRef CAS.
- M. Njuguna, C. Yan, N. Hu, J. Bell and P. Yarlagadda, Composites, Part B, 2012, 43, 2711–2717 CrossRef CAS.
- Ö. Erdem, E. Derin, S. Zeibi Shirejini, K. Sagdic, E. G. Yilmaz, S. Yildiz, G. A. Akceoglu and F. Inci, Adv. Mater. Technol., 2022, 7, 2100572 CrossRef.
- X. Chen, F. Wang, L. Shu, X. Tao, L. Wei, X. Xu, Q. Zeng and G. Huang, Mater. Des., 2022, 221, 110926 CrossRef CAS.
- R. Zhang, P. Pan, Q. Dai, X. Yang, Z. Yang, J. Wei, J. Liu and Q. Yuan, J. Mater. Sci.: Mater. Electron., 2018, 29, 5589–5596 CrossRef CAS.
- Y. Zheng, Y. Li, K. Dai, M. Liu, K. Zhou, G. Zheng, C. Liu and C. Shen, Composites, Part A, 2017, 101, 41–49 CrossRef CAS.
- W. Li, F. Xu, W. Liu, Y. Gao, K. Zhang, X. Zhang and Y. Qiu, Composites, Part A, 2018, 108, 107–113 CrossRef CAS.
- T. Yan, Z. Wang and Z.-J. Pan, Curr. Opin. Solid State Mater. Sci., 2018, 22, 213–228 CrossRef CAS.
- X. Zhang, G. Yang, L. Zong, M. Jiang, Song, C. Ma, T. Zhang, Y. Duan and J. Zhang, ACS Appl. Mater. Interfaces, 2019, 12, 1378–1386 CrossRef PubMed.
- H. Lee, M. J. Glasper, X. Li, J. A. Nychka, J. Batcheller, H.-J. Chung and Y. Chen, J. Mater. Sci., 2018, 53, 9026–9033 CrossRef CAS.
- R. Sun, L. Gao, F. Liu, H. Su, L. Wu, Z. Zou, L. Wu, H. Zhang and C. Liao, Carbon, 2022, 194, 185–196 CrossRef CAS.
- S.-S. Xue, Z.-H. Tang, W.-B. Zhu, Y.-Q. Li, P. Huang and S.-Y. Fu, Composites Communications, 2022, 29, 100987 CrossRef.
- S. Sun, Y. Liu, X. Chang, Y. Jiang, D. Wang, C. Tang, S. He, M. Wang, L. Guo and Y. Gao, J. Mater. Chem. C, 2020, 8, 2074–2085 RSC.
- R. R. Nair, P. Blake, A. N. Grigorenko, K. S. Novoselov, T. J. Booth, T. Stauber, N. M. Peres and A. K. Geim, Science, 2008, 320, 1308 CrossRef CAS PubMed.
- A. Ciampaglia, R. Ciardiello, F. Cesano, G. Belingardi and V. Brunella, Composite Structures, 2023, 304, 116373 CrossRef CAS.
- M. Liu, Y. Sheng, C. Huang, Y. Zhou, L. Jiang, M. Tian, S. Chen, S. Jerrams, F. Zhou and J. Yu, Composites, Part A, 2023, 164, 107296 CrossRef CAS.
- M. Sun, P. Li, H. Qin, N. Liu, H. Ma, Z. Zhang, J. Li, B. Lu, X. Pan and L. Wu, Chem. Eng. J., 2023, 454, 140459 CrossRef CAS.
- P. Zhan, Y. Jia, W. Zhai, G. Zheng, K. Dai, C. Liu and C. Shen, Composites, Part A, 2023, 107431 CrossRef CAS.
- X. Wang, M. Qu, K. Wu, D. W. Schubert and X. Liu, Adv. Compos. Hybrid Mater., 2023, 6, 63 CrossRef CAS.
- T. Liu, Z. He, H. Liu, J. Yang, S. Zhang, J. Yu, M. Ji, C. Zhu and J. Xu, ACS Appl. Mater. Interfaces, 2021, 13, 18100–18109 CrossRef CAS PubMed.
- J. Xia, S. Khaliliazar, M. M. Hamedi and S. Sonkusale, MRS Bull., 2021, 46, 502–511 CrossRef CAS.
- T. Wang, Z. Ouyang, F. Wang and Y. Liu, SN Appl. Sci., 2020, 2, 1–22 Search PubMed.
- H. Li and Z. Du, ACS Appl. Mater. Interfaces, 2019, 11, 45930–45938 CrossRef CAS PubMed.
- A. D. La Rosa and S. A. Grammatikos, Fibers, 2019, 7, 101 CrossRef CAS.
- Y. Zhang, W. Zhang, G. Ye, Q. Tan, Y. Zhao, J. Qiu, S. Qi, X. Du, T. Chen and N. Liu, Adv. Mater. Technol., 2020, 5, 1900880 CrossRef CAS.
- B. Choi, J. Lee, H. Han, J. Woo, K. Park, J. Seo and T. Lee, ACS Appl. Mater. Interfaces, 2018, 10, 36094–36101 CrossRef CAS PubMed.
- Y. Zhang, W. Zhang, G. Ye, Q. Tan, Y. Zhao, J. Qiu, S. Qi, X. Du, T. Chen and N. Liu, Adv. Mater. Technol., 2020, 5, 1900880 CrossRef CAS.
- M. Ren, Y. Zhou, Y. Wang, G. Zheng, K. Dai, C. Liu and C. Shen, Chem. Eng. J., 2019, 360, 762–777 CrossRef CAS.
- J. Gao, X. Wang, W. Zhai, H. Liu, G. Zheng, K. Dai, L. Mi, C. Liu and C. Shen, ACS Appl. Mater. Interfaces, 2018, 10, 34592–34603 CrossRef CAS PubMed.
- J. Gao, X. Wang, W. Zhai, H. Liu, G. Zheng, K. Dai, L. Mi, C. Liu and C. Shen, ACS Appl. Mater. Interfaces, 2018, 10, 34592–34603 CrossRef CAS PubMed.
- X. Wu, Y. Han, X. Zhang and C. Lu, ACS Appl. Mater. Interfaces, 2016, 8, 9936–9945 CrossRef CAS PubMed.
- R. Wang, N. Jiang, J. Su, Q. Yin, Y. Zhang, Z. Liu, H. Lin, F. A. Moura, N. Yuan and S. Roth, Adv. Funct. Mater., 2017, 27, 1702134 CrossRef.
- H. Liu, L.-l. Zhu, Y. He and B.-w. Cheng, Mater. Des., 2017, 113, 254–263 CrossRef CAS.
- Z. Cao, R. Wang, T. He, F. Xu and J. Sun, ACS Appl. Mater. Interfaces, 2018, 10, 14087–14096 CrossRef CAS PubMed.
- S. Cai, C. Xu, D. Jiang, M. Yuan, Q. Zhang, Z. Li and Y. Wang, Nano Energy, 2022, 93, 106904 CrossRef CAS.
- M. Zhu, J. Li, J. Yu, Z. Li and B. Ding, Angew. Chem., 2022, 134, e202200226 CrossRef.
- J. Lee, S. Shin, S. Lee, J. Song, S. Kang, H. Han, S. Kim, S. Kim, J. Seo and D. Kim, ACS Nano, 2018, 12, 4259–4268 CrossRef CAS PubMed.
- O. Atalay, W. R. Kennon and M. D. Husain, Sensors, 2013, 13, 11114–11127 CrossRef PubMed.
- X. Li, T. Hua and B. Xu, Carbon, 2017, 118, 686–698 CrossRef CAS.
- H. Souri and D. Bhattacharyya, Sens. Actuators, A, 2019, 285, 142–148 CrossRef CAS.
- C. Zhu, R. Guo, X. Chen, E. Chalmers, X. Liu, Y. Wang, B. B. Xu and X. Liu, Adv. Sci., 2020, 7, 2109109 Search PubMed.
- H. Liu, Q. Li, Y. Bu, N. Zhang, C. Wang, C. Pan, L. Mi, Z. Guo, C. Liu and C. Shen, Nano Energy, 2019, 66, 104143 CrossRef CAS.
- G. Cai, B. Hao, L. Luo, Z. Deng, R. Zhang, J. Ran, X. Tang, D. Cheng, S. Bi and X. Wang, ACS Appl. Mater. Interfaces, 2020, 12, 29717–29727 CAS.
- X. Xie, H. Huang, J. Zhu, J. Yu, Y. Wang and Z. Hu, Composites, Part A, 2020, 135, 105932 CrossRef CAS.
- J. Pan, B. Hao, W. Song, S. Chen, D. Li, L. Luo, Z. Xia, D. Cheng, A. Xu and G. Cai, Composites, Part B, 2020, 183, 107683 CrossRef CAS.
- X. Li, H. Hu, T. Hua, B. Xu and S. Jiang, Nano Res., 2018, 11, 5799–5811 CrossRef CAS.
- H. Sun, K. Dai, W. Zhai, Y. Zhou, J. Li, G. Zheng, B. Li, C. Liu and C. Shen, ACS Appl. Mater. Interfaces, 2019, 11, 36052–36062 CrossRef CAS PubMed.
- H. Li and Z. Du, ACS Appl. Mater. Interfaces, 2019, 11, 45930–45938 CrossRef CAS PubMed.
- J. J. Feng, X. Wang, Z. Lv, J. Qu, X. Lu, Q. Wei and Q. Wang, Adv. Mater. Technol., 2020, 5, 2000560 CrossRef.
- R. Xu, X. Zheng, M. Chen, L. Sun, J. Chen, F. Wang and Y. Ma, Appl. Sci., 2020, 10, 6230 CrossRef CAS.
- X. Liu, C. Tang, X. Du, S. Xiong, S. Xi, Y. Liu, X. Shen, Q. Zheng, Z. Wang and Y. Wu, Mater. Horiz., 2017, 4, 477–486 RSC.
- X. Liu, D. Liu, J.-h. Lee, Q. Zheng, X. Du, X. Zhang, H. Xu, Z. Wang, Y. Wu and X. Shen, ACS Appl. Mater. Interfaces, 2018, 11, 2282–2294 CrossRef PubMed.
- S. Wang, H. Ning, N. Hu, Y. Liu, F. Liu, R. Zou, K. Huang, X. Wu and S. Weng, Adv. Mater. Interfaces, 2020, 7, 1901507 CrossRef.
- T. Zhu, L. Liu, J. Huang, S. Li, Y. Lei, W. Cai, Y. Lai and H. Li, J. Mater. Sci. Technol., 2023, 138, 108–116 CrossRef.
- T. Zhu, L. Liu, J. Huang, S. Li, Y. Lei, W. Cai, Y. Lai and H. Li, J. Mater. Sci. Technol., 2023, 138, 108–116 CrossRef.
- Y. Zheng, Y. Li, Y. Zhou, K. Dai, G. Zheng, B. Zhang, C. Liu and C. Shen, ACS Appl. Mater. Interfaces, 2019, 12, 1474–1485 CrossRef PubMed.
- D. Lu, S. Liao, Y. Chu, Y. Cai, Q. Wei, K. Chen and Q. Wang, Adv. Fiber Mater., 2023, 5, 223–234 CrossRef CAS.
- Z. Zhou, W. Zhang, J. Zhang, Y. Zhang, X. Yin and B. He, Sens. Actuators, A, 2023, 349, 114004 CrossRef CAS.
- D. W. Kim, S. W. Kim, G. Lee, J. Yoon, S. Kim, J.-H. Hong, S.-C. Jo and U. Jeong, Light: Sci. Appl., 2023, 12, 61 CrossRef CAS PubMed.
- B. Wu, Z. Lin, Y. Liang, Z. Zhou and H. Lu, Mechanical Systems and Signal Processing, 2023, 186, 109855 CrossRef.
- T. Tan, A. A. Gatti, B. Fan, K. G. Shea, S. L. Sherman, S. D. Uhlrich, J. L. Hicks, S. L. Delp, P. B. Shull and A. S. Chaudhari, npj Digital Medicine, 2023, 6, 46 CrossRef PubMed.
- C. Gao, Y. Liu, F. Gu, Z. Chen, Z. Su, H. Du, D. Xu, K. Liu and W. Xu, Chem. Eng. J., 2023, 460, 141769 CrossRef CAS.
- S. Yang, W. Yang, R. Yin, H. Liu, H. Sun, C. Pan, C. Liu and C. Shen, Chem. Eng. J., 2023, 453, 139716 CrossRef CAS.
- T. Zhu, L. Liu, J. Huang, S. Li, Y. Lei, W. Cai, Y. Lai and H. Li, J. Mater. Sci. Technol., 2023, 138, 108–116 CrossRef.
- Z. Shen, F. Liu, S. Huang, H. Wang, C. Yang, T. Hang, J. Tao, W. Xia and X. Xie, Biosens. Bioelectron., 2022, 114298 CrossRef CAS PubMed.
- Z. Shen, F. Liu, S. Huang, H. Wang, C. Yang, T. Hang, J. Tao, W. Xia and X. Xie, Biosens. Bioelectron., 2022, 114298 CrossRef CAS PubMed.
- Y. Liu, M. Yu, B. Xia, S. Wang, M. Wang, M. Chen, S. Dai, T. Wang and T. T. Ye, IEEE Internet Things J., 2021, 8, 16486–16497 Search PubMed.
- X. He, J. Gu, Y. Hao, M. Zheng, L. Wang, J. Yu and X. Qin, Chem. Eng. J., 2022, 450, 137937 CrossRef CAS.
- D. Lu, Y. Chu, S. Liao, W. Li, Y. Cai, Q. Wei and Q. Wang, Compos. Sci. Technol., 2022, 230, 109778 CrossRef CAS.
- F. Sun, Y. Zhu, C. Jia, T. Zhao, L. Chu and Y. Mao, J. Energy Chem., 2023, 79, 477–488 CrossRef.
- Q. Zhang, T. Jin, J. Cai, L. Xu, T. He, T. Wang, Y. Tian, L. Li, Y. Peng and C. Lee, Adv. Sci., 2022, 9, 2103694 CrossRef CAS PubMed.
- J. Tang, Y. Wu, S. Ma, T. Yan and Z. Pan, ACS Appl. Mater. Interfaces, 2023, 15, 7392–7404 CrossRef CAS PubMed.
- A. I. Faisal, S. Majumder, T. Mondal, D. Cowan, S. Naseh and M. J. Deen, Sensors, 2019, 19, 2629 CrossRef PubMed.
- Y. Zhao, Z. Lin, S. Dong and M. Chen, Opt. Laser Technol., 2023, 161, 109227 CrossRef.
- H. Kim, A. Shaqeel, S. Han, J. Kang, J. Yun, M. Lee, S. Lee, J. Kim, S. Noh and M. Choi, ACS Appl. Mater. Interfaces, 2021, 13, 39868–39879 CrossRef CAS PubMed.
- S.-H. Zhang, F.-X. Wang, J.-J. Li, H.-D. Peng, J.-H. Yan and G.-B. Pan, Sensors, 2017, 17, 2621 CrossRef PubMed.
|
This journal is © The Royal Society of Chemistry 2023 |
Click here to see how this site uses Cookies. View our privacy policy here.