DOI:
10.1039/D3AN01324C
(Paper)
Analyst, 2024,
149, 212-220
A chimeric hairpin DNA aptamer-based biosensor for monitoring the therapeutic drug bevacizumab†
Received
2nd August 2023
, Accepted 8th November 2023
First published on 21st November 2023
Abstract
The accurate and rapid detection of specific antibodies in blood is very important for efficient diagnosis and precise treatment. Conventional methods often suffer from time-consuming operations and/or a narrow detection range. In this work, for the rapid determination of bevacizumab in plasma, a series of chimeric hairpin DNA aptamer-based probes were designed by the modification, labeling and theoretical computation of an original aptamer. Then, the dissociation constant of the modified hairpin DNA to bevacizumab was measured and screened using microscale thermophoresis. The best chimeric hairpin DNA aptamer-based probe was then selected, and a one-step platform for the rapid determination of bevacizumab was constructed. This strategy has the advantages of being simple, fast and label-free. Because of the design and screening of the hairpin DNA, as well as the optimization of the concentration and electrochemical parameters, a low detection limit of 0.37 pM (0.054 ng mL−1) with a wide linear range (1 pM–1 μM) was obtained. Finally, the rationally constructed biosensor was successfully applied to the determination of bevacizumab in spiked samples, and it showed good accuracy and precision. This method is expected to truly realize accurate and rapid detection of bevacizumab and provides a new idea for the precise treatment of diseases.
1. Introduction
Antibodies have attracted great attention due to their numerous applications in clinical diagnosis and therapy. Some anti-double strand DNA (dsDNA) antibodies are valuable biomarkers for the diagnosis of autoimmune diseases.1 On the other hand, in the last decade therapeutic antibodies have been increasingly used for the treatment of malignant tumors, autoimmune diseases, and so on.2 For example, the vascular endothelial growth factor-A (VEGF-A)-targeting monoclonal antibody bevacizumab (Avastin®), the first approved angiogenesis inhibitor, has been successfully used for treating many types of malignant tumors.3,4 The pharmacokinetic profiles of bevacizumab have been systematically studied, and in all clinical trials, bevacizumab showed low clearance, limited central chamber volume as well as a long half-life, which allows the targeted treatment of bevacizumab plasma levels to be maintained through a series of dosed schedules (e.g., once every two or three weeks).5 However, individual values after bevacizumab administration were scattered, with an average difference of four times between the lowest and highest concentrations per administration (70 to 300 ng mL−1).6 Therefore, it is of great significance to monitor the blood concentration of bevacizumab in order to effectively improve its therapeutic effect and ensure precise treatment.
However, the currently dominant ELISA detection strategies often suffer from drawbacks, such as a tedious operation process or the use of expensive but easily inactivated reagents in the detection of antibodies in real blood samples.7 Consequently, simple, fast but effective antibody detection methods are urgently needed to achieve accurate and personalized diagnosis and treatment. Aptamers are single-stranded oligonucleotides, and screened by systematic evolution of ligands by exponential enrichment (SELEX), which can bind to a various of targets with high specificity and affinity.8,9 Aptamers exhibit a number of merits over antibodies, including cost-effectiveness, programmable replication, and high physicochemical stability.10,11 In particular, aptamers typically undergo significant spatial conformational changes when bound to targets, which provides great flexibility for designing novel biosensing strategies with a high sensitivity and selectivity.10 After nearly three decades of development, the effectiveness of aptamers in clinical diagnosis, targeted therapy and other fields has gained more recognition and attention.12,13 Among them, aptamer-based affinity biosensing strategies have aroused sustained interest for detecting a variety of targets, such as metal ions, active molecules, protein biomarkers and viruses or whole cells.
Hairpin DNA is a stable stem-loop DNA secondary structure formed by the complementary pairing of neck DNA bases.14,15 The chemical nature of the oligonucleotides allows their easy synthesis and modification. Consequently, many studies have been reported on the engineering of hairpin DNA structures with advanced bioavailability, regulation ability, and multifunctional properties.16 By embedding specific aptamers, the hairpin DNA probes have a high hybridization specificity and selectivity for target molecules such as DNA/RNA, active molecules and proteins.17 Plaxco and co-workers have been studying electrochemical switching biosensors based on hairpin DNA or peptide spatial conformation changes and have successfully applied them to the direct and rapid detection of antibodies in whole blood.18 In recent years, electrochemical switching biosensors have been shown to be sensitive and selective enough to analyze complex samples such as plasma or serum, and the related studies have been extensively reported.19–32 However, most of these hairpin DNA probes are used to detect DNA or RNA using the principle of complementary base pairing.20,22,25,27,29–32 If we want to detect other non-nucleic acid targets using hairpin DNA probes, the incorporation of a highly specific aptamer is still necessary.
The hairpin DNA aptamer chimera (Ach) probe might be a promising solution to the problem, because it maintains not only the structural flexibility of hairpin DNA, but also its high binding affinity towards the target. Hence, in this study, an Ach probe for bevacizumab was designed and optimized based on its target affinity measured by microscale thermophoresis (MST). Then, a simple one-step electrochemical switch biosensor was constructed (Ach biosensor) for the determination of bevacizumab. As shown in Scheme 1, when the Ach is modified on the electrode interface, the methylene blue (MB) probe is very close to the sensing interface, resulting in an obvious electrochemical signal. Once bevacizumab is introduced, the bevacizumab binds with the Ach to induce spatial conformation changes of the Ach probe, and the electroactive molecule moves away from the electrode interface resulting in signal reduction, thus achieving one-step rapid detection. In comparison with the previously reported switch-based detection strategies,18,33,34 this Ach biosensor does not require additional labeled antigens or other affinity ligands. Therefore, it is much simpler in terms of operation and structure design, and it is expected to achieve rapid and effective detection of therapeutic antibodies in biological fluids.
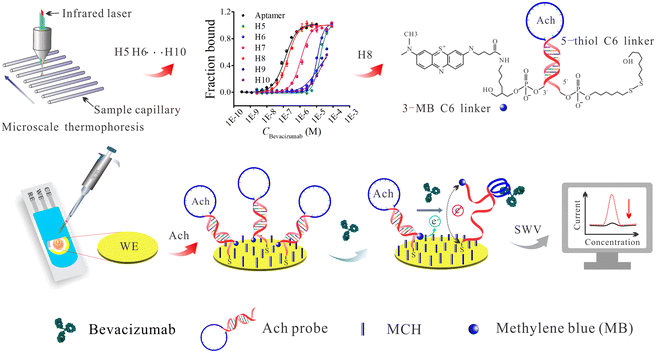 |
| Scheme 1 Schematic diagram of the Ach probe based biosensing strategy for single-step electrochemical detection of bevacizumab. | |
2. Experimental
2.1. Materials and instrumentation
The 6-carboxyfluorescein (6-FAM) modified DNA molecules and methylene blue (MB) modified hairpin DNA were custom-made by Shanghai Sangon Biotech (Shanghai, China). The rituximab injection solution (10 mg mL−1), trastuzumab solid powder (100 mg) and bevacizumab injection solution (25 mg mL−1) were provided by Shanghai Roche Pharmaceuticals (Shanghai, China). The infliximab powder (100 mg) was obtained from Cilag AG (Schaffhausen, Switzerland). Human IgG (≥95%), Tween 20, bovine serum albumin (BSA), 6-mercaptohexanol (MCH) and tris-(2-carboxyethyl) phosphine hydrochloride (TCEP) were obtained from Sigma-Aldrich (Shanghai, China). Sodium phosphate buffer solutions (PBS) (10 mM, pH 7.4) were purchased from Solarbio Science & Technology (Beijing, China). All the deionized water (18.2 MΩ cm) was produced in our own laboratory with a laboratory purification system (E-well Biotechnology). Instrumentation details are given in the ESI.†
All the experiments were performed in accordance with the “Ethical Review of Life Sciences and Medical Research Involving Human Beings” guidelines, issued by the National Health Commission, Ministry of Education, Ministry of Science and Technology of China. This study has been approved by the ethics committee at Jinan University. Informed consent was obtained from human participants of this study.
2.2. Design and screening of the Ach probes
Hairpin DNAs with stems containing different numbers of DNA base pairs (bps) ranging from 5 to 10 (underlined regions) were designed and their detail base sequences are listed in Table S1 (ESI).† The original aptamer for bevacizumab was reported in a previous study.35 The bevacizumab aptamer sequence (blue letters) was embedded in the DNA hairpin structure. Moreover, a 6-FAM fluorescent group was labeled at the 3 terminal of each hairpin sequence to obtain an MST signal output. Subsequently, each hairpin DNA sequence designed (H5, H6, H7, H8, H9 and H10) was incubated with bevacizumab separately, and their corresponding dissociation rate constant (Kd) values were measured by MST. Then, these Kd values and MST fitting curves were compared with the Kd value of the original bevacizumab aptamer to select the optimal hairpin DNA for further experiments.
2.3. Pretreatment and modification of gold electrodes
The pretreatment process of the gold electrodes was based on previous research with a slight modification.36 Briefly, the gold screen printed electrode (4.0 mm) was first immersed in fresh piranha solution (concentrated sulfuric acid (H2SO4) is mixed with 30% hydrogen peroxide at a ratio of 3 to 1) for 3–5 min, followed by washing several times with deionized water. Then, the electrode was electrochemically cleaned in 0.5 M H2SO4 with cyclic voltammetry (CV) scanning from – 0.1 V to 1.35 V (scan rate: 0.5 V s−1) until a steady voltammogram was obtained. On the other hand, 100 μL (5 μM) of a sulfhydryl and MB-modified hairpin DNA were mixed with 5 μL (100 mM) of TCEP in a 1.8 mL microcentrifuge tube and then incubated in a dark place at room temperature for 60 minutes to reduce the disulfide bonds. Subsequently, the prepared electrodes were incubated with 10 μL of 5 μM hairpin DNA (pretreated with TCEP) at room temperature followed by immersion in an aqueous solution of 2 mM MCH (10 μL) for 2 h at room temperature to block the remaining active sites. Next, the prepared electrode was further washed with deionized water, and the electrode was soaked in PBS and stored in a dark place at 4 °C.
2.4. Concentration optimization of the hairpin DNA probe
The stock solutions of the configured Ach probes were diluted with deionized water to different concentrations (2.0 μM, 1.0 μM, 500 nM, 200 nM and 100 nM). Samples (50 μL) of each of the diluted solutions were added separately onto the working surfaces of the five electrodes. Next, 10 μL of 2 mM MCH solution was dropped onto the electrode surface to seal the remaining active sites to reduce the non-specific binding. Then, the unreacted MCH molecules were washed several times with PBS and deionized water. Finally, square-wave voltammetry (SWV) was employed to measure the initial signal, and the signal intensity generated by different concentrations of DNA probes was compared to obtain the optimal concentration of the DNA probes.
2.5. Electrochemical characterization and measurement of the Ach biosensor
In these experiments, CV and electrochemical impedance spectroscopy (EIS) were mainly utilized to characterize the electrode preparation process. The electrochemical test solution consisted of 5 mM [Fe(CN)6]3−/4− and 0.1 M KCl. The initial potential of the CV was 0.5 V, the high/low potential were 0.5 and −0.2 V, and the other parameters were set by default. The parameters of the EIS test were set as follows: the high and low frequency were 100
000 and 0.1 Hz, respectively, the disturbance potential (amplitude) was 0.01 V, and the other parameters were set by default. The SWV was used for detection in an electrochemical window from −0.5 V to −0.1 V, and the amplitude, step potential and frequency were set at 25 mV, 2 mV and 25 Hz, respectively.
3. Results and discussion
3.1. Optimization of the temperature and time for the binding between the DNA aptamer and bevacizumab
Prior to the screening experiments, the linear DNA aptamer was allowed to interact with bevacizumab, and the incubation temperature was optimized using the MST binding assay. Because the sequence of the loop in the hairpin DNA was identical to that of the bevacizumab aptamer, the optimal temperature obtained for the linear aptamer with bevacizumab can be used for the binding of the hairpin DNA to bevacizumab. In these experiments, the concentration of bevacizumab was 10 μM, whereas the DNA aptamer was 100 nM (this value was based on the optimization of the detection performance of the MST instrument, whose optimization process and results are shown in Fig. S1, ESI†). The results of the MST temperature optimization are shown in Fig. S2 (ESI).† The results showed that as the temperature increased from 22 to 35 °C, the MST curve of the complexes formed by the aptamer and bevacizumab increased, whereas the MST curve of the single aptamer gradually decreased, and the relative fluorescence (FL) value of the aptamer in the presence of bevacizumab gradually decreased, suggesting that the binding was gradually weakened. The default temperature in binding experiments was normally the temperature of the human body, which is about 37 °C. However, according to these results, a lower temperature was more conducive for binding, so that 22 °C was chosen for the subsequent MST experiments (the minimum temperature control of the instrument is 22 °C).
To obtain an accurate and effective incubation time for the hairpin DNAs with bevacizumab, different incubation times (15, 30, 45, or 60 min) for the DNA aptamer and bevacizumab were investigated to determine a reference value. As shown in Fig. S3 (ESI),† only 15 min of the incubation time led to a slightly lower binding, and the fitted curves for the other four incubation times were very close. Therefore, in the subsequent MST experiments, an incubation time of 30 min was selected, which not only saved time, but also ensured an effective binding between the DNA aptamer and bevacizumab.
3.2. Screening of the H5–H10 hairpin DNAs
Under the optimized conditions such as initial concentration of aptamer (100 nM), incubation temperature (22 °C) and time (30 min), the screening of the hairpin DNAs was carried out (the sequence details are given in Table S1, ESI†). This experiment aimed to obtain a stable hairpin that also had a high affinity to bevacizumab. The affinity tests of the six hairpin DNAs towards bevacizumab were performed using MST, and the results are shown in Fig. 1. The binding of H9 and H10 to bevacizumab failed to fit an “S” shaped curve (Fig. 1C), even with the addition of a high concentration of bevacizumab. This indicated that the neck base sequences of H9 and H10 were maybe too long, and the binding force between the aptamer sequence in the loop and the bevacizumab was weaker than the complementary force from the H9 and H10 neck bps. These results were also in good agreement with the thermodynamic data obtained by simulation using online UNAFold software. For example, the Gibbs’ free energy, entropy, and enthalpy values of H9 and H10 were significantly lower than those of H8, H7, H6 and H5 (Fig. 1B). Then, with a reduction of the complementary bps in the neck of the hairpin DNA to eight, the affinity was significantly enhanced (32.5 μM to 56.3 nM), and it was close to that of the linear aptamer (Table 1). However, with a further reduction of the neck bps to seven, the affinity of the hairpin DNA to bevacizumab was found to decrease slightly, and with an additional reduction of the neck bps to six and five, the affinity of the hairpin DNAs to bevacizumab decreased further. The trend in binding affinity indicates that the presence of eight bps in the hairpin DNA is a critical value, and the structure of the hairpin was then relatively stable. In addition, it was concluded that the binding force between the aptamer and bevacizumab was slightly stronger than the hydrogen bonds of the eight bps, so that the hairpin DNA can sensitively open in the presence of bevacizumab. Finding this critical value was also the purpose of these experiments. When the amounts of neck bps were further decreased to seven or six, the hairpin structure may not be stable and it forms a variety of different spatial structures in the solution, which was not conducive to the binding of the aptamer sequence in the hairpin DNA to bevacizumab.
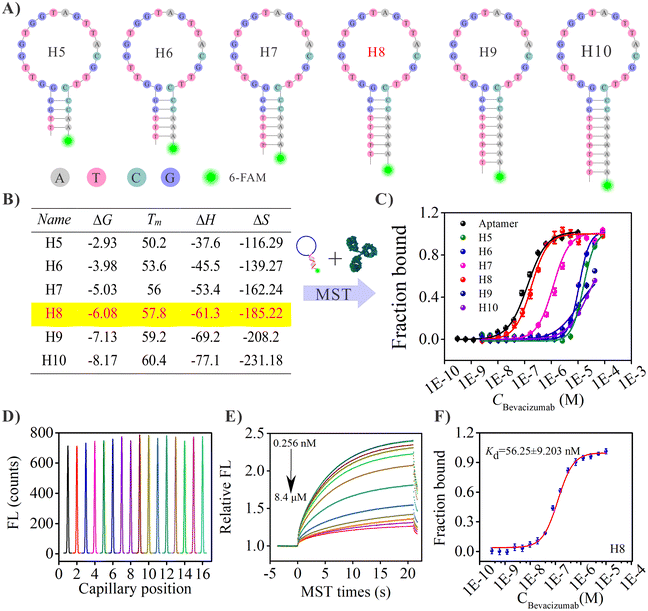 |
| Fig. 1 (A) The structures of the six hairpin DNA probes, and their neck complementary base pairs were 5, 6, 7, 8, 9 and 10, a 6-FAM was labeled on the 3 terminal of the hairpin DNAs for the MST screening. (B) Thermodynamic parameters for the six hairpin DNA probes. (C) MST screening of the six hairpin DNA probes. (D) The initial fluorescence signal of 16 concentration gradient (1 : 1 dilution) samples prior to MST testing. (E) The MST traces of the H8 hairpin DNA interacting with the bevacizumab complex under optimal conditions. (F) Fitting of the changes in the MST signals of H8 (red line) with the bevacizumab concentration to yield a Kd of 56.25 ± 9.20 nM. Related standard deviations (RSD) of 3 independent experiments. The concentrations of bevacizumab were in the range from 0.256 nM to 8.4 μM, whereas the concentrations of the hairpin DNAs (H5-H10) and the linear aptamer were fixed at 50 nM. | |
Table 1 The Kd values for the complexes between the aptamer or the hairpin DNAs and bevacizumab
Name |
Ligand name |
K
d
|
K
d confidence |
Aptamer ∼ Beva |
Aptamer |
5.51 × 10−8 |
4.89 × 10−9 |
H5 ∼ Beva |
H5 |
9.31 × 10−7 |
2.21 × 10−6 |
H6 ∼ Beva |
H6 |
8.45 × 10−7 |
1.10 × 10−6 |
H7 ∼ Beva |
H7 |
3.71 × 10−7 |
1.15 × 10−7 |
H8 ∼ Beva |
H8 |
5.63 × 10−8 |
9.20 × 10−9 |
H9 ∼ Beva |
H9 |
2.18 × 10−5 |
— |
H10 ∼ Beva |
H10 |
3.25 × 10−5 |
— |
Based on the previous results, H8 was selected as the capture/detection probe for bevacizumab, in the subsequent experiments. The affinity data for the linear aptamer and the hairpin DNAs: H5, H6, H7, H8, H9 and H10, interacting with bevacizumab are shown in Table 1. The Kd values indicated that the H8 affinity was the closest to that of the linear aptamer. For the selected hairpin DNA probe (H8), the initial fluorescence signals prior to the MST test and the MST curves for the 16 samples with increasing bevacizumab concentrations, are shown in Fig. 1D and E, respectively, whereas the Kd fitting curve is shown in Fig. 1F. The MST data for the interaction between the other hairpin DNA probes and bevacizumab are not shown.
3.3. Characterization of the Ach biosensor and verification of its feasibility
In order to verify the fabrication process of the Ach probe-based biosensor, the EIS, CV and SWV techniques were utilized to study electrochemical performance of the biosensing interface. Fig. 2A shows a schematic diagram of the preparation process of the biosensor. Fig. 2B shows the results of the EIS characterization for the different assembly processes of the biosensor, and the EIS curve (curve a) of a bare gold electrode was almost a straight line with slope of 1, which indicated that the bare electrode had been properly pretreated, and its electron transfer impedance (Rct) was almost negligible. After the Ach probe was fixed, the Rct value increased significantly (curve b) due to the steric hindrance and non-conductivity of the Ach probe, indicating that the Ach probe was successfully modified on the electrode interface. When the MCH reagent was further introduced into the screen printed gold electrode (SPGE)/Ach interface, the mercaptan reagent binds to the remaining active site on the surface of the gold electrode, which caused a slight increase in the impedance value of the biosensing interface (curve c). However, when the bevacizumab was further introduced into the system, the bevacizumab was bound to the Ach probe, and the steric hindrance caused by the large size of the bevacizumab hindered the electron transport at the electrode interface, resulting in an increase in the impedance value (curve c). Due to the extremely low concentration of bevacizumab, the charge transfer impedance change was extremely weak.
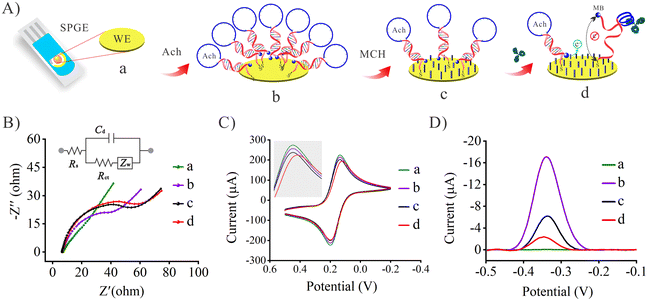 |
| Fig. 2 (A) Electrode process characterization and validation of the biosensor feasibility. The EIS (B) and CV characterization (C) for different modified electrodes (a, b, c and d) in [Fe(CN)6]3−/4− (5 mM) containing 0.1 M KCl. (D) The SWV response curves of bevacizumab (10 nM) for the different processes in 10 mM PBS. | |
Meanwhile, the CV technique was also performed to verify the fabrication processes of the biosensing interface. As shown in Fig. 2C, the electroactive probe [Fe(CN)6]3−/4− shows a couple of intense redox peaks on the pretreated gold electrode (curve a). The current intensity of the redox peaks decreased slightly after modifying the Ach probe. It is thought that most electrons can still transfered well in the non-dense nucleic acid network,38 so the change of voltammetric signal is very weak. After introducing MCH, the hydrophilic thiols and steric hindrance effect could hinder the [Fe(CN)6]3−/4− probes, and slightly decreased the peak current (curve c). When the bevacizumab was introduced into the biosensor incubation, the peak current was further reduced (curve d) because the large size of bevacizumab prevented the electroactive probes from approaching the electrode. The previous CV characterization results were in good agreement with the trend of the impedance signal, which proves the successful preparation of the biosensor.
In addition to the previous characterization of the electrode assembly process, the viability of the Ach probe-based biosensor for bevacizumab was further verified using SWV. As shown in Fig. 2D, curves a, b and c still represent the electrode assembly processes. Combined with Fig. 2A, it was inferred that curve a corresponded to the original bare SPGE, there were no Ach probes at this stage, and thus there was no signal (electroactive probe: MB). Curve b shows a strong decrease of the peak current from MB, with which most of the Ach probes were labeled. When coated on the electrode, the MCH competes for some of the Ach sites, so that the peak current decreases significantly and reaches a steady state (curve c). These particular results also demonstrated the successful assembly of the electrode surface. Next, 50 μL of bevacizumab (10 nM) were added to the biosensing system to investigate its feasibility for bevacizumab detection. After incubation for 30 min, the SWV test showed that the peak current decreased significantly (curve d). This was because the binding of bevacizumab to the Ach probe forced the spatial conformation of the probe to change, and the MB molecule at the end of the Ach probe was far away from the biosensing interface, and the voltammetry signal decreased.37 These results show that the Ach-based biosensor had been successfully assembled, and has good potential for future use in bevacizumab detection.
3.4. Optimization of the hairpin DNA probe concentration
The density of the hairpin DNA on the electrode surface is very important for the sensing performance. A low concentration of the hairpin DNA gave rise to a low capture efficiency for the target, and the encapsulation material occupied a large proportion of the electrode surface, leading to a large background impedance, which was not conducive to electron transport. There was steric hindrance between the hairpin DNA probes at a high concentration, which was not effective and resulted in probe waste. Therefore, it is highly necessary to optimize the concentration of the hairpin DNA. According to the preliminary results, the concentration of the hairpin DNA was at its optimum in the range from 100 to 2000 nM. Fig. S7 (ESI)† shows that when the concentration of the hairpin DNA was 1000 nM, the highest current signal can be obtained under the same SWV conditions, and this value was selected for use in further experiments. Using the formula in section S1.3 (ESI†) and further calculation, the actual density of the hairpin DNA at the electrode interface was found to be about 3.19 pmol mm−2.
3.5. Analytical performance of the Ach biosensor
3.5.1. Specificity and selectivity.
The specificity of a biosensor is the ability to assess unequivocally the analyte in complex mixtures containing structural analogs or other related substances. Monoclonal antibodies such as rituximab, trastuzumab, infliximab (10 μM), BSA (10 μM), human immunoglobulin (hIgG), bevacizumab (10 nM) and their mixtures dissolved in PBS were selected to evaluate the specificity of the proposed biosensor. As shown in Fig. 3A, the current signals generated by rituximab, trastuzumab, infliximab, hIgG, BSA and other proteins were almost equivalent to that of the blank, whereas bevacizumab, and the mixture containing bevacizumab, produced a current drop of almost the same intensity. This indicated that the sensor had good specificity for bevacizumab. In Fig. 3B, the histogram of the current intensity differences shows that the signals generated by the non-target proteins such as rituximab, trastuzumab, infliximab, hIgG and BSA were only slightly higher than those generated by the blank, whereas the current intensity differences generated by the target protein bevacizumab and the mixture were much higher than those of the non-target proteins. This clearly demonstrated that the sensor has a high specificity for bevacizumab.
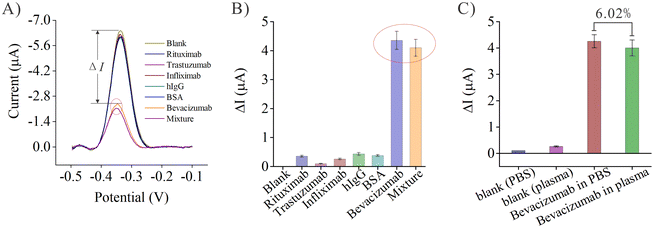 |
| Fig. 3 (A) The SWV current response curves of the different monoclonal antibodies (10 μM), BSA (10 μM), hIgG, the target bevacizumab (10 nM) and their mixture for specificity evaluation. (B) Histograms of the current intensity differences for the proteins and their mixture (ΔI). (C) The SWV current intensity differences for blank samples and bevacizumab (10 nM) in PBS and 10-fold diluted plasma for the selectivity evaluation. | |
Next, bevacizumab was dissolved in PBS and diluted healthy human plasma (the final concentration of bevacizumab was 10 nM in both mixtures). Both PBS and healthy human plasma were used as blank controls to evaluate the selectivity of the biosensor. As shown in Fig. 3C, the current intensity differences for PBS and blank plasma were very small, whereas the current intensity difference generated by bevacizumab in PBS was relatively high, indicating that the sensor had good selectivity for bevacizumab in PBS. The signal generated by bevacizumab in plasma was then tested. Similarly, the current intensity difference generated by the sensor in response to bevacizumab in plasma was almost as high as that generated by bevacizumab in PBS, with only a 6.02% difference. These results further demonstrated that the sensor is still highly selective for bevacizumab in a complex matrix such as plasma and can almost completely overcome the interference from the plasma matrix.
3.5.2. Sensitivity and linearity.
As shown in Fig. 4A, no significant decrease in the electrochemical signal was observed for a very low concentration (0.01 pM) of bevacizumab (dissolved in blank plasma). Then, the concentration of the target was gradually increased, and the linear range and sensitivity of the sensor was evaluated in the concentration range from 0.1 pM to 1 μM (Fig. 4B). The sensor was found to have a good linearity in the concentration range from 1 pM to 1 μM (Fig. 4C), whereas the signal saturation occurred in the very low and high concentration ranges. The fitted linear equation for the relationship between the current intensity and logarithmic concentration of bevacizumab was I (μA) = 7.75 × 10−7 log
c − 4.18 × 10−6 (R2 = 0.994). With a 98.3% confidence interval, the standard deviation σ for 10 blank samples was determined, and the detection limit of the biosensor was calculated to be 0.37 pM using the expression: LOD = 3σ/slope.
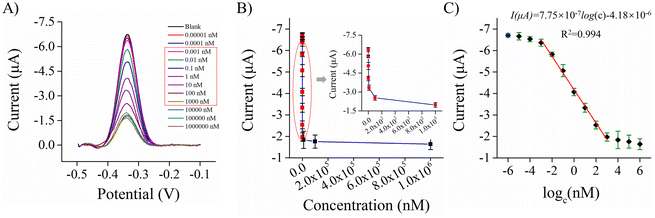 |
| Fig. 4 (A) The SWV responses of the biosensor at various concentrations of bevacizumab (the linear concentration range is shown in the red box, from 0.001 to 1000 nM). (B) The relationship between current intensity and bevacizumab concentration (inset: the linear range). (C) The linear relationship between the SWV current intensity and the logarithmic concentration of bevacizumab. | |
3.5.3. Regenerability, stability and repeatability.
The regenerability, stability and repeatability of the biosensor were also evaluated. Guanidine hydrochloride (6 M) was first used to remove bevacizumab from the biosensing interface at room temperature. After washing, PBS drops were added and incubated for 10 min, and then the electrical signal of the sensor was measured again. As shown in Fig. 5A, the electrical signal of the regenerated sensor could reach more than 96% of its initial value, indicating a satisfactory regeneration of the biosensor. The regeneration experiment was performed repeatedly, and the electrical signal remained above 95% and 90% of the initial value after five and ten cycles, respectively. The long-term stability of the sensor was evaluated by using it continually for 12 measurements done over 12 consecutive days (Fig. 5B). It was found that the biosensor could provide a stable signal for 8 d, whereas the response decreased by about 20% from the ninth day. Then, the repeatability of the biosensor in the fabrication process was evaluated. Specifically, five electrodes were prepared at the same time and characterized after each step of the process. As shown in Fig. 5C, the current response of the electrodes had good reproducibility after each of the three steps of the fabrication process of the biosensor.
 |
| Fig. 5 (A) Regeneration of the biosensor by an 8 min wash with 6 M guanidine hydrochloride at room temperature (concentration of bevacizumab: 10 nM). (B) Long-term stability of the biosensor (SPGE/MB/MCH) signal for 12 consecutive days, the biosensor was stored at 4 °C with a drop of PBS on its surface. (C) Batch-to-batch repeatability of the biosensor evaluated by comparing five electrodes after each of the three steps of the fabrication process. | |
3.6. Analytical applications of the Ach biosensor
After the evaluation of the analytical performance of the biosensor, its practical application was further investigated. Healthy human plasma samples were spiked with bevacizumab at three concentrations (0.01, 0.1 and 1 nM). The accuracy and precision of the sensor responses were evaluated using the recoveries and the RSDs, respectively. As shown in Table 2, the recoveries for the spiked plasma samples at the three concentrations were 105.3%, 97.6% and 108.7%, respectively, whereas the corresponding RSD values were 6.9%, 7.2% and 5.8%, respectively. Therefore, these results indicated that the biosensor had good potential for the accurate and precise detection of bevacizumab in plasma samples.
Table 2 Recovery and reproducibility of bevacizumab in spiked human plasma
Samples |
Amount (nmol L−1) |
Dilution factor |
Recoverya (%) |
RSDa (%) |
For each concentration, the average value of three experiments was used to calculate the recoveries and the relative standard deviations.
|
1 |
0.01 |
10 |
105.3 |
6.9 |
2 |
0.1 |
10 |
97.6 |
7.2 |
3 |
1 |
10 |
108.7 |
5.8 |
4. Conclusions
By modifying the original aptamer of bevacizumab, a chimeric hairpin DNA aptamer probe (Ach probe) with a specific recognition ability for bevacizumab was successfully designed. Based on the spatial conformational changes of the probe when interacting with bevacizumab, a one-step electrochemical biosensing platform for the rapid detection of bevacizumab in plasma was constructed. The screened Ach probe could not only exist in the form of stable hairpin structure, but also maintained a high specificity for bevacizumab. By optimizing the concentration of the Ach probe on the electrode, a higher initial current signal was obtained, and the highly sensitive detection of bevacizumab, with a LOD of 0.37 pM, could be realized. Finally, the satisfactory determination of the target antibody in the spiked plasma samples was also achieved. Thus, the chimeric hairpin DNA aptamer-based biosensing platform can be used for the one-step determination of bevacizumab in a broad concentration range (1 pM–1 μM), which has great potential for the future therapeutic drug monitoring of biopharmaceuticals.
Conflicts of interest
The authors declare they have no conflict of interests.
Acknowledgements
This work was supported by the National Natural Science Foundation of China (Grant No. 82273893, 82173773, 82304444), the Natural Science Foundation of Guangdong Province, China (Grant No. 2022A1515011576, 2021A1515220099, 2021A0505030039, 2022A1515110641), and the Anhui Medical University Research Foundation, China (Grant No. 2022xkj123).
References
- N. L. Bragazzi, A. Watad, G. Damiani, M. Adawi, H. Amital and Y. Shoenfeld, Expert Rev. Mol. Diagn., 2019, 19, 969–978 CrossRef CAS.
- L. M. Weiner, R. Surana and S. Z. Wang, Nat. Rev. Immunol., 2010, 10, 317–327 CrossRef CAS PubMed.
- S. J. Casak, M. Donoghue, L. Fashoyin-Aje, X. Jiang, L. Rodriguez, Y.-L. Shen, Y. Xu, X. Jiang, J. Liu and H. Zhao, Clin. Cancer Res., 2021, 27, 1836–1841 CrossRef CAS PubMed.
- P. L. McCormack and S. J. Keam, Drugs, 2008, 68, 487–506 CrossRef CAS PubMed.
- N. Iwamoto, Y. Umino, C. Aoki, N. Yamane, A. Hamada and T. Shimada, Drug Metab. Pharmacokinet., 2016, 31, 46–50 CrossRef CAS.
- G. Nugue, M. Bidart, M. Arlotto, M. Mousseau, F. Berger and L. Pelletier, PLoS One, 2013, 8, e72021 CrossRef CAS PubMed.
- C. Fredolini, S. Byström, E. Pin, F. Edfors, D. Tamburro, M. J. Iglesias, A. Häggmark, M.-G. Hong, M. Uhlen and P. Nilsson, Expert Rev. Proteomics, 2016, 13, 83–98 CrossRef CAS PubMed.
- C. Tuerk and L. Gold, Science, 1990, 249, 505–510 CrossRef CAS PubMed.
- A. D. Ellington and J. W. Szostak, Nature, 1990, 346, 818–822 CrossRef CAS PubMed.
- S. P. Song, L. H. Wang, J. Li, J. L. Zhao and C. H. Fan, TrAC, Trends Anal. Chem., 2008, 27, 108–117 CrossRef CAS.
- K. Chen, J. Zhou, Z. Shao, J. Liu, J. Song, R. Wang, J. Li and W. Tan, J. Am. Chem. Soc., 2020, 142, 12079–12086 CrossRef CAS PubMed.
- J. Li, L. T. Mo, C. H. Lu, T. Fu, H. H. Yang and W. H. Tan, Chem. Soc. Rev., 2016, 45, 1410–1431 RSC.
- H. G. Sun and Y. L. Zu, Molecules, 2015, 20, 11959–11980 CrossRef CAS.
- A. Bonanni and M. Pumera, ACS Nano, 2011, 5, 2356–2361 CrossRef CAS PubMed.
- P. Svoboda and A. Di Cara, Cell. Mol. Life Sci., 2006, 63, 901–918 CrossRef CAS PubMed.
- J. Zheng, R. H. Yang, M. L. Shi, C. C. Wu, X. H. Fang, Y. H. Li, J. H. Li and W. H. Tan, Chem. Soc. Rev., 2015, 44, 3036–3055 RSC.
- P. V. Riccelli, F. Merante, K. T. Leung, S. Bortolin, R. L. Zastawny, R. Janeczko and A. S. Benight, Nucleic Acids Res., 2001, 29, 996–1004 CrossRef CAS PubMed.
- A. Vallee-Belisle, F. Ricci, T. Uzawa, F. Xia and K. W. Plaxco, J. Am. Chem. Soc., 2012, 134, 15197–15200 CrossRef CAS PubMed.
- A. Vallee-Belisle and K. W. Plaxco, Curr. Opin. Struct. Biol., 2010, 20, 518–526 CrossRef CAS PubMed.
- J. C. Cunningham, N. J. Brenes and R. M. Crooks, Anal. Chem., 2014, 86, 6166–6170 CrossRef CAS PubMed.
- C. Y. Deng, X. M. Pi, P. Qian, X. Q. Chen, W. M. Wu and J. Xiang, Anal. Chem., 2017, 89, 966–973 CrossRef CAS PubMed.
- X. Y. Gao, X. L. Wang, Y. C. Li, J. L. He and H. Z. Yu, Anal. Chem., 2018, 90, 8147–8153 CrossRef CAS PubMed.
- J. Jia, H. G. Chen, J. Feng, J. L. Lei, H. Q. Luo and N. B. Li, Anal. Chim. Acta, 2016, 908, 95–101 CrossRef CAS PubMed.
- B. Y. Jiang, F. Z. Li, C. Y. Yang, J. Q. Xie, Y. Xiang and R. Yuan, Anal. Chem., 2015, 87, 3094–3098 CrossRef CAS PubMed.
- P. H. Ling, J. P. Lei, L. Zhang and H. X. Ju, Anal. Chem., 2015, 87, 3957–3963 CrossRef CAS PubMed.
- J. Pang, Z. P. Zhang and H. Z. Jin, Biosens. Bioelectron., 2016, 77, 174–181 CrossRef CAS PubMed.
- Q. Su, D. Wesner, H. Schonherr and G. Noll, Langmuir, 2014, 30, 14360–14367 CrossRef CAS PubMed.
- W. Wei, L. Q. Zhang, Q. W. Ni, Y. P. Pu, L. H. Yin and S. Q. Liu, Anal. Chim. Acta, 2014, 845, 38–44 CAS.
- D. C. Zhang, Y. R. Yan, H. Y. Que, T. T. Yang, X. X. Cheng, S. J. Ding, X. M. Zhang and W. Cheng, ACS Sens., 2020, 5, 557–562 CrossRef CAS PubMed.
- W. Q. Zhang, H. Xu, X. X. Zhao, X. Q. Tang, S. Yang, L. Y. Yu, S. Zhao, K. Chang and M. Chen, Anal. Chim. Acta, 2020, 1122, 39–47 CrossRef CAS PubMed.
- X. M. Meng, M. R. Xu, J. Y. Zhu, H. S. Yin and S. Y. Ai, Electrochim. Acta, 2012, 71, 233–238 CrossRef CAS.
- X. P. He, G. F. Wang, G. Xu, Y. H. Zhu, L. Chen and X. J. Zhang, Langmuir, 2013, 29, 14328–14334 CrossRef CAS PubMed.
- A. Porchetta, R. Ippodrino, B. Marini, A. Caruso, F. Caccuri and F. Ricci, J. Am. Chem. Soc., 2018, 140, 947–953 CrossRef CAS PubMed.
- S. Ranallo, D. Sorrentino and F. Ricci, Nat. Commun., 2019, 10, 5509 CrossRef CAS PubMed.
- T. Yamada, T. Saito, Y. Hill, Y. Shimizu, K. Tsukakoshi, H. Mizuno, H. Hayashi, K. Ikebukuro, T. Toyo'oka and K. Todoroki, Anal. Chem., 2019, 91, 3125–3130 CrossRef CAS PubMed.
- Y. Xiao, R. Y. Lai and K. W. Plaxco, Nat. Protoc., 2007, 2, 2875–2880 CrossRef CAS PubMed.
- H. Li, P. Dauphin-Ducharme, G. Ortega and K. W. Plaxco, J. Am. Chem. Soc., 2017, 139, 11207–11213 CrossRef CAS PubMed.
- R. Tavallaie, J. McCarroll, M. Le Grand, N. Ariotti, W. Schuhmann, E. Bakker, R. D. Tilley, D. B. Hibbert, M. Kavallaris and J. J. Gooding, Nat. Nanotechnol., 2018, 13(11), 1066–1071 CrossRef CAS PubMed.
|
This journal is © The Royal Society of Chemistry 2024 |
Click here to see how this site uses Cookies. View our privacy policy here.