DOI:
10.1039/D3AN01405C
(Paper)
Analyst, 2024,
149, 231-243
NaYF4:Yb/Ho upconversion nanoprobe incorporated gold nanoparticle (AuNP) based FRET immunosensor for the “turn-on” detection of cardiac troponin I†
Received
16th August 2023
, Accepted 10th November 2023
First published on 15th November 2023
Abstract
Cardiac troponin I (cTnI) is a significant biomarker for acute heart attack. Hence, fast, economical, easy and real time monitoring of cardiac troponin I (cTnI) is of great importance in diagnosis and prognosis of heart failure in the healthcare domain. In this work, an immunoassay based on NaYF4:Yb/Ho based photon-upconversion nanoparticle (UCNP) with narrow emission peaks at 540 nm and 655 nm respectively, is synthesized. Then, it is encapsulated with amino functionalized silica using 3-aminopropyltriethoxysilane (APTES) to form APTES@SiO2-NaYF4:Yb/Ho UCNPs. When AuNPs is added to this system, the fluorescence is quenched by the electrostatic interaction with APTES@SiO2-NaYF4:Yb/Ho UCNPs, thereby exhibiting a FRET-based biosensor. When the cTnI antigen is introduced into the developed probe, an antibody–antigen complex is formed on the surface of the UCNPs resulting in fluorescence recovery. The developed sensor shows a linear response towards cTnI in the range from 0.1693 ng mL−1 to 1.9 ng mL−1 with a low limit of detection (LOD) of 5.5 × 10−2 ng mL−1. The probe exhibits adequate selectivity and sensitivity when compared with coexisting cardiac biomarkers, biomolecules and in real human serum samples.
1. Introduction
Acute myocardial infarction (AMI) is a serious health issue that increases the mortality and morbidity rates by a sudden restriction in blood flow to the heart. It is one of the most prevalent forms of cardiovascular illness associated with acute ischemia and hypoxia which is followed by permanent tissue damage in the myocardial cells.1,2 The MI is caused by the damage in heart cells resulting in impaired cardiac function leading to heart failure (HF).3 According to World Health Organisation (WHO) statistics, 17.9 million died due to cardiovascular diseases (CVDs) in 2019, representing 32% of all global mortality. In accordance with the World Heart Federation (WHF) vision statement, the burden of CVD is projected to rise to 22.2 million in 2030.4 Hence, in order to lower the risk of the death rate due to heart attack, it is highly beneficial to develop a prompt and accurate diagnostic system for the easy, economical and early detection of the onset of MI.5 Cardiac biomarkers are used extensively as biological indicators for the effective detection of MI. During the cell damage, biomarker proteins such as creatine kinase, myoglobin, C-reactive protein and cardiac troponins in the cell are released which gradually flow through the blood stream6.Compared to other cardiac biomarkers, cTnI and cTnT appear in the blood stream and reaches a peak for 2–3 days after the onset of a severe MI. According to recent studies, the cTnI concentration threshold between healthy individuals and patients is between 0.5 and 2 ng mL−1. Following the AMI event, within 4–6 hours, the cTnI concentration can increase to 50 ng mL−1, and eventually to a concentration of about 550 ng mL−1.7 Moreover, unlike other biomarkers whose concentration will reduce rapidly, its concentration will continue to be increased for a duration of 6–8 days allowing enough of diagnostic window for medical diagnosis of AMI8,9 Hence, cTnI is considered to be a significant biomarker having a high specificity for acute myocardial injury.10
The electrocardiogram (ECG) is the current standard method for determining whether MI has occurred and this measures the electric signals from the heart, as a result of the pacing abnormalities caused by the heart tissues. This method offers sensitivity and timely recognition of the health status of the heart. However, it has limitations in judging the prognosis of the MI in a patient.11 The conventional method for measuring the cTnI levels are enzyme-linked immunosorbent sandwich immunoassays (ELISA),12 radioimmunoassay,13 optoelectronic devices,14 surface plasmon resonance imaging15etc., which are time-consuming, expensive, and complicated operational protocols which make them unfavorable for use in clinical and point-of-care device fabrications. Despite the current challenges faced by the current sensing modalities, fluorescent-based sensing methods are becoming popular in view of its economic feasibility in developing real time monitoring of biomarkers and are currently greatly appreciated by researchers due to their advantages such as photoluminescence properties, simple synthesis methods, biocompatibility, real-time sensing and low-cost.16,17 There are many fluorescent platforms reported for the sensitive detection of cTnI with low detection limits, such as organic dyes,18 carbon dots,19 quantum dots,20 colorimetric detection by gold nanoparticles (NPs)21etc.
Among these, photon-UCNPs generated from lanthanides are an emerging area of nanoparticle research with their unique optical properties due to the presence of successive electronic transitions in the 4f subshells.22 These NPs can sequentially absorb multiphotons at a longer wavelength (the 980 nm region) and emission at a shorter wavelength region (UV-visible region) with high energy photons. This unique anti-Stokes shift property enables its widespread application in the field of biomedicine by avoiding autofluorescence caused by UV-visible excitation in biological samples23,24 Other key characteristics of the UCNPs are high photostability, deep tissue penetration, chemical stability, large anti-Stokes shift, and a narrow emission peak.25 Sirkka et al.26 and Raiko et al.27 have fabricated NaYF4:Yb,Er based heterogenous UC immunoassays immobilized in streptavidin coated microwells by a biotinylated anti-cTnI monoclonal antibody and a microplate based NaYF4:Yb,Er UCNPs sandwich immunoassay for the detection of the cTnI.26 There are challenges with these immunoassays because of aggregation due to reduction, and non-specific binding interactions with the antibody which necessitates careful optimization conditions for specific detection of cTnI.27 In 2021, Bayoumy et al. developed a NaYF4:Yb,Er UCNP based lateral flow immunoassay (LFIA) for the detection of cTnI in human blood.28 However, the matrix effect, stability, sensitivity and quantitative detection are major limiting factors of LFIA.
The fluorescence resonance energy transfer (FRET)-based photon-UCNPs are potential candidates for use in sensing platforms as energy donors in combination with two dimensional nanomaterials,29 metal NPs),30 organic dyes and quantum dots31 as energy acceptors in biosensing applications32,33 Among these, the gold nanoparticles (AuNPs) have comparable absorption at the UCNPs emission region. However, the FRET-based immunoassay for detection of cardiac biomarkers is seldom explored. The UCNPs mostly consist of a host matrix, sensitizer, and an activator, while Yb3+ is used as sensitizer due to its large absorption cross section.34 The lanthanide dopants such as Er3+, Tm3+ and Ho3+ are often selected as activators due to their matching ladder like energy levels.34 Most of the reported work focused on energy transfer between 2F7/2 to 2F5/2 (Yb3+/Er3+). Among the activators, holmium has well matched energy levels 2F5/2 to 5I6 (Yb3+/Ho3+), long lifetimes, possesses a unique spectral emission at 544 nm and 648 nm, and its paramagnetic behaviour has been less explored in developing immunoassays. For this reason, we have chosen Holmium based UCNPs for developing an immunosensor for the specific detection of cTnI.35–37
In this perspective, we have synthesized a Holmium based NaYF4:Yb/Ho photon-upconversion nanoparticle (UCNP) using a microwave assisted method. The surface modification of the prepared UCNPs results in the formation of amino functionalized photon-UCNPs (APTES@SiO2-NaYF4:Yb/Ho).38 For cTnI detection, a FRET-based biosensor was fabricated by combining the properties of the antibody conjugated UCNPs and AuNPs. On introduction of cTnI, an antigen–antibody complex is formed which disrupts the FRET-based system leading to fluorescence enhancement. The probe exhibits more specificity to cTnI in presence of other interfering biomolecules and ions. The practical application of the developed probe was validated by the detection of cTnI in a human serum sample. A schematic illustration of the proposed work is given in Scheme 1.
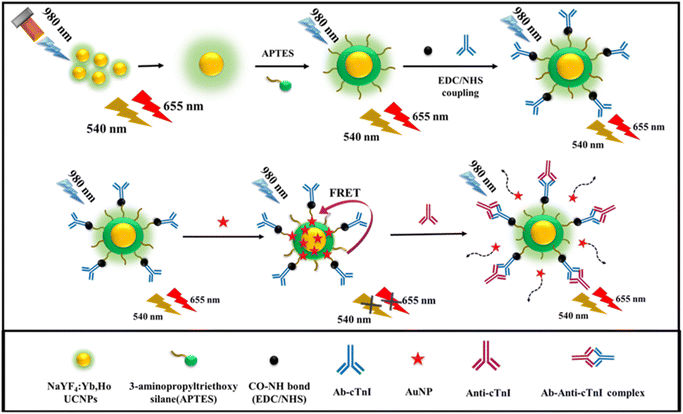 |
| Scheme 1 The schematic illustration of the detection of cTnI, based on developed upconversion nanoprobe. | |
2. Experimental section
2.1. Materials
Ytterbium(III) nitrate pentahydrate (YbNO3·5H2O), yttrium(III) nitrate hexahydrate (YNO3·6H2O), phosphate buffered saline (PBS), and the cTnT were purchased from Sigma-Aldrich. Holmium(III) chloride hexahydrate (HoCl3·6H2O) was obtained from Alfa Aesar. The 1-ethyl-3-(3-dimethylaminopropyl)carbodiimide (EDC) and N-hydroxysuccinimide (Sulfo-NHS) were purchased from Spectrochem, Mumbai, India. Cardiac troponin I (CTnI), antibody-CTnI (ab-CTnI) and Human B-Type natriuretic peptide (proBNP) were purchased from Abcam, India. The 3-aminopropyltrimethoxysilane (APTES) and tetraethoxysilane (TEOS) were obtained from Tokyo Chemical Industry (TCI) Chemicals, India. Ammonia, chloroauric acid (HAuCl4·3H2O), creatinine, glucose, potassium chloride, sodium chloride (NaCl), sodium hydroxide, trisodium citrate, and so on were purchased from Nice Chemicals, Kochi, Kerala, India. All the experiments were carried out using ultrapure distilled water.
2.2. Instrumentation
The upconversion luminescence spectra were recorded using 2 W laser power excitation source in a fluorescence lifetime spectrofluorometer (FLS1000, Edinburgh Instruments, UK). The UV visible absorption spectra were measured using a fluorescence and absorption spectrometer (Duetta, Horiba Scientific). The ATR-FTIR analysis was carried out using an FTIR spectrometer (Cary 630, Agilent). The dynamic light scattering (DLS) and zeta potential analysis were studied using a nanoparticle analyzer (SZ-100, Horiba Scientific). The samples were synthesized using a domestic microwave oven. The lifetime analysis was carried out using a microsecond flash lamp (Edinburg Instruments) with an arbitrary function generator (AFG31000 Series, Tektronix). The scanning electron microscopy (SEM) analysis was also performed (EVO 18, Carl Zeiss). The high-resolution X-ray photoelectron spectrometry (XPS) was also carried out (ESCALAB Xi+, ThermoScientific).
2.3. Synthesis of the NaYF4:Yb/Ho photon-upconversion nanoparticle
The synthesis of the NaYF4:Yb/Ho was carried out using a new microwave assisted synthesis, with minor modification, found in a previous report.39 Here, the rare earth nitrates and chlorides were used as precursors. Initially, 0.52 g of YNO3·6H2O, 0.169 g of YbNO3·6H2O and 0.018 g of HoCl3·6H2O were mixed with 15 mL of ethylene glycol and 15 mL of distilled water, and then magnetically stirred for 5 min. To this mixture, 0.3704 g of ammonium fluoride (NH4F) and 0.117 g of NaCl were added and stirred well until a homogeneous solution was obtained. The mixture was then placed under microwave irradiation for 10–15 min at 350 W. The process was continued until a white precipitate of NPs was obtained. Next, the precipitate was centrifuged, washed with ethanol and water, and then filtered. The precipitate obtained was then dried in a hot air oven at 50 °C for 30–40 min. The fine white powder of the synthesized NaYF4:Yb/Ho photon-UCNPs obtained was stored in a refrigerator at 4 °C until required.
2.4. Surface modification of the UCNPs (APTES@SiO2-NaYF4:Yb/Ho)
The powdered photon-UCNPs (40 mg) were dissolved in 3 mL of PBS buffer. To this mixture, 5 mL of isopropyl alcohol (IPA) was added and then the solutions was ultrasonicated for 30–35 min until a colloidal solution was obtained. To this a mixture of 10 mL of distilled water and 1 mL of NH3 were added, and then magnetically stirred for 10 min at 35 °C. To this mixture, 5 μL of TEOS and 20 μL of APTES were added dropwise, and the reaction was continued for 3 h.40 The solution obtained was centrifuged, filtered, washed and then dried in an oven. The white powder obtained was the amino functionalized photon-UCNPs. It was then collected and stored at 4 °C in a refrigerator.
2.5. Synthesis of gold nanoparticle (AuNPs)
Synthesis of the AuNPs was carried out using a citrate reduction method.41 The HAuCl4·3H2O solution and trisodium citrate solution were used in a 5
:
1 ratio, and 100 mL of 0.05 mM HAuCl4·3H2O was mixed with 20 mL of 10 mM trisodium citrate, and the pH is adjusted to 5. This mixture was stirred magnetically at 100 °C until the color of the solution changed from purple to wine red. The gold NPs obtained were stored at 4 °C until required for further analysis.
2.6. Conjugation of antibody-AuNPs-UCNPs immunoassay
The cTnI antibody (Ab-cTnI) was conjugated onto the surface of the photon-UCNP by an EDC-sulfo-NHS mediated coupling reaction. Typically, about 30 μL of a mixture of 0.4 mg of EDC and 1.1 mg of NHS in PBS buffer, was dissolved in 2970 μL of Ab-cTnI (1 μg mL−1) for 15 min. Next, 3 mL of amino-functionalized UCNPs in PBS buffer was added to this mixture, where the ratio between Ab-cTnI and the UCNPs was maintained at a 1
:
1 ratio. It was then incubated, with continuous magnetic stirring, for about 1 hour under cold conditions at a temperature of about 20 °C. Next, the Ab-cTnI conjugated UCNPs was stored at −2 °C until required for further studies.
2.7. Detection of cTnI
About 3 mL of the prepared Ab-cTnI coupled upconversion nanoprobe (Ab-cTnI-APTES@SiO2-NaYF4:Yb,Ho) was placed in a quartz cuvette. Then, 0.05 mM of synthesized AuNPs were added incrementally up to 30 μL to form the Ab-cTnI-AuNP-APTES@SiO2:NaYF4:Yb/Ho upconversion nanoprobe. To this fluorescence quenched system, 5 μg mL−1 of different concentrations of the cTnI antigen (0–1.9 ng mL−1) were added in incremental volumes of 10 μL, 20 μL, 30 μL, and so on, and the upconversion spectra were recorded. All the measurements were done in triplicate to ensure the repeatability.
2.8. Real sample analysis
The real sample analysis was conducted in human blood serum samples extracted from healthy volunteers and spiked with the cTnI antigen at one of three different concentrations such as concentrated serum, 10 times and 100 times diluted serum. All the experiments were performed in compliance with The Indian Council of Medical Research (ICMR) recommendations, and approved by the Human Ethical Committee at the University of Kerala (Order no: ULECRIHS/UOK/2019/48, University of Kerala, Thiruvananthapuram). Informed consent was obtained from all the human subjects who volunteered for the study involving real human blood serum samples.
3. Results and discussion
3.1. Synthesis and characterization of the photon-upconversion nanoparticle (NaYF4:Yb/Ho)
To potentially enhance the emission profiles, the selection of suitable sensitizers and activators with well-matched energy levels is vital for the signaling and to improve the upconversion luminescence (UCL) intensity. Traditional UCNPs have a low quantum yield due to limitations such as surface deactivation defects, thus many techniques have been employed to refine the host materials, such as developing an active core–shell structure, implementing plasmonic architecture on the surface of the UCNPs and so on.42 The conventional methods of preparation of the UCNPs are thermal decomposition, coprecipitation, hydrothermal, sol gel synthesis which requires optimized preparation protocols.43
In this work, the NaYF4:Yb/Ho photon-UCNPs were synthesized by a microwave assisted method at 350 W. Microwave irradiation methods are less time consuming, and the products have good reproducibility and minimized side reactions.44 During synthesis, the rare earth nitrates was mixed with ethylene glycol solvent. As the reaction proceeded, the rare earth ions were released from the precursors. Furthermore, on addition of NH4F and NaCl, the rare earth ions reacted with the Na and F ions and underwent a particle size controlled growth to form NPs. A fine white powdered sample was obtained after dehydration in an oven. Here, Yb3+ was selected as the sensitizer as it has a sharp absorption cross section of about 10−20 cm2 at the 980 nm region, and Ho3+ was chosen as the activator ion.45 The energy level between the 2F5/2 of Yb3+ as the sensitizer, matched well with the 5I6 energy level of Ho3+. Moreover, the Ho3+ was capable of being sensitized by Yb3+ followed by an energy transfer of 5F4 to 5S2 by a two-photon phenomenon leading to UCL emission with a green emission at 540 nm caused by it receiving energy from Yb3+ as shown in Fig. 1A. Simultaneously, the excited state 5I6 of Ho3+ which was sensitized by Yb3+ can also relax to the ground state 5I7 and was further populated to 5F5 accompanied by a red emission at 655 nm due to the electrons returning back to the ground state 5I8.46 The chromaticity plot (CIE plot: x = 0.3785, y = 0.5571) corresponding to green emission is shown in Fig. 1B. The synthesized nanoparticle was subjected to UCL with 980 nm as the excitation source at different powers ranging from 0.65 W cm−2 to 0.90 W cm−2 as shown in Fig. 1C.
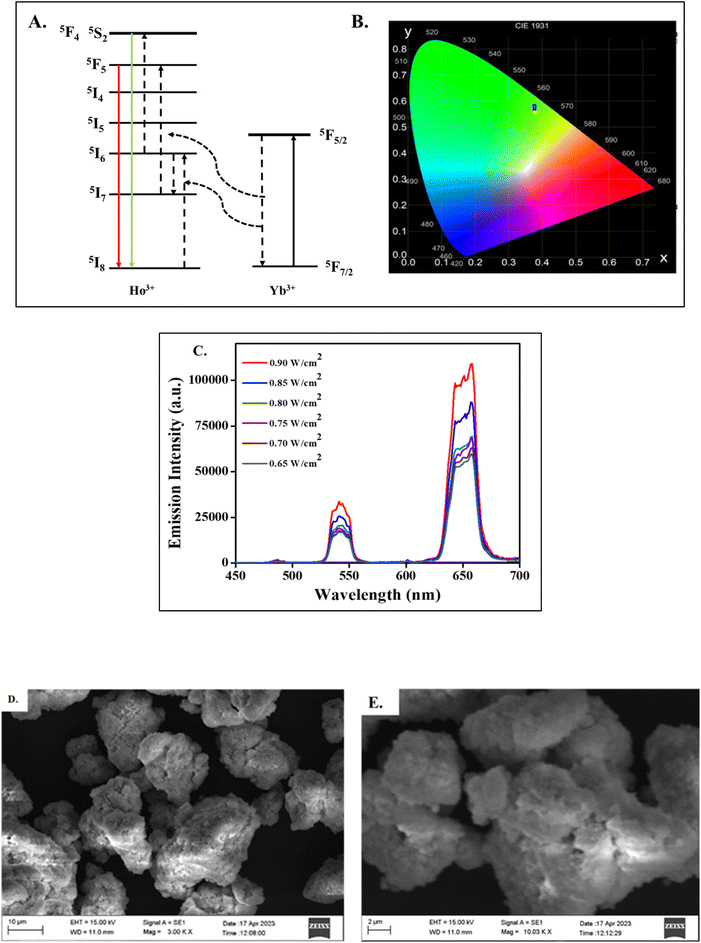 |
| Fig. 1 (A) Illustration of the energy transfer mechanism from Yb3+ to Ho3+ in up conversion luminescence. (B) The chromaticity plot of the prepared NaYF4:Yb/Ho UCNPs. (C) The emission spectrum of NaYF4:Yb/Ho at different power intensities (0.65 W cm−2–0.90 W cm−2) (D) The SEM images of the synthesized NaYF4:Yb/Ho UCNPs powder at a magnification of 3.00 K X, and (E) at a magnification of 10.03 K X. | |
A good colloidal stability of the NPs is a prerequisite criterion for most of the applications in biological domains. Indeed, the synthesized nanoparticle showed good dispersal behaviour in dimethyl formamide (DMF), dimethyl sulfoxide (DMSO), and PBS buffer. In which the signal counts and the stability of the material were most noticeable in PBS (pH∼7.4) and for this reason, the entire study was conducted in PBS medium. Moreover, the PBS was considered as an appropriate medium for antibody conjugation without causing denaturation of the antibody and thereby facilitating the effective binding of the Ab-cTnI to the UCNPs.47 The solvent dependent emission peaks are shown in Fig. S1 (ESI†). In order to evaluate the size distribution and colloidal stability of synthesized NaYF4:Yb/Ho photon-UCNPs, a DLS study was conducted. The nanoparticle exhibited a hydrodynamic size of ∼41.1 nm which revealed the particle size of the synthesized NPs, as shown in Fig. S2 (ESI†). The morphological surface of the UCNPs was studied using SEM analysis as shown in Fig. 1D and E. The SEM image depicts the uniform cauliflower like crystalline structure of the prepared upconversion material. This indicates that the Holmium doped UCNPs prepared by microwave synthesis exhibit a regular crystalline shape with luminescence properties.48
The high resolution X-ray photoelectron spectroscopy (XPS) was used to evaluate the elemental composition and oxidation states of the elements present on the surface of the NaYF4:Yb/Ho UCNPs. From the survey spectrum, Fig. S3h (ESI†), it was evident that the UPNPs contained C, F, Ho, Na, O, Y, and Yb as elements that corresponded to particular satellite peaks. Fig. S3a (ESI†) corresponds to O 1s which displays two characteristic binding energy peaks at 532.12 eV and 537.2 eV. The two peaks of O 1s were used to identify the O–H on the surface of nanoprobe. The C 1s spectra corresponded to oxygenated C
C and C–H on the surface and give a single peak at 283.91 eV as shown in Fig. S3b (ESI†). The F1s has a sharp satellite peak in the survey spectrum and displayed a single peak at a binding energy of 684.14 eV as shown in Fig. S3c (ESI†), which shows that the core shell of the nanoprobe was composed of fluoride. The peak corresponding to the Na 1s spectra has a single peak at 1071.33 eV as shown in Fig. S3d (ESI†) representing the Na+ ions in the coordination sites of the nanoprobe surface. The doping concentration of the elements can be attributed from the results of the XPS analysis. From Fig. S3e (ESI†), it was evident that Ho was doped on the surface of nanoprobe with an atomic composition of 5.24% which corresponds to two characteristic peaks at 157.8 eV and 160.08 eV. These results suggest that successful doping of the Ho3+ element on the surface of UC nanoprobe has occurred.49 In Fig. S3f (ESI†), the Y 3d has two characteristics peaks at 158.74 eV and 162 eV which correspond to elemental Y3+ at the lattice points. The Yb 4d has a single peak with a low binding energy of 185.03 eV with a low intensity attributed to the 0.28% atomic composition on the surface of nanoprobe as shown in Fig. S3g (ESI†). The XPS survey spectrum show the presence of the satellite peaks of the corresponding elements with atomic compositions of O 1s (12.48%), C 1s (10.49%), F 1s (55.6%), Na 1s (7.34%), Ho 4d (0.35%), Y 3d (13.46%) and Yb 4d (0.28%) respectively.
3.2. Development of the amino-functionalized NaYF4:Yb/Ho nanoprobe
The surface modification of photon-UCNP is a key step in optimizing the properties for biomedical applications, disease therapy and the development of immunoassays. It can be done by using various methods including ligand exchange, functionalization, bioconjugation, and so on according to the particular application.34 The as-synthesized NaYF4:Yb/Ho was surface functionalized using the Stöber method. Typically, the white precipitate of the synthesized NaYF4:Yb/Ho NPs were initially coated with a layer of SiO2 using TEOS, followed by amination of the amino-terminated silane coupling agent, APTES, by a basic hydrolysis reaction. The APTES contains amino functionalized -NH2 groups that can bind the Ab-cTnI with the upconversion nanoprobe (NaYF4:Yb,Ho). The amination process offers better stability and provides cytotoxicity to the surface of the UCNPs. The synthesized NPs were characterized by FTIR spectroscopy and the surface charge was determined by zeta potential analysis.
Fig. 2A shows the FTIR spectra of the amino-functionalized photon-UCNP. The synthesized NaYF4:Yb/Ho gives a corresponding peak at 1419 cm−1 which shows the C–H bending, and a peak at 3282.1 cm−1 which is attributed to the O–H stretching vibrations which were gradually broadened after the surface modification. It was evident that after the amination reaction, there was an appearance of a sharp peak at 1032 cm−1 that corresponded to the Si–O stretching vibration due to the formation of a silica coating on the surface of the synthesized NaYF4:Yb/Ho NPs. The peaks at 1635 cm−1 and 3230 cm−1 correspond to the N–H bending, and the stretching vibration of the NH2 groups on the surface.50 The surface charge of the APTES@SiO2 was determined by zeta potential analysis, and it was found to be +2.7 mV as shown in Fig. S4 (ESI†). This positive charge shows the presence of positively charge NH2 groups on the surface of synthesized UCNP. The emission spectra of the APTES@SiO2-NaYF4:Yb/Ho NPs were obtained by excitation using a 980 nm NIR laser at 0.78 W cm−2. Two peaks corresponding to 540 nm and 655 nm were obtained and are shown in Fig. 2B. The selection of the excitation power was optimized in accordance with the emission intensity of the synthesized sample (NaYF4:Yb/Ho) and the maximum permissible exposure for the commonly utilized 980 nm CW laser irradiation was 0.73 W cm−2.51
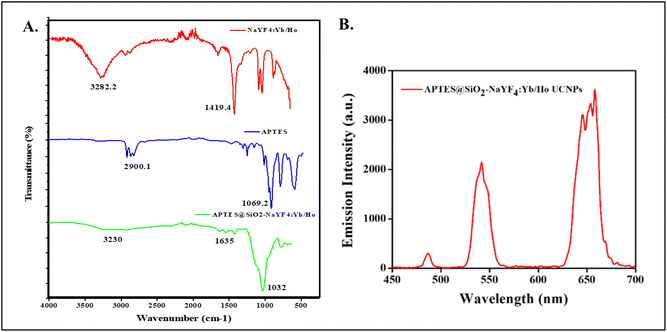 |
| Fig. 2 (A) The FTIR spectra of NaYF4:Yb/Ho photon-upconversion nanoparticle (red) and the amino functionalized APTES@SiO2-NaYF4:Yb/Ho nanophosphor. (B). The emission spectra of APTES@SiO2−NaYF4:Yb/Ho upconversion nanoparticle (λex = 980 nm, λem = 540 nm and 655 nm). | |
3.3. Development of the UCNPs probe for sensing cTnI
Metal NPs were used to fabricate the nanomaterials due to its high potential properties including plasmonic enhancements, and metallic and optical properties. Because, the metal nanoparticle exhibits a plasmon absorption peak in the range of 400–600 nm which is far away from the excitation wavelength of the UCNPs, the possibility of exploring this plasmonic absorption peak of the AuNPs as absorber is significant. In this work, the amino functionalized photon-UCNP (APTES@SiO2-NaYF4:Yb/Ho) which has emission peaks at 540 nm (which matches with the plasmonic absorption peak of the AuNPs) and 655 nm which act as a donor with the AuNP which acts as an acceptor with an absorption peak at about 520–540 nm.
The ultra-small sized AuNPs was synthesized by a citrate reduction method.52 In the reduction process, 0.05 mM of HAuCl4 was used as a precursor when, the Au3+ is converted to Au0 in presence of a reducing agent (trisodium citrate) with a specific ratio (5
:
1) at a pH of 5. The color of the AuNPs is a crucial size determining factor. When, on interaction with light, the collective oscillation of the electron clouds around the nanoparticle are confined, it results in a sharp plasmonic absorption peak around the green region at 540 nm as shown in Fig. 3A. Subsequently, the AuNPs appear to have a complementary wine-red color under visible light.53 The hydrodynamic size of synthesized AuNP was determined using DLS and was found to be ∼52.8 nm which revealed the formation of small sized NPs as shown in Fig. S5 (ESI†). The zeta potential value of the AuNP was −1.7 mV as shown in Fig. S6 (ESI†), which was attributed to negatively charged citrate group on the surface. The morphological characterization of the synthesized AuNPs was done using SEM analysis, and it was observed that the synthesized AuNPs exhibited an aggregated spherical shaped nanocrystals as shown in Fig. 3C.54
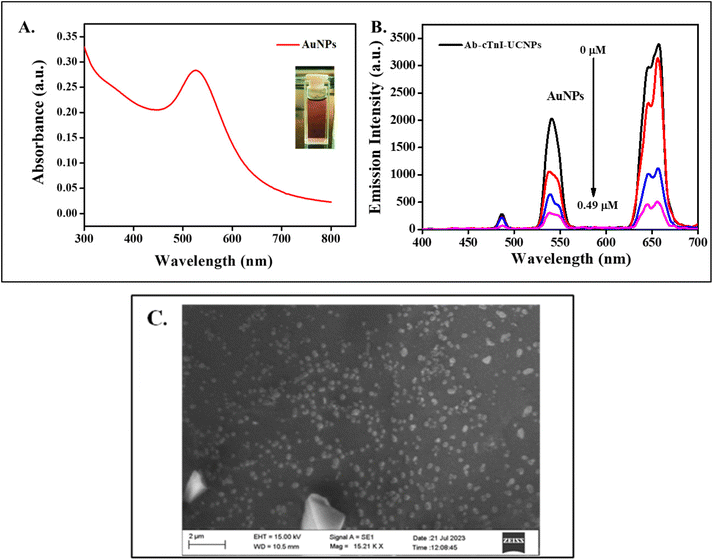 |
| Fig. 3 (A) The UV-visible absorption spectra of synthesized gold nanoparticle at 540 nm, and digital photograph of the synthesized wine-red colored gold nanoparticles with a particle size of ∼52.3 nm (inset). (B) The emission spectra of Ab-cTnI conjugated APTES@SiO2-NaYF4:Yb/Ho on addition on 0.49 μM of AuNPs. (C) The SEM image of the synthesized AuNP. | |
The synthesized APTES@SiO2-NaYF4:Yb/Ho was used as a probe for the conjugation of cTnI antibody (Ab-cTnI). Typically, 2970 μL of Ab-cTnI (1 μg mL−1) was conjugated onto the surface of the UCNPs, where the amine group binds with Ab-cTnI during the EDC/NHS coupling reaction.55 The EDC/NHS are carbodiimides such as the EDC typically present when the antibody conjugation process is taking place. Simultaneously, the NHS acts as an additive to EDC and is employed in the crosslinking reaction on the antibody surface loading on the probe.56 The emission spectra of the APTES@SiO2-NaYF4:Yb/Ho NPs and Ab-cTnI conjugated APTES@SiO2-NaYF4:Yb/Ho was obtained and is shown in Fig. S7 (ESI†). The DLS of the antibody conjugated amino-functionalized UCNPs was measured and the result obtained was ∼238.6 nm as shown in Fig. S9 (ESI†). The size of the UCNPs was increased from 41.1 nm to 238.6 nm which was attributed to the successful conjugation of the Ab-cTnI on the surface of the UCNPs. The zeta potential analysis of the Ab-cTnI conjugated amino-functionalized UCNPs was also carried out, and the result obtained was +2 mV as shown in Fig. S10 (ESI†). This positive charge enhanced the possibility of FRET manifestation with the negatively charged AuNPs.
The synthesized AuNPs were added at incremental volumes of 10 μL, 20 μL, and so on to the Ab-cTnI conjugated amino-functionalized photon-UCNPs system (Ab-cTnI-APTES@SiO2-NaYF4:Yb/Ho). This positively charged antibody conjugated UCNP nanoprobe interacted with the negatively charged citrate capped AuNPs via electrostatic interactions.57 Moreover, the characteristic surface plasmon resonance absorption peak of AuNP at about 520–600 nm overlaps with the emission peak of the photon-UCNP at 540 nm facilitating a FRET mechanism as shown in Fig. S8 (ESI†). Due to the specific FRET mechanism between the UCNPs and the AuNPs, the energy transfer upconversion (ETU) and co-operative sensitization upconversion (CSU) between the 2F5/2 of Yb3+ to the 5I6 of Ho3+ in the energy levels of the photon-UCNP was restricted thereby inhibiting the photon avalanche process resulting in the “turn-off” response of the UCL signals.58 The emission spectra of the UCNPs with an increasing concentration of AuNPs (0.49 μM) is shown in Fig. 3B. The resonance energy transfer of the AuNP at 540 nm (5S2 to 5I8) had a lingering effect on the lower lying energy levels which may depopulate the sensitizer. Similar properties are exhibited by the up conversion nanoprobe with two emission peaks.59 Moreover, the minor overlap of the red tail of the AuNP absorption band (540–700nm) with that of the 648 nm (5F5 to 5I8) emission peak of the up conversion nanoprobe may also lead to a secondary inner filter effect (sIFE) between the AuNPs and the up conversion nanoprobe emission peaks.59
Fig. 4 shows the Stern–Volmer plot showing the effect of the addition of different concentrations of AuNPs on the emission intensity of the APTES@SiO2-NaYF4:Yb/Ho nanoprobe up to a concentration of 0.07 mM. The equation of the Stern Volmer plot is I/I0 = 1 + Ksv [Q]. The graph shows a linear response with R2 = 0.9846 and an intercept of 0.885. The concentration of the AuNPs of 0.03 mM (30 μL) was selected as the optimum quenched system for further sensing of cTnI antigen. This concentration was fixed for the cTnI probe because beyond further addition of AuNPs irreversibly quenched the UCNPs system.
 |
| Fig. 4 The linear response of the luminescence intensity (I/I0) of the APTES@SiO2-NaYF4:Yb/Ho photon-upconversion nanoparticles against the increasing concentration of AuNPs. | |
3.4. Plausible mechanism of detection of cTnI
The emission response of the Ab-cTnI conjugated APTES@SiO2-NaYF4:Yb/Ho upconversion nanoprobe was evaluated against increasing concentrations of the cTnI antigen. It was observed that the fluorescence was “turned-on” on addition of the cTnI antigen in a concentration range of 0 ng mL−1 to 1.9 ng mL−1. The electrostatic interaction between the antibody conjugated UCNPs (donor) with the AuNPs (acceptor) brought them into close proximity thus facilitating the FRET mechanism. The fluorescence resonance energy transfer was a dynamic process that happens because of dipole–dipole coupling where the energy transfer occurred non-radiatively from the excited state of the donor fluorophore to the ground state of an acceptor. The AuNP-based energy transfer system exhibited both FRET and nano surface energy transfer (NSET) and a minor contribution from the secondary inner filter effect (sIFE).60 The NSET is a non-radiative point dipole and a surface dipole interaction. The NSET efficiency is based on Fermi's golden rule which states that the rate of energy transfer is inversely proportional to the fourth power of distance between the donor–acceptor pair.61
When the cTnI antigen was introduced onto the nanoprobe, the Ab-cTnI penetrates onto the surface of the UCNPs and binds to Ab-cTnI to form an antibody–antigen complex. This in turn increases the separation and the distance between the AuNP (quencher) and Ab-cTnI conjugated APTES@SiO2-NaYF4:Yb/Ho upconversion nanoprobe associate beyond the FRET manifestation distance. According to the Foster theory, the rate of energy transfer has an inverse sixth power relationship between the distance separating the two interacting molecules (donor and acceptor).62 Because, the antigen–antibody complex is strongly specific, the distance between the AuNP and the Ab-cTnI conjugated APTES@SiO2-NaYF4:Yb/Ho upconversion nanoprobe was disturbed. Consequently, the FRET process was restricted as the quencher AuNP departs from the surface of UCNPs resulting in fluorescence recovery. The increase in upconversion emission was observed at 540 nm and 655 nm upon NIR irradiation due to the energy transfer mechanisms. The emission spectra of the UCNP on addition of the cTnI antigen (0–1.9 ng mL−1) is shown in Fig. 5A. The emission “turn-off” spectra in the absence of cTnI is shown in the green curve, and later on, after addition of the cTnI antigen the fluorescence enhancement was observed (see the red curve). The reason behind the fluorescence recovery was that upon introduction of the cTnI antigen, it split the AuNP-Ab-cTnI conjugated upconversion nanoprobe (AuNP-Ab-cTnI@APTES@SiO2:NaYF4:Yb/Ho) by inhibiting the FRET process.57 All the spectral measurements were recorded in triplicate for each addition of the cTnI antigen (0–1.9 ng mL−1) to the AuNP-APTES@SiO2-NaYF4:Yb/Ho UC nanoprobe to ensure the repeatability of the experiment. In order to ensure reproducibility of the AuNP-APTES@SiO2-NaYF4:Yb/Ho nanoprobe for the detection of the cTnI antigen (1.9 ng mL−1), the turn-on response of the cTnI antigen at different days with the probe was noted and the results confirmed there was good turn-on behavior as shown in Fig. S11A (ESI†). The results confirmed the feasibility of using the developed probe for sensitive detection of the cTnI antigen. The time dependent studies with incubation times of 5 min to 60 min were also evaluated. The response of the AuNP-APTES@SiO2-NaYF4:Yb/Ho probe towards the anti-cTnI increased and after a certain time, it remained constant. The time dependent plot is shown in Fig. S11B (ESI†).
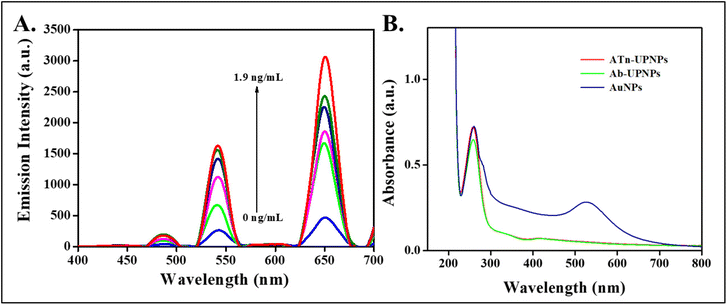 |
| Fig. 5 (A) The upconversion emission turn-on response spectra with the addition of increasing concentrations of the cTnI antigen on the AuNPs-APTES@SiO2-NaYF4:Yb/Ho nanoparticles. (B) The UV-visible absorption spectra of the Ab-cTnI conjugated NaYF4:Yb/Ho nanoprobe (green), cTnI antigen-Ab-cTnI conjugated NaYF4:Yb/Ho nanoprobe (red), gold nanoparticle (blue). | |
The UV-visible absorption spectra of the antibody conjugated APTES@SiO2:NaYF4:Yb/Ho nanoparticle was measured and a strong absorption peak was observed at 290 nm due to the absorption of the antibody-conjugated NPs and the presence of an absorption peak at 530 nm was attributed to the surface plasmon resonance peak of the synthesized AuNPs as shown in Fig. 5B. On addition of the cTnI antigen to this system, there was no peak shift at 290 nm, but there was an increase in the absorbance of the Ab-cTnI conjugation on the UCNPs resulting in fluorescence recovery. A DLS study of the cTnI antigen on the developed nanoprobe was carried out and the hydrodynamic size increased from 238.6 nm to 361.5 nm due to the antigen–antibody cTnI complex formation on the surface as shown in Fig. S12 (ESI†).63
The fluorescence lifetime decay profile of the synthesized NaYF4:Yb,Ho photon-UCNP was determined using a microsecond flash lamp as shown in Fig. 6A. It was found that the average lifetime (Æ®avg) was 164.2 μs and on addition of the antibody conjugated AuNP quenched the NaYF4:Yb,Ho which exhibited a decrease in the average lifetime to 102.7 μs. Furthermore, on addition of the anti-cTnI to the system, the fluorescence lifetime increased from 102.7 μs to 132.75 μs with a biexponential fit. Considering the possibility of NSET mechanism, the distance of AuNP and up conversion nanoprobe was not restricted much. The rate of energy transfer was inversely proportional to the fourth power of the distance between’ the donor–acceptor (photon UCNP pair in the NSET). The formation of an immunocomplex on the surface resulted in a linear turn-on response. The change in lifetime was attributed to the FRET mechanism existing between the donor and acceptor moieties.64–66
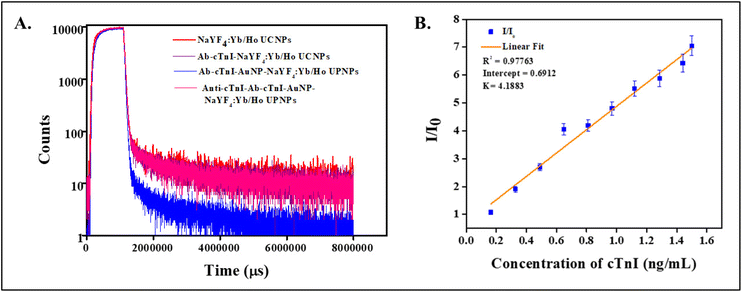 |
| Fig. 6 (A) The fluorescence decay lifetime profile of NaYF4:Yb/Ho photon-upconversion nanoparticle (red), Ab-conjugated NaYF4:Yb/Ho photon-upconversion nanoparticle (violet), the Ab-cTnI-AuNP-conjugated NaYF4:Yb/Ho photon-upconversion nanoparticle (blue) and the anti-cTnI-Ab-cTnI-AuNP conjugated NaYF4:Yb/Ho photon-upconversion nanoparticle (pink). (B) The linear fit plot of the intensity ratio (I/I0) against the concentration of the cTnI antigen (0.164–1.9 ng mL−1). | |
Fig. 6B shows the Stern–Volmer plot for determining the effect of the UCL intensity with the increasing concentration of the cTnI antigen. The equation for the Stern Volmer plot is: I/I0 = 1 + Ksv [Q]. The graph shows a linear response with increasing concentrations of cTnI ranging from 0.163 ng mL−1 to 1.9 ng mL−1. The graph shows a linear response with an R2 of 0.97763 with the intercept at 0. 6912. From this data, the LOD was calculated by using the equation: 3σ/K, where σ is the standard deviation and K is the slope obtained from Stern–Volmer plot. The LOD obtained was 5.5 × 10−2 ng mL−1. Table S2 (ESI†) compares the LOD of the proposed probe with those found in previous reports. The significantly low LOD value validated the sensitive detection of the cTnI.
3.5. Selectivity and interference
The specificity and sensitivity of an immunoassay is the key factor in determining the interaction of the antibody–antigen binding studies. Hence, to evaluate the selectivity of the APTES@SiO2-NaYF4:Yb/Ho up conversion nanoprobe, two common cardiac biomarkers such as cardiac troponin T (0.0319 ng ml−1) and proBNP (319 pg ml−1) were evaluated in addition to biomolecules such as creatinine (0.319 mM), glucose (31.9 mM), and ions such as Na+ and K+ (31.9 mM) were also selected. The concentrations of biomarkers and biomolecules were kept at the concentrations that appear in human body fluids. As shown in Fig. 7A, the results reveal that the developed probe is specific to the cardiac troponin I antigen (cTnI). An interference study of the developed probe was also carried out. Fig. 7B shows the interference bar diagram where, the upconversion luminescence turn-on response towards the cTnI antigen (1.9 ng mL−1), in the presence of other coexisting biomarkers and ions, was evaluated. Interestingly, it was found that on addition of the cTnI antigen (1.9 ng mL−1), the probe showed a good turn-on response towards cTnI over other interfering biomolecules and ions which validates the sensitivity and practical applicability of the probe.
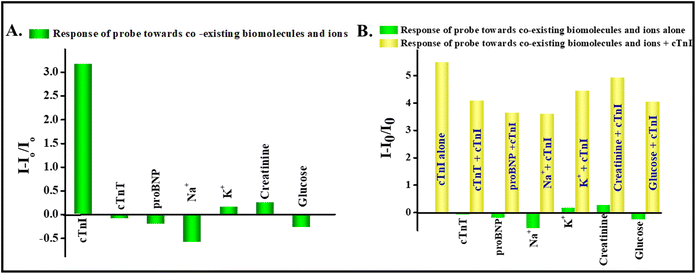 |
| Fig. 7 (A) The selectivity of the APTES@SiO2-NaYF4:Yb/Ho-AuNP system towards cardiac antigens, biomolecules and ions (green bar diagram). (B) Interference study of the APTES@SiO2-NaYF4:Yb/Ho-AuNP system towards cTnI in the presence of other coexisting antigens, biomolecules and ions at the same concentrations (yellow bar diagram) and the selective response depicted in green bar diagram. | |
3.6. Real sample analysis
To evaluate the practical applicability and clinical significance, the developed APTES@SiO2-NaYF4:Yb/Ho-AuNPs upconversion nanoprobe was subjected to real biological fluid samples such as human serum. The study was conducted in human serum extracted from blood samples collected from healthy volunteers. Three different concentrations were prepared such as concentrated serum, 10 times diluted serum and 100 times diluted serum. The probe showed a good recovery percentage in the range from 97%–111% which validated the sensing application of the cTnI in biological samples. Table S1 (ESI†) shows the recovery percentage obtained from different concentrations of cTnI in spiked human serum samples.
4. Conclusions
In summary, a holmium-based photon-upconversion nanoparticle conjugated with cTnI antibody (Ab-cTnI) was synthesized using microwave assisted method. The assay works on the principle of FRET between UCNPS and AuNPs by means of an electrostatic interaction by surface functionalization and donor–acceptor spectral overlap. The UCL emission was suppressed on addition of 0.03 mM AuNPs by the formation of an antibody UCNP quencher complex. However, in the presence of the cTnI antigen, the fluorescence is recovered due to the strong antigen–antibody binding interaction thereby inhibiting the FRET mechanism. The probe shows a turn-on linear response in the range 0–1.9 ng mL−1. A limit of detection of 5.5 × 10−2 ng mL−1 was obtained. The probe extended its application to a real biological matrix, human serum with good recovery percentage in the range of 97%–111% with adequate selectivity and sensitivity with coexisting biomolecules. Thus, the upconversion nanoprobe developed paves the way for the development of a potential nanoprobe for the detection of cTnI in clinics.
Ethical approval
All the experiments were performed in accordance with the Indian Council of Medical Research (ICMR) ethical standards. All the experiments followed the ICMR guidelines and were approved by the University Level Human Ethical Committee, University of Kerala, Thiruvananthapuram. All the experiments were approved by the University Level Human Ethical Committee Order no: ULECRIHS/UOK/2019/48, University of Kerala, Thiruvananthapuram. Informed consent was obtained from all the human subjects who volunteered for the study involving real human blood serum samples.
Author contributions
All the authors contributed to the study. Anju S. Madanan conceived the idea of the work. Design, material preparation and data collection and analysis were performed by Merin K. Abraham. The original draft of the manuscript was written by Merin K. Abraham and all the authors commented on previous versions of the manuscript. All the authors read and approved the final manuscript.
Data availability
All datasets are available from the corresponding author upon reasonable request.
Conflicts of interest
The authors declare no competing financial interests or personal relationships that could have appeared to influence the work reported in this paper.
Acknowledgements
The authors thank the Head of the Department of Chemistry, University of Kerala, Thiruvananthapuram, Kerala for his support and suggestions, and also for the use of the laboratory, and instrumental facilities offered. We also thank the International Interuniversity Centre for Sensing and Imaging (IIUCSI), the Advanced Research Laboratory for Molecular Sensing and Imaging, the Kerala State Plan Fund 2020-21, the University of Kerala. The author also thank Higher Education Department, University of Kerala Annual Research Grant,Government of Kerala for research fellowships and CLIF, University of Kerala for the sophisticated instrumental analysis offered. Merin K Abraham thanks the University of Kerala for providing a University Fellowship. Anju S Madanan thanks the University of Kerala, Thiruvananthapuram, for the Junior Research Fellowship (JRF). Susan Varghese thanks for the e-grantz Fellowship. Ali Ibrahim Shkhair and Geneva Indongo thank the Government of India for Indian Council for Cultural Relations (ICCR) Scholarship. Greeshma Rajeevan thanks the University of Kerala for providing the University Fellowship.
References
- E. Braunwald, E. M. Antman, J. W. Beasley, R. M. Califf, M. D. Cheitlin, J. S. Hochman, R. H. Jones, D. Kereiakes, J. Kupersmith, T. N. Levin, C. J. Pepine, J. W. Schaeffer, E. E. Smith, D. E. Steward, P. Theroux, R. J. Gibbons, J. S. Alpert, K. A. Eagle, D. P. Faxon, V. Fuster, T. J. Gardner, G. Gregoratos, R. O. Russell and S. C. Smith, J. Am. Coll. Cardiol., 2000, 36, 970–1062 CrossRef CAS PubMed.
- M. Zhang, Z. Wang, Q. Cheng, Z. Wang, X. Lv, Z. Wang and N. Li, Med. Sci. Monit., 2020, 26, e923188–e923181 CAS.
- D. Gabriel-Costa, Pathophysiology, 2018, 25, 277–284 CrossRef CAS PubMed.
- S. Patel, F. Ram, S. K. Patel and K. Kumar, Clin. Epidemiol. Global Health, 2020, 8, 671–677 CrossRef.
- H. Tanaka, F. A. Dinenno, K. D. Monahan, C. M. Clevenger, C. A. DeSouza and D. R. Seals, Circulation, 2000, 102, 1270–1275 CrossRef CAS PubMed.
- M. F. M. Fathil, M. K. Md Arshad, S. C. B. Gopinath, U. Hashim, R. Adzhri, R. M. Ayub, A. R. Ruslinda, M. Nuzaihan, A. H. Azman, M. Zaki and T. H. Tang, Biosens. Bioelectron., 2015, 70, 209–220 CrossRef CAS PubMed.
- A. J. S. Ahammad, J.-J. Lee, L. Minsu, Y.-H. Choi, K. Koh, J.-H. Kim and M. Lee, Int. J. Electrochem. Sci., 2011, 6, 1906–1916 CrossRef CAS.
- X. Han, S. Li, Z. Peng, A. M. Othman and R. Leblanc, ACS Sens., 2016, 1, 106–114 CrossRef CAS.
- S. Ather, R. S. Hira, M. Shenoy, O. Fatemi, A. Deswal, D. Aguilar, K. Ramasubbu, M. Bolos, W. Chan and B. Bozkurt, Int. J. Cardiol., 2013, 166, 394–398 CrossRef PubMed.
- E. Antman, J. P. Bassand, W. Klein, M. Ohman, J. L. Lopez Sendon, L. Rydén, M. Simoons, M. Tendera, B. R. Chaitman, P. Clemmensen, E. Falk, M. C. Fishbein, M. Galvani, J. Garson, A. C. Grines, C. Hamm, U. Hoppe, A. Jaffe, H. Katus, J. Kjekshus, W. Klein, P. Klootwijk, C. Lenfant, D. Levy, R. I. Levy, R. Luepker, F. Marcus, U. Naslund, M. Ohman, O. Pahlm, P. Poole-Wilson, R. Popp, K. Pyorala, J. Ravkilde, N. Rehnquist, W. Roberts, R. Roberts, J. Roelandt, L. Ryden, S. Sans, M. L. Simoons, K. Thygesen, H. Tunstall-Pedoe, R. Underwood, B. F. Uretsky, F. Van de Werf, L. M. Voipio-Pulkki, G. Wagner, L. Wallentin, W. Wijns and D. Wood, J. Am. Coll. Cardiol., 2000, 36, 959–969 CrossRef PubMed.
- V. S. Mahajan and P. Jarolim, Circulation, 2011, 124, 2350–2354 CrossRef PubMed.
- P. Carraro, M. Plebani, M. C. Varagnolo, M. Zaninotto, M. Rossetti and A. Burlina, J. Clin. Lab. Anal., 1994, 8, 70–75 CrossRef CAS PubMed.
- B. Cummins, M. L. Auckland and P. Cummins, Am. Heart J., 1987, 113, 1333–1344 CrossRef CAS PubMed.
- W. U. Dittmer, T. H. Evers, W. M. Hardeman, W. Huijnen, R. Kamps, P. de Kievit, J. H. M. Neijzen, J. H. Nieuwenhuis, M. J. J. Sijbers, D. W. C. Dekkers, M. H. Hefti and M. F. W. C. Martens, Clin. Chim. Acta, 2010, 411, 868–873 CrossRef CAS PubMed.
- Z. R. Guo, C. R. Gu, X. Fan, Z. P. Bian, H. F. Wu, D. Yang, N. Gu and J. N. Zhang, Nanoscale Res. Lett., 2009, 4, 1428–1433 CrossRef CAS PubMed.
- Y. Chen, Y. Gao, Y. He, G. Zhang, H. Wen, Y. Wang, Q. P. Wu and H. Cui, J. Med. Chem., 2021, 64, 1001–1017 CrossRef CAS PubMed.
- S. Yadav, A. Parihar, M. A. Sadique, P. Ranjan, N. Kumar, A. Singhal and R. Khan, ACS Appl. Opt. Mater., 2023, 1, 1245–1262 CrossRef CAS.
- Y. Cai, K. Kang, Q. Li, Y. Wang and X. He, Molecules, 2018, 23, 1102 CrossRef PubMed.
- J. Wei, X. Zhang, S. M. Mugo and Q. Zhang, Anal. Chem., 2022, 94, 12772–12780 CrossRef CAS PubMed.
- J. Li, Y. Lv, N. Li, R. Wu, J. Li, J. You, H. Shen, X. Chen and L. S. Li, Sens. Actuators, B, 2021, 344, 130275 CrossRef CAS.
- X. Liu, Y. Wang, P. Chen, A. McCadden, A. Palaniappan, J. Zhang and B. Liedberg, ACS Sens., 2016, 1, 1416–1422 CrossRef CAS.
- B. Zhou, B. Shi, D. Jin and X. Liu, Nat. Nanotechnol., 2015, 10, 924–936 CrossRef CAS PubMed.
- T. Wan, A. R. Aleem, S. Huang, R. Chen, Z. Zhou, M. Sun, J. He, L. Yu and H. Wen, Mater. Today Adv., 2023, 17, 100326 CrossRef CAS.
- G. Chen, H. Qiu, P. N. Prasad and X. Chen, Chem. Rev., 2014, 114, 5161–5214 CrossRef CAS PubMed.
- J. Choi and S. Y. Kim, J. Biomater. Appl., 2022, 37(4), 646–658 CrossRef CAS PubMed.
- N. Sirkka, A. Lyytikäinen, T. Savukoski and T. Soukka, Anal. Chim. Acta, 2016, 925, 82–87 CrossRef CAS PubMed.
- K. Raiko, A. Lyytikäinen, M. Ekman, A. Nokelainen, S. Lahtinen and T. Soukka, Clin. Chim. Acta, 2021, 523, 380–385 CrossRef CAS PubMed.
- S. Bayoumy, I. Martiskainen, T. Heikkilä, C. Rautanen, P. Hedberg, H. Hyytiä, S. Wittfooth and K. Pettersson, Sci. Rep., 2021, 11, 1–9 CrossRef.
- S. Gogoi and R. Khan, Phys. Chem. Chem. Phys., 2018, 20, 16501–16509 RSC.
- S. Barua, S. Gogoi and R. Khan, Synth. Met., 2018, 244, 92–98 CrossRef CAS.
- R. Devi, S. Gogoi, H. S. Dutta, P. J. Saikia, A. Singhal and R. Khan, Biosens. Bioelectron.: X, 2022, 11, 100168 CAS.
- Q. Su, W. Feng, D. Yang and F. Li, Acc. Chem. Res., 2017, 50, 32–40 CrossRef CAS PubMed.
- S. Gogoi and R. Khan, New J. Chem., 2018, 42, 6399–6407 RSC.
- M. Wang, G. Abbineni, A. Clevenger, C. Mao and S. Xu, Nanomedicine, 2011, 7, 710–729 CrossRef CAS PubMed.
- K. Lingeshwar Reddy, V. Srinivas, K. R. Shankar, S. Kumar, V. Sharma, A. Kumar, A. Bahuguna, K. Bhattacharyya and V. Krishnan, J. Phys. Chem. C, 2017, 121, 11783–11793 CrossRef CAS.
- S. Wu, N. Duan, X. Li, G. Tan, X. Ma, Y. Xia, Z. Wang and H. Wang, Talanta, 2013, 116, 611–618 CrossRef CAS PubMed.
- D. A. Gálico, R. Ramdani and M. Murugesu, Nanoscale, 2022, 14, 9675–9680 RSC.
- N. V. Beloglazova, A. Foubert, A. Gordienko, M. D. Tessier, T. Aubert, E. Drijvers, I. Goryacheva, Z. Hens and S. De Saeger, Talanta, 2016, 160, 66–71 CrossRef CAS PubMed.
- C. Mi, Z. Tian, C. Cao, Z. Wang, C. Mao and S. Xu, Langmuir, 2011, 27, 14632–14637 CrossRef CAS PubMed.
- M. Liu, Y. Ye, C. Yao, W. Zhao and X. Huang, J. Mater. Chem. B, 2014, 2, 6626–6633 RSC.
- G. Frens, Nat. Phys. Sci., 1973, 241, 20–22 CrossRef CAS.
- F. Vetrone, R. Naccache, V. Mahalingam, C. G. Morgan and J. A. Capobianco, Adv. Funct. Mater., 2009, 19, 2924–2929 CrossRef CAS.
- M. Wang, G. Abbineni, A. Clevenger, C. Mao and S. Xu, Nanomedicine, 2011, 7, 710–729 CrossRef CAS PubMed.
- A. Kumar, Y. Kuang, Z. Liang and X. Sun, Mater. Today Nano., 2020, 11, 100076 CrossRef.
- Y. Wang, K. Zheng, S. Song, D. Fan, H. Zhang and X. Liu, Chem. Soc. Rev., 2018, 47, 6473–6485 RSC.
- F. Wang and X. Liu, Chem. Soc. Rev., 2009, 38, 976–989 RSC.
- R. J. Kubiak, N. Lee, Y. Zhu, W. R. Franch, S. V. Levitskaya, S. R. Krishnan, V. Abraham, P. F. Akufongwe, C. J. Larkin and W. I. White, J. Immunol. Res., 2016, 2016, 1485615 Search PubMed.
- K. Das, G. A. Kumar, L. Mirandola, M. Chiriva-Internati and J. Chaudhuri, Front. Mater. Sci., 2019, 13, 389–398 CrossRef.
- X. Li, J. Zhu, Z. Man, Y. Ao and H. Chen, Sci. Rep., 2014, 4, 1–7 CAS.
- P. Ghosh, A. Kar and A. Patra, J. Phys. Chem. C, 2010, 114, 715–722 CrossRef CAS.
- G. Chen, H. Qiu, P. N. Prasad and X. Chen, Chem. Rev., 2014, 114, 5161–5214 CrossRef CAS PubMed.
- C. Daruich De Souza, B. Ribeiro Nogueira and M. E. C. M. Rostelato, J. Alloys Compd., 2019, 798, 714–740 CrossRef CAS.
- G. S. Perera, S. A. Athukorale, F. Perez, C. U. Pittman and D. Zhang, J. Colloid Interface Sci., 2018, 511, 335–343 CrossRef CAS PubMed.
- U. Cataldi, R. Caputo, Y. Kurylyak, G. Klein, M. Chekini, C. Umeton and T. Bürgi, J. Mater. Chem. C, 2014, 2, 7927–7933 RSC.
- M. J. E. Fischer, Methods Mol. Biol., 2010, 627, 55–73 CrossRef CAS PubMed.
- S. Asiaei, B. Smith and P. Nieva, Biomicrofluidics, 2015, 9(6), 064115 CrossRef PubMed.
- D. Mendez-Gonzalez, S. Melle, O. G. Calderón, M. Laurenti, E. Cabrera-Granado, A. Egatz-Gómez, E. López-Cabarcos, J. Rubio-Retama and E. Díaz, Nanoscale, 2019, 11, 13832–13844 RSC.
- F. T. Rabouw, P. T. Prins, P. Villanueva-Delgado, M. Castelijns, R. G. Geitenbeek and A. Meijerink, ACS Nano, 2018, 12, 4812–4823 CrossRef CAS PubMed.
- L. J. Huang, X. Tian, J. T. Yi, R. Q. Yu and X. Chu, Anal. Methods, 2015, 7, 7474–7479 RSC.
- M. S. Arai and A. S. S. de Camargo, Nanoscale Adv., 2021, 3, 5135–5165 RSC.
- C. Chen and N. Hildebrandt, TrAC, Trends Anal. Chem., 2020, 123, 115748 CrossRef CAS.
- D. Shrestha, A. Jenei, P. Nagy, G. Vereb and J. Szöllősi, Int. J. Mol. Sci., 2015, 16, 6718–6756 CrossRef CAS PubMed.
- X. Cui, M. Liu and B. Li, Analyst, 2012, 137, 3293–3299 RSC.
- R. Huang, S. Liu, J. Huang, H. Liu, Z. Hu, L. Tao and B. Zhou, Nanoscale, 2021, 13, 4812–4820 RSC.
- D. Shrestha, A. Jenei, P. Nagy, G. Vereb and J. Szöllősi, Int. J. Mol. Sci., 2015, 16, 6718 CrossRef CAS PubMed.
- A. Pilch-Wrobel, A. M. Kotulska, S. Lahtinen, T. Soukka and A. Bednarkiewicz, Small, 2022, 18, 2200464 CrossRef CAS PubMed.
|
This journal is © The Royal Society of Chemistry 2024 |
Click here to see how this site uses Cookies. View our privacy policy here.