DOI:
10.1039/D3AN01690K
(Paper)
Analyst, 2024,
149, 180-187
Enhancing charge transfer in a W18O49/g-C3N4 heterostructure via band structure engineering for effective SERS detection and flexible substrate applications†
Received
4th October 2023
, Accepted 1st November 2023
First published on 10th November 2023
Abstract
Chemical mechanism (CM)-related surface-enhanced Raman spectroscopy (SERS) has received tremendous interest due to its exceptional stability and excellent uniformity. Nevertheless, there remains a demand for ingenious methodologies for promoting effective charge transfer (CT) to improve SERS sensitivity further. Herein, a band structure engineered W18O49/g-C3N4 heterostructure (WCN) was first employed as a CM-based SERS substrate with remarkable enhancement and sensitivity. To investigate the Raman enhancement properties of the substrate, malachite green (MG) was employed as the Raman probe with the excitation of a 633 nm laser. The WCN substrate exhibits a Raman enhancement factor (EF) of 2.6 × 107, achieving a limit of detection (LOD) of 1.9 × 10−10 M for MG. The outstanding Raman amplification behavior can be attributed to the heterojunction-induced efficient CT process, energy band matching resonance due to minor doping with g-C3N4 serving as a band gap modifier, and improved photo-induced charge transfer (PICT) efficiency via the oxygen vacancies in the W18O49 units. Additionally, a flexible SERS substrate based on WCN was constructed using a vacuum filtration method and utilized to detect prohibited pharmaceutical residues on fish skin. The integration of this WCN and a nylon membrane not only preserves the Raman activity of the WCN for sensitive detection but also endows the Raman substrate with high flexibility and good mechanical durability, making it a potential candidate for in situ detection in particular environments.
Introduction
Surface-enhanced Raman spectroscopy (SERS) is an emerging method for trace species and food safety analysis, biological imaging, and material surface science exploration with the advantages of high sensitivity and spectral resolution, quick response, and non-destructive detection modes.1–4 Apart from noble-metal SERS substrates dominated by the electromagnetic mechanism, chemical enhancement mechanism (CM)-based substrates, which are related to the photo-induced charge transfer (PICT) pathways among the substrates and adsorbed molecules, have drawn increasing interest in the SERS-related fields.5–8 In comparison to noble metals, CM-based materials present higher selectivity, better stability, and uniformity.9–11 In recent years, novel CM-based substrates have been proposed and utilized along with the development of new synthetic techniques. Some CM-based substrates such as graphene, metal–organic frameworks (MOFs), and semiconductor metal oxides have been investigated and successfully employed as alternatives to noble metals for monitoring various targets.12–16 Zhu et al. reported oxygen vacancy-engineered Bi2MoO6 as a functional SERS-active substrate, and introducing oxygen vacancies facilitates efficient charge separation and electron transfer, further promoting Raman performance.17 Guo and his co-workers developed amorphous ZnO nanocages to be a SERS substrate. The metastable electronic states of a-ZnO NCs play an essential role in the high-efficiency charge transfer (CT) process and Raman enhancement.18 However, the CT process is a short-range pathway, and CM-based substrates tend to suffer from a low enhancement factor (EF) and insufficient charge separation efficiency, which in turn restricts their practical applications, although several approaches have been applied to enhance the SERS response of such materials.19,20 Thus, it remains urgently necessary to significantly enhance the SERS capability of CM-based substrates by establishing advanced strategies.
Compared to CM-based nanomaterials with single components, heterostructure-like substrates allow more enhanced CT efficiency and higher stability.20,21 In a heterostructure, the charge recombination is limited due to the influential interfacial CT events, and the advantages of each component are consolidated, leading to a significant enhancement in the performance,22 which are not available with single-component non-metallic substrates. Furthermore, the composition of heterostructure materials can be diverse, and they can be highly regulated and controlled in terms of nanostructure and morphology.23 All the above factors suggest that heterostructure-based substrates are promising SERS candidates and provide us with new ideas for designing high-performance Raman substrates.
Herein, a g-C3N4/W18O49 heterostructure (WCN) was constructed through a hydrothermal reaction and employed as a CM-based substrate with superior Raman enhancement (EF = 2.6 × 107), high sensitivity (LOD for malachite green (MG) is 1.9 × 10−10 M) and high reproducibility (Scheme 1). The remarkable SERS activities were primarily ascribed to a heterojunction-induced efficient CT process, energy band matching resonance due to minor doping with g-C3N4 as a band gap modifier, and the improved PICT efficiency via the oxygen vacancies in the W18O49 units. The enhanced CT process in WCN was confirmed by density functional theory (DFT) calculations. Additionally, the high-EF WCN substrate was also fabricated as a flexible SERS substrate supported by a nylon filter membrane for sensitively detecting residual MG on fish skin. The integration of this WCN and a nylon membrane not only preserves the Raman activity of the WCN for sensitive detection but also endows the Raman substrate with high flexibility and good mechanical durability, making it a potential candidate for in situ detection in unique environments and food safety analysis.
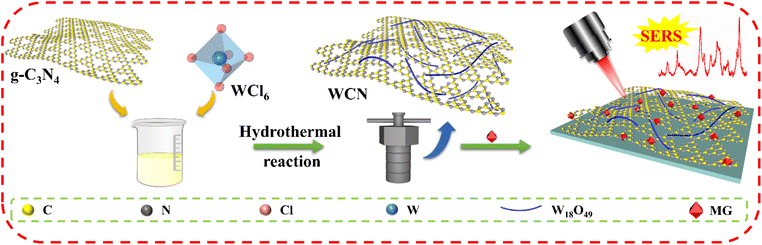 |
| Scheme 1 The design and fabrication process of the g-C3N4/W18O49 heterostructure (WCN) for Raman enhancement. The WCN was used as a CM-based substrate for MG detection under 633 nm laser excitation. | |
Results and discussion
Characterization of the WCN
g-C3N4 was fabricated by a two-step calcination method, while W18O49 and WCN were synthesized via a facile solvothermal method.24 Note that the incorporation of g-C3N4 was conducive to the adjustment of the band structure in the WCN heterostructure, which affected the effective charge transfer among molecules and substrates to optimize SERS activity.25 The X-ray diffraction (XRD) measurement results in Fig. 1a reveal information regarding the phase composition and crystallographic structure of the as-synthesized samples. The distinct diffraction peak at 27.2° in all patterns is attributed to the (002) plane, corresponding to the in-plane stacking of conjugated aromatic systems of graphite-like materials.26 Meanwhile, the (010) and (020) diffraction peaks of W18O49 located at 23.1° and 47.2° are consistent with those of monoclinic-phase W18O49 (JCPDS: 71-2450).27 The XRD patterns of the WCN possessed all the typical diffraction peaks of g-C3N4 and W18O49 without any other impurity peaks. These results confirm that the WCN was successfully fabricated. Transmission electron microscopy (TEM) and scanning electron microscopy (SEM) images, together with energy-dispersive spectroscopy (EDS) elemental mapping images, were subsequently employed to examine the microstructure, morphology, and composition of the fabricated samples. As Fig. S1a–c† demonstrate, the prepared g-C3N4 predominantly exhibits a layered structure with a wrinkled surface, reflecting the layer-stacking of analogous graphite conjugated aromatic systems through weak van der Waals bonds at random.26 As for pure W18O49, an urchin-like structure in Fig. S2a† with a uniform diameter of about 836 nm can be clearly observed. The magnified TEM image in Fig. S2b† indicates that the W18O49 has a hierarchical structure consisting of many radial nanowires.
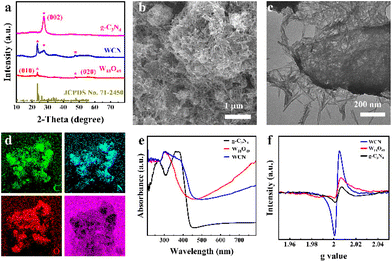 |
| Fig. 1 (a) XRD patterns of the g-C3N4 nanosheets, W18O49, and WCN. (b) SEM and (c) TEM images of WCN. (d) EDS elemental mapping images of C, N, O, and W in WCN. (e) UV-vis DRS spectra of the prepared g-C3N4 nanosheets, W18O49, and WCN. (f) In situ EPR spectra of g-C3N4, W18O49, and WCN. | |
The g-C3N4 nanosheets were subjected to a hydrothermal reaction process and employed as supports for the adsorption of W6+ ions. Tungsten oxide monomers were anchored on the template and they grew horizontally along a specific crystal plane, ultimately forming thin nanowires instead of collective structures.28 As shown in Fig. 1b, the solvothermal route enables the formation of the WCN heterostructure created by crossed W18O49 nanowires loaded on the g-C3N4 nanosheets. As observed in the TEM image (Fig. 1c), the edges of g-C3N4 nanosheets can be obviously identified, and the surfaces of these nanosheets exhibit high-density and well-distributed loading of W18O49 nanowires, with an average length of approximately 200 nm. In addition, the EDS images (Fig. 1d) prove the coexistence and uniform distributions of C, N, O, and W. The optical properties of g-C3N4, W18O49, and WCN were investigated by ultraviolet-visible diffuse reflectance spectroscopy (UV-vis DRS). As shown in Fig. 1e, g-C3N4 exhibits an intrinsic absorption edge at 485 nm, which indicates a narrow bandgap for g-C3N4. However, a very strong absorption tail for W18O49 can be noticed in the region of visible/NIR light, and the improved light harvesting capacity could be attributed to the collective oscillations of surficial charges of W18O49 due to the oxygen vacancies.29 The stable oxygen vacancies could act as photon traps and enhance light–matter interactions.30 The spectra of WCN also contain the absorption tail region derived from the oxygen vacancies in the W18O49 units and the absorption from the g-C3N4. According to the typical Tauc plots in Fig. S3a–c,† the bandgap levels for g-C3N4, W18O49, and WCN were estimated to be 2.82 eV, 3.09 eV, and 2.74 eV. The bandgap energy (Eg) narrowing could contribute to more efficient absorbance of light, thus enabling an effective CT process between the substrate and molecules.31 Note that the added g-C3N4 in the WCN heterostructure has no apparent effect on the absorption spectra but simply acts as a modifier of the overall bandgap of the whole material. An in situ electron paramagnetic resonance (EPR) analysis was conducted to verify the generation of oxygen vacancies in the synthesized samples. As displayed in Fig. 1f, the representative signals of oxygen vacancies in g-C3N4, W18O49, and WCN are present at a g factor of ∼2.004, which can be ascribed to the trapping of electrons by oxygen vacancies. In addition, the oxygen vacancy signal intensity of WCN is higher than that of g-C3N4 and W18O49, indicating not only the formation of more oxygen vacancies due to the coupling effect of g-C3N4 and W18O49,32 but also efficient electron separation on the catalyst surface.33
X-ray photoelectron spectroscopy (XPS) measurements were carried out to further substantiate the chemical states and surface element compositions of W18O49, g-C3N4, and WCN. As shown in Fig. S4,† the full-scale XPS spectra of W18O49, g-C3N4, and WCN indicate the coexistence of C, N, W, and O elements, which is in accordance with the EDS results in Fig. 1d. In detail, as shown in Fig. 2a, two significant peaks in the high-resolution spectrum of C 1s at 284.8 and 288.3 eV are attributable to N–C
N and sp2 C–C of graphitic carbon.34 As for the N 1s spectra (Fig. 2b), the three peaks located at 398.55, 400.5 and 401.3 eV originated from sp2 C–N
C, N–(C)3, and C–N–H, respectively.35 For pure W18O49, the W 4f spectrum (Fig. 2c) demonstrates four peaks, and the two peaks centered at 33.9 and 36.6 eV are consistent with W 4f 7/2 and W 4f 5/2 of W5+, respectively. The other two peaks located at 35.4 and 37.4 eV are derived from W 4f 7/2 and W 4f 5/2 of W6+, respectively.36 The existence of oxygen vacancies in W18O49 and WCN could induce the generation of pentavalent tungsten ions, which on the other hand, could also promote CT within the substrate and molecules. The O 1s spectrum of W18O49 (Fig. 2d) exhibits two prominent peaks at 531.7 and 533.1 eV, which are assigned to W–O and –OH.37 The XPS spectra of the WCN sample showed the standard XPS peaks of pure W18O49 and g-C3N4 nanosheets, indicating that the formation of WCN was successful. However, a distinct shift of the XPS peaks can be noticed in comparison with the individual constituents after the establishment of the WCN heterojunction. The C 1s and N 1s spectra of WCN shift to higher binding energies, and the characteristic W 4f and O 1s peaks shift toward lower energies. The binding energy is relevant to the electron density. Therefore, the electron density in g-C3N4 and W18O49 was theoretically altered owing to changes in the binding energies of C 1s, N 1s, W 4f, and O 1s in WCN.38 Fourier transform infrared (FTIR) spectroscopy was further performed to analyze the functional groups on the surface of g-C3N4, W18O49, and WCN. As presented in Fig. 2e, for pure g-C3N4, a series of significant vibration bands derive from triazine units (804 cm−1) and the typical stretching vibration modes of the CN heterocycles (1245–1640 cm−1). Meanwhile, the FTIR spectrum of W18O49 displays the stretching vibration bands ascribed to O–W–O (600–835 cm−1). And the other peaks can be attributed to W
O (950 cm−1) and the –OH of the absorbed water molecules (1618 cm−1). Apparently, typical peaks of g-C3N4 and W18O49 can be identified in the FTIR spectra of the WCN. As shown in Fig. 2f, further comparison and analysis of the above feature peaks derived from g-C3N4 and W18O49 in the prepared WCN suggest a slight shift in the peak position of triazine units, which indicates a possible chemical interaction at the interface between W18O49 and g-C3N4.28 These characterization results demonstrate energy band engineering in WCN and the existence of oxygen vacancies, which is favorable for investigating WCN as an efficient CM-based substrate in SERS applications.
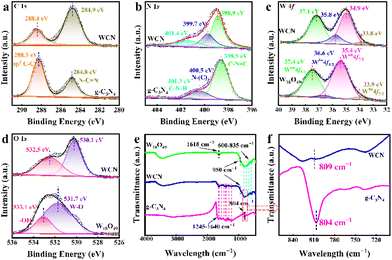 |
| Fig. 2 (a) C1s, (b) N 1s, (c) W 4f, (d) O 1s XPS spectra, and (e) FTIR spectra of the g-C3N4 nanosheets, W18O49 and WCN. (f) The magnified image of the selected area in (e). | |
SERS performance of WCN substrates
The Raman enhancement capability of the WCN substrates was primarily investigated using MG as the Raman probe. Its typical Raman spectrum and molecular formula are illustrated in Fig. S5.† Additionally, the corresponding detailed peak assignments are listed in ESI Table S1.† And the signature Raman peaks at 1176 cm−1 and 1617 cm−1, which are derived from the stretching vibration modes of C–H and C–C bonds in MG, were selected to analyze the SERS intensity. Quite interestingly, the pure g-C3N4, W18O49, and WCN samples with different ratios were found to demonstrate intense discrepancies in Raman enhancements. Fig. 3a displays the Raman signals of MG (10−6 M) collected from the different samples (g-C3N4, W18O49, and WCN), with the excitation wavelength being 633 nm. Obviously, the WCN yields the highest Raman enhancement intensity among all substrates. The selected SERS signal characteristic peaks in Fig. 3b for MG at 1176 cm−1 and 1617 cm−1 further demonstrate the differentiation of enhancement contributions from different substrates, and the error bars indicate the standard deviation obtained from six separate measurements. In addition, the Raman signal of MG (10−6 M) in Fig. 3c clearly indicates the highest enhancement of the SERS signal with 633 nm laser excitation. This outstanding SERS performance results primarily from the effective charge transfer associated with modulated energy bands and oxygen vacancies in the WCN substrate.
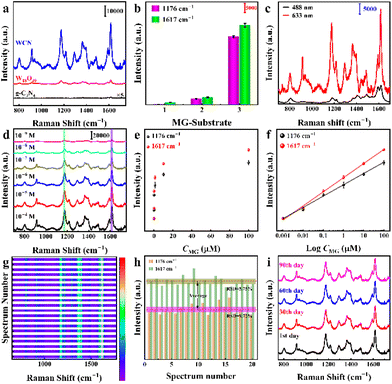 |
| Fig. 3 (a) Raman spectra of MG (10−6 M) adsorbed on the g-C3N4 nanosheets, urchin-like W18O49 and WCN. (b) The selected characteristic peak intensities at 1176 cm−1 and 1617 cm−1 in (a). (c) Raman spectra of MG (10−6 M) adsorbed on the WCN under excitation laser wavelengths of 488 nm (black line) and 633 nm (red line). (d) SERS spectra of MG at various concentrations (10−4 M to 10−9 M) collected on the WCN substrate. (e) The relationship of the intensities at 1176 cm−1 and 1617 cm−1 with concentrations of MG. (f) The linear correlation of the intensities at 1176 cm−1 and 1617 cm−1 with logarithmic concentrations of MG. (g) SERS spectral heatmap of 20 random spots for MG (10−6 M) collected on the WCN substrate and (h) the corresponding intensity histograms at 1176 cm−1 and 1617 cm−1. (i) SERS stability test of the WCN substrate during 90 days of storage. | |
After identifying the most optimal substrate and most suitable excitation laser, the Raman enhancement properties of the proposed WCN substrate were evaluated using MG solutions at varying concentrations (Fig. 3d). It is evident that the Raman peak intensity diminishes stepwise with decreasing concentration of the molecules in the range of 10−4–10−9 M. As depicted in Fig. 3e, the signature peaks at 1176 cm−1 and 1617 cm−1 of MG at a concentration of 10−9 M suggest the excellent sensitivity of the WCN substrate. As indicated in Fig. 3f, the Raman scattering intensity is linearly and positively correlated with the concentration of MG in the range of 10−4–10−9 M. The linear fitting equations are y1 = (28
064 ± 834) + (8144 ± 379)log
CMG and y2 = (38
341 ± 878) + (10
929 ± 352)log
CMG for the peaks at 1176 cm−1 and 1617 cm−1, respectively. And the respective squared correlation coefficients (R2) are calculated to be 0.991 and 0.995 (the details are in the ESI†). Based on the formula 3σ/S, where σ represents the standard deviation of the blank samples and S is the slope,39 the limit of detection (LOD) was estimated to be as low as 3.6 × 10−10 M for the peak at 1176 cm−1 and 1.9 × 10−10 M for the peak at 1617 cm−1, indicating the superior ability of WCN substrates to detect MG at low concentrations. In addition, the most important parameter for evaluating the enhancement performance of a substrate, enhancement factor (EF), was calculated using MG on bare glass and a WCN substrate, and the peak intensity at 1617 cm−1 of MG was chosen to assess the value of EF. To ensure the accuracy of the estimated values, the intensity of the characteristic peak at 1617 cm−1 was determined by averaging 20 randomly collected measurement spots. It can be observed that the EF approaches 2.6 × 107 for the peak at 1617 cm−1. To the best of our knowledge, this developed SERS substrate demonstrates a considerable enhancing performance, which is comparable to that of other noble metal-free SERS substrates summarized in Table S2.† Additionally, compared with previous methods (Table S3†), the present biosensing system displayed higher sensitivity. It is a pioneer in the field of semiconductor-based SERS substrate materials. Such superior SERS behavior can reasonably be explained by the band matching due to the energy band engineering in the WCN heterostructures, which can facilitate effective charge transfer between the molecules and substrate. Fig. 3g illustrates the heatmap of 20 random spots for MG (10−6 M) collected on the WCN substrate. Apparently, there is a very limited change in the Raman intensities of MG molecules, and the relative standard deviation (RSD) can be calculated to be 9.72% (1176 cm−1) and 5.75% (1617 cm−1), indicating the reproducibility of the WCN substrate (Fig. 3h). As can be seen in Fig. S6,† the reproducibility of different batches of WCN substrates was further investigated. The RSD values are 8.81% (1176 cm−1) and 7.86% (1617 cm−1), respectively. These results demonstrate signal enhancement with high reproducibility over a large area and from batch to batch. As another essential factor, the stability of the WCN substrate should be taken into account. As displayed in Fig. 3i and Fig. S7†, the Raman spectra and the intensity of MG peaks on the substrate remained virtually unchanged over 90 days, demonstrating its long-term stability under ambient conditions.
SERS enhancement mechanism
Serving as a CM-supported substrate, the Raman enhancement of WCN strongly depends on effective CT pathways. To gain a more profound comprehension of the intriguing light-sensitive behavior, we conducted an extensive analysis of the electronic structure and corresponding CT through DFT calculations (for the computational details, see the ESI†). It is obviously seen in Fig. 4a–c that the amount of electrons that transfer from MG to WCN (1.60 e−) was considerably higher than that in g-C3N4 (0.55 e−) or W18O49 (0.72 e−). And DFT calculations revealed strong evidence for effective CT between WCN and MG, resulting in remarkably improved Raman performance for MG on the WCN substrate. In addition, the transient photocurrent response curves illustrated in Fig. S8† demonstrate the distinction in the photoelectric response activities of g-C3N4, W18O49, and WCN under simulated light irradiation. The WCN has the highest photocurrent conversion efficiency in comparison with g-C3N4 and W18O49, which can be derived from the oxygen vacancies and the heterostructures in WCN. The stronger separation of electrons and holes can facilitate CT efficiency, thus further improving Raman performance. On the other hand, the matching degree of energy levels between the molecules and substrate is a major indicator for realizing CM-based Raman enhancement, and the charge transfer mediated SERS process was further determined through the energy levels. At first, the bandgap energies (Eg) of the three samples were determined according to the Tauc plots in Fig. S3.† Additionally, the corresponding valence band (VB) energies were determined by VB-XPS analysis (Fig. S9†). And the values of EVBvs. NHE for g-C3N4 nanosheets, W18O49, and WCN are 1.13, 2.5, and 2.36 eV, respectively. Therefore, the related ECB for the three samples can be calculated to be −1.69, −0.59, and −0.38 eV, individually (for the calculation details, see the ESI†). Meanwhile, the CB and VB energies of WCN are positioned separately at −4.12 eV and −6.86 eV below the vacuum level, respectively, and the oxygen vacancy state (VO) is located at about 0.5–1.0 eV below the CB.40 The HOMO and LUMO levels for MG molecules are estimated to be −7.66 eV and −6.11 eV, respectively.41 In SERS detection (Fig. 4d), the newly introduced defective energy level caused by the oxygen vacancies can act as an intermediate level for electron transport among MG and the WCN substrate. In addition, the electrons at the HOMO and LUMO levels of MG can easily travel to the energy levels of WCN due to the energy matching degree, thus leading to a favorable molecule–substrate charge transfer pathway. Considering all this, a CM-based substrate enhanced by the PICT mechanism could be proposed. With the incorporation of g-C3N4, the WCN heterostructures retain the original light collection capability, and the electron excitation efficiency is enlarged.1 Notice that the energy barrier between the LUMO level (−6.11 eV) of the MG molecule and the CB level (−4.12 eV) of the substrate is 1.99 eV, which is close to the energy of a 633 nm laser (1.96 eV), which also explains why a 633 nm laser can stimulate and generate greater enhancement. Thus, the CT vibronic coupling can be feasible between the substrate and molecules, resulting in the intensification of the Raman signal. As a result, the PICT transfers electrons from the VB state (|V〉) of WCN to the LUMO state (|K〉) of MG through the transition moment, borrowing intensity from molecular transitions through the Herzberg–Teller coupling term (hCK).42 This implies that the electronic changes and polarizability of the molecule are greatly strengthened, leading to a remarkable improvement in SERS. In this way, the Raman enhancement is encouraged by the synergistic effects of a heterojunction-induced efficient CT process, energy band matching resonance due to minor doping with g-C3N4 serving as a band gap modifier, and the improved PICT efficiency via the oxygen vacancies in the W18O49 units.
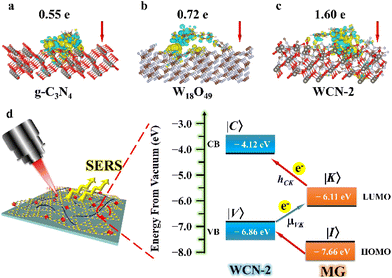 |
| Fig. 4 The electron density difference for MG on (a) g-C3N4 nanosheets, (b) W18O49, and (c) WCN. Isosurfaces taken at 0.001 e Å−3. Yellow means gaining electrons and blue means losing electrons. (d) Schematic diagram of SERS detection and the energy-level diagrams of MG on WCN with respect to the vacuum level and the proposed PICT pathways. | |
Application of the WCN heterostructures as flexible SERS substrates
Generally speaking, conventional SERS substrates are loaded on a rigid carrier, which is inappropriate for direct SERS detection and limits their application in certain circumstances, as these substrates are expected to be in different shapes and softness to satisfy the actual requirements. In addition, convenient transportation and storage of substrates and in situ detection of the target are significant.43 Nylon filter membranes are ideal support substrates for SERS-active materials with the advantages of high flexibility, high hydrophilicity, and low cost.44,45 Taking advantage of the SERS performance of the WCN substrate and nylon filter membranes, we have attempted to further extend its application by constructing flexible SERS substrates. As shown in Fig. 5a, a flexible SERS substrate supported by a nylon filter membrane was fabricated by a simple vacuum filtration process (Fig. S10†). The Raman spectra in Fig. S11† clearly reveal that the Raman enhancement of the flexible substrate originates from the material. The fabrication of the flexible substrate has no negative effect on the Raman enhancement properties of the WCN material, while the filter membrane just serves as a support for the Raman-active material. The WCN substrate can be easily cut into small pieces in required shapes, such as squares, circles, rectangles, and triangles (Fig. S12†). The SERS spectral heatmap (Fig. 5c) was obtained from ten randomly selected spots for MG on the flexible WCN substrate (Fig. 5b). As shown in Fig. 5d, the column chart of the corresponding characteristic peak intensities at1176 cm−1 and 1617 cm−1 suggests a uniform SERS distribution with small RSD values. Besides the homogeneity and adjustable shapes, the flexible substrate offers excellent mechanical stability. As displayed in Fig. 5e and h, torsions and bends are employed to be the simulation of mechanical deformation due to external forces. The SERS spectra in Fig. 5f and i demonstrate the high reproducibility of the flexible substrate. Compared with the original state without deformation, the selected characteristic peak intensity (Fig. 5g and j) exhibits a negligible change in Raman intensity. The stability of the Raman spectra and signal intensity of the characteristic peaks was also demonstrated in 200 mechanical stability experiments (Fig. S13†). All the above results prove the excellent mechanical durability and indicate potential applications in SERS-related detection for this flexible substrate.
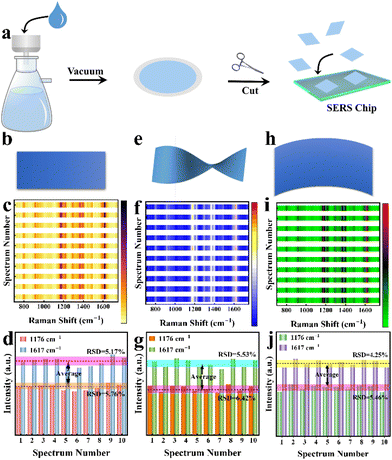 |
| Fig. 5 (a) The fabrication process of the flexible SERS substrate. The schematic of the substrate under (b) normal, (e) torsional, and (h) flexural conditions. The SERS spectral heatmaps of the substrate under (c) normal, (f) torsional, and (i) flexural conditions. Characteristic peak intensities of the substrate under (d) normal, (g) torsional, and (j) flexural conditions. | |
Additionally, we examined MG residues on the surface of fish with the proposed WCN flexible substrates. As shown in Fig. 6a, the detection of MG molecules on fish was simulated by recovering and detecting different concentrations of probe molecules sprayed on the surface of fish. Benefiting from the flexibility of the substrate, we can employ the substrate with a suitable shape covering different parts of the fish for swabbing detection. The Raman signals and the fingerprint peak intensity for MG are displayed in Fig. 6b and c. The signal response of MG is strongly dependent on its concentration over a range of concentrations. And the Raman signals of MG can still be well observed even at 1.0 × 10−9 M, indicating the excellent sensitivity of the flexible substrate. The recoveries (Fig. 6d) were calculated to be between 73.1% and 85.1% by comparing the Raman signals of MG molecules obtained from the detection of flexible substrate adsorption on glass slides (Fig. S14†) and fish. These encouraging findings prove that the proposed WCN matrix can be applied for the practical and accurate detection of drug residues on fish skin and therefore it provides an ideal platform for portable in situ detection.
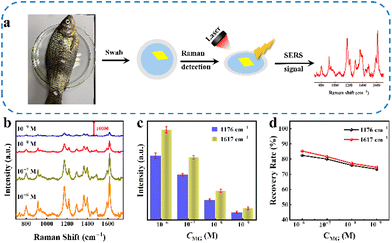 |
| Fig. 6 (a) Simulation for detection, (b) SERS spectra, and (c) the intensity of selected peaks of MG residues at different concentrations (10−6 M to 10−9 M) adsorbed on the crucian surface obtained using the flexible WCN substrate. (d) The calculated recovery rates. | |
Conclusions
In conclusion, band structure engineered W18O49/g-C3N4 (WCN) heterostructures were constructed as Raman-active materials and further supported by nylon filter membranes to fabricate flexible SERS substrates. Proven by experimental and theoretical calculations, g-C3N4 was utilized as an energy band regulator in the WCN heterostructure, which optimizes the matching degree in energy bands among the heterostructures and molecules and facilitates the PICT process. Benefiting from the more effective electron–hole separation in the heterostructure, the charge transfer between the substrate and molecules is more vigorous, which leads to a significantly enhanced Raman signal. On the other hand, the preservation of oxygen vacancies further accelerates the PICT route and improves the signal output. The LOD for MG is 1.9 × 10−10 M with the EF even up to 2.6 × 107. We have developed flexible WCN substrates with good stability, reproducibility, and sensitivity for the sensitive detection of drug residues on fish skin based on this excellent Raman-active material. This work provides a method for improving the Raman enhancement of heterogeneous structures and demonstrates the practical potential of flexible SERS substrates for in situ detection, providing a meaningful reference for the design of plasmon-free substrates.
Author contributions
Lu Tan: conceptualization, investigation, characterization and writing – original draft. Shuzhen Yue: formal analysis and data curation. Yongbing Lou: funding acquisition, validation and supervision. Jun-Jie Zhu: funding acquisition, project administration and supervision.
Conflicts of interest
There are no conflicts to declare.
Acknowledgements
This work was supported by the Guangdong Basic and Applied Basic Research Foundation (2020B1515120026), the Free Exploration Basic Research Foundation of Central Government Guided Local Science and Technology Development (2021Szvup058), the Special Fund of Jiangsu Province for Science and Technology Achievements Transformation (BA2020060), the Priority Academic Program Development of Jiangsu Higher Education Institutions, and Jiangsu Funding Program for Excellent Postdoctoral Talent (20220ZB22).
References
- Y. Su, B. Yuan, Y. Jiang, P. Wu, X. Huang, J.-J. Zhu and L.-P. Jiang, Chem. Sci., 2022, 13, 6573–6582 RSC.
- J. Liu, T. Zheng and Y. Tian, Angew. Chem., Int. Ed., 2019, 58, 7757–7761 CrossRef CAS PubMed.
- R. Mei, Y. Wang, X. Zhao, S. Shi, X. Wang, N. Zhou, D. Shen, Q. Kang and L. Chen, ACS Sens., 2023, 8, 372–380 CrossRef CAS.
- P. Wu, X. Luo, Y. Xu, J. Zhu, W. Jia, N. Fang, C. Cai and J.-J. Zhu, Anal. Chem., 2022, 94, 17541–17550 CrossRef CAS.
- R. Wang, H. Liu, T. Xu, Y. Zhang, C. Gu and T. Jiang, Biosens. Bioelectron., 2023, 227, 115160 CrossRef CAS.
- Y. Xu, J. Lin, X. Wu, X. Xu, D. Zhang, Y. Xie, T. Pan, Y. He, A. Wu and G. Shao, J. Mater. Chem. B, 2022, 10, 3808–3816 RSC.
- Y. Su, S. Wen, X. Luo, F. Xue, S. Wu, B. Yuan, X. Lu, C. Cai, L.-P. Jiang, P. Wu and J.-J. Zhu, ACS Appl. Mater. Interfaces, 2021, 13, 135–147 CrossRef CAS.
- E. Feng, T. Zheng, X. He, J. Chen and Y. Tian, Sci. Adv., 2018, 4, eaau3494 CrossRef CAS PubMed.
- G. Song, H. Sun, J. Chen, Z. Chen, B. Liu, Z. Liu, S. Cong and Z. Zhao, Anal. Chem., 2022, 94, 5048–5054 CrossRef CAS PubMed.
- G. Song, W. Gong, S. Cong and Z. Zhao, Angew. Chem., 2021, 133, 5565–5571 CrossRef.
- X. Liu, Z. Ye, Q. Xiang, Z. Xu, W. Yue, C. Li, Y. Xu, L. Wang, X. Cao and J. Zhang, Chem, 2023, 9, 1464–1476 CAS.
- D. Liu, X. Chen, Y. Hu, T. Sun, Z. Song, Y. Zheng, Y. Cao, Z. Cai, M. Cao, L. Peng, Y. Huang, L. Du, W. Yang, G. Chen, D. Wei, A. T. S. Wee and D. Wei, Nat. Commun., 2018, 9, 193 CrossRef.
- W. Liu, Z. Wang, W. Yan, Z. Zhao, L. Shi, L. Huang, Y. Liu, X. He and S. Cui, Carbon, 2023, 202, 389–397 CrossRef CAS.
- H. Sun, S. Cong, Z. Zheng, Z. Wang, Z. Chen and Z. Zhao, J. Am. Chem. Soc., 2019, 141, 870–878 CrossRef CAS PubMed.
- J. Jin, Z. Guo, D. Fan and B. Zhao, Mater. Horiz., 2023, 10, 1087–1104 RSC.
- J.-H. Fu, Z. Zhong, D. Xie, Y.-J. Guo, D.-X. Kong, Z.-X. Zhao, Z.-X. Zhao and M. Li, Angew.
Chem., Int. Ed., 2020, 59, 20489–20498 CrossRef CAS PubMed.
- L. Tan, B. Yuan, Y. Lou, Y. Su and J.-J. Zhu, J. Alloys Compd., 2023, 945, 169233 CrossRef CAS.
- X. Wang, W. Shi, Z. Jin, W. Huang, J. Lin, G. Ma, S. Li and L. Guo, Angew. Chem., Int. Ed., 2017, 56, 9851–9855 CrossRef CAS.
- X. Zheng, H. Guo, Y. Xu, J. Zhang and L. Wang, J. Mater. Chem. C, 2020, 8, 13836–13842 RSC.
- Y. Zhou, Q. Gu, T. Qiu, X. He, J. Chen, R. Qi, R. Huang, T. Zheng and Y. Tian, Angew. Chem., Int. Ed., 2021, 60, 26260–26267 CrossRef CAS.
- J. Wan, Q. Liu, P. Tang, Y. Ji, W. Zhong, W. Cheng, X. Xing, X. Lu and L. Zhong, Analyst, 2023, 148, 869–875 RSC.
- Z. Wei, S. Xie, W. Xiong, S. Zen, D. Chen, T. Jiang, D. Chen, J. Zhou and C. Gu, Phys. Chem. Chem. Phys., 2023, 25, 10820–10826 RSC.
- Q. Lv, J. Tan, Z. Wang, P. Gu, H. Liu, L. Yu, Y. Wei, L. Gan, B. Liu, J. Li, F. Kang, H.-M. Cheng, Q. Xiong and R. Lv, Nat. Commun., 2023, 14, 2717 CrossRef CAS.
- S. Zhang, W. Yi, Y. Guo, R. Ai, Z. Yuan, B. Yang and J. Wang, Nanoscale, 2021, 13, 3493–3499 RSC.
- A. Chaves, J. G. Azadani, H. Alsalman, D. R. da Costa, R. Frisenda, A. J. Chaves, S. H. Song, Y. D. Kim, D. He, J. Zhou, A. Castellanos-Gomez, F. M. Peeters, Z. Liu, C. L. Hinkle, S.-H. Oh, P. D. Ye, S. J. Koester, Y. H. Lee, P. Avouris, X. Wang and T. Low, npj 2D Mater. Appl., 2020, 4, 29 CrossRef CAS.
- P.-P. Li, Y. Cao, C.-J. Mao, B.-K. Jin and J.-J. Zhu, Anal. Chem., 2019, 91, 1563–1570 CrossRef CAS.
- J. Z. Y. Tan, S. Gavrielides, M. Belekoukia, W. A. Thompson, L. Negahdar, F. Xia, M. M. Maroto-Valer and A. M. Beale, Chem. Commun., 2020, 56, 12150–12153 RSC.
- M. Wang, G. Tan, M. Dang, Y. Wang, B. Zhang, H. Ren, L. Lv and A. Xia, J. Colloid Interface Sci., 2021, 582, 212–226 CrossRef CAS.
- R. Dören, B. Leibauer, M. A. Lange, E. Schechtel, L. Prädel, M. Panthöfer, M. Mondeshki and W. Tremel, Nanoscale, 2021, 13, 8146–8162 RSC.
- H. Liang, H. Xi, S. Liu, X. Zhang and H. Liu, Nanoscale, 2019, 11, 18183–18190 RSC.
- Q. Zhou, Y. Song, N. Li, D. Chen, Q. Xu, H. Li, J. He and J. Lu, ACS Sustainable Chem. Eng., 2020, 8, 7921–7927 CrossRef CAS.
- J. Li, X. Wu, W. Pan, G. Zhang and H. Chen, Angew. Chem., Int. Ed., 2018, 57, 491–495 CrossRef CAS.
- F. Rong, Q. Lu, H. Mai, D. Chen and R. A. Caruso, ACS Appl. Mater. Interfaces, 2021, 13, 21138–21148 CrossRef CAS.
- J. Li, Y. Tian and T. Zheng, Chem. Commun., 2022, 58, 6542–6545 RSC.
- J. Chu, X. Han, Z. Yu, Y. Du, B. Song and P. Xu, ACS Appl. Mater. Interfaces, 2018, 10, 20404–20411 CrossRef CAS.
- M. Wang, J. Chen, Z. Tian, W. Dai, B. Cui, X. Cui, D. Wang, Y. Xiao, X. Lian, C. Jiang, H. Yang, Y. Wang, Z. Sun, Y. Ding, Y.-Y. Sun, J. Zhang and W. Chen, Energy Environ. Sci., 2023, 16, 523–534 RSC.
- H. Cheng, M. Wen, X. Ma, Y. Kuwahara, K. Mori, Y. Dai, B. Huang and H. Yamashita, J. Am. Chem. Soc., 2016, 138, 9316–9324 CrossRef CAS.
- X. Tan, L. Wang, C. Cheng, X. Yan, B. Shen and J. Zhang, Chem. Commun., 2016, 52, 2893–2896 RSC.
- J. Ge, Y. Zhao, X. Gao, H. Li and G. Jie, Anal. Chem., 2019, 91, 14117–14124 CrossRef CAS.
- S. Cong, Y. Yuan, Z. Chen, J. Hou, M. Yang, Y. Su, Y. Zhang, L. Li, Q. Li, F. Geng and Z. Zhao, Nat. Commun., 2015, 6, 7800 CrossRef CAS PubMed.
- Y. Zou, L. Jiang, T. Zhai, T. You, X. Jing, R. Liu, F. Li, W. Zhou and S. Jin, J. Alloys Compd., 2021, 865, 158919 CrossRef CAS.
- J. R. Lombardi, R. L. Birke, T. Lu and J. Xu, J. Chem. Phys., 1986, 84, 4174–4180 CrossRef CAS.
- X. Wang, E. Zhang, H. Shi, Y. Tao and X. Ren, Analyst, 2022, 147, 1257–1272 RSC.
- A. L. Picone, M. L. Rizzato, A. R. Lusi and R. M. Romano, Food Chem., 2022, 373, 131570 CrossRef CAS.
- X. Liu, J. Ma, P. Jiang, J. Shen, R. Wang, Y. Wang and G. Tu, ACS Appl. Mater. Interfaces, 2020, 12, 45332–45341 CrossRef CAS.
|
This journal is © The Royal Society of Chemistry 2024 |
Click here to see how this site uses Cookies. View our privacy policy here.