DOI:
10.1039/D3AY01821K
(Paper)
Anal. Methods, 2024,
16, 122-127
Ultrasensitive fluorescence immunoassay of pepsinogen I based on enzyme-triggered decomposition of AuNCs/MnO2
Received
14th October 2023
, Accepted 28th November 2023
First published on 29th November 2023
Abstract
Gastric cancer is a prevalent malignant tumor of the gastrointestinal tract accompanied by a high mortality rate; therefore, early gastric cancer screening is critical for improving patient survival. In this study, we present a facile fluorescence immunoassay for highly sensitive screening of pepsinogen I (PG I) based on a one-pot biomimetic mineralization process for the synthesis of gold nanocluster-anchored manganese dioxide (AuNCs/MnO2) nanosheets. MnO2 first quenches the fluorescence of AuNCs through the Förster resonance energy transfer effect, whereas the introduction of ascorbic acid (AA) leads to the decomposition of MnO2 and rapidly recovers the fluorescence of AuNCs. Based on the above principles and phenomena, we developed a sensitive fluorescence immunoassay for the in situ generation of AA to detect PG I. Specifically, in the presence of PG I, the sandwich-type immunoreactivity-enriched alkaline phosphatase-labeled secondary antibody catalyzes the production of AA from the substrate, which enhances the fluorescence intensity. Under optimized conditions, the fluorescence intensity increased linearly with the concentration of PG I (0.05 to 200 ng mL−1) with a limit of detection (LOD) of 0.013 ng mL−1 (S/N = 3). The designed sensing platform has good stability (more than one year) and excellent anti-interference capability and demonstrates satisfactory accuracy for detection in real samples compared to commercial ELISA kits.
Introduction
With the increasing pressure of social competition, fast-paced lifestyle, and heavy mental pressure, more and more people suffer from digestive system diseases due to changes in lifestyle and dietary habits. In particular, gastric cancer has become a common malignant tumor of the digestive tract worldwide with a high mortality rate and an increasing incidence year by year.1–3 Gastroscopy has been widely used as the gold standard method for the examination of gastric diseases. However, for some patients, especially the elderly, the examination process is accompanied by severe physical discomfort. Pepsinogen I (PG I) is an inactive precursor of pepsin. Since pepsinogen is secreted by the stomach, it is not affected by short-term diet or other external conditions and enters the bloodstream through the gastric mucosa.4–7 Therefore, the level of PG I in the serum of each individual is relatively stable and accurately reflects the morphological and functional status of the gastric mucosa. Numerous studies have shown that changes in serum pepsinogen concentration are associated with diseases such as Helicobacter pylori infection, peptic ulcer disease, gastritis, and gastric cancer.8 In this regard, the development of a sensitive in vitro PG I diagnostic technique is of great importance for patients with gastric diseases.
To date, technologies based on different kinds of physical/chemical signaling mechanisms in combination with biomolecules (antibodies, nucleic acid aptamers, peptide chains) have been widely reported for various types of chemical/biomolecular assays, such as electrochemical biosensors, photoelectrochemical immunoassays, colorimetric biosensors, and fluorescence biosensors.9–15 Among these methods, fluorescence is arguably the optical method with the longest propagation time and has been routinely used for decades for the detection of various markers due to its high efficiency, low cost, and ease of handling.16–18 Theoretically, fluorescent signals are generated when a substance is subjected to an excitation energy that causes an electron to jump and emit energy. Therefore, fluorescent signals have a high signal-to-noise ratio, and weak signals can be easily detected, realizing the purpose of highly sensitive detection.19–21 However, there are still two key scientific challenges in the pursuit of developing low-cost, sensitive, and simple fluorescence immunoassay strategies. In general, many fluorescent substrates are synthesized either as complex pathway organic molecules or as inorganic materials under harsh reaction conditions (high temperature, hydrothermal) with toxic raw materials. In addition, there is a lack of effective signaling modes combined with immunoassays to construct more efficient fluorescence sensing platforms.
Gold nanoclusters (AuNCs) are typical fluorescent species of fluorescent metal nanoclusters that have been extensively studied.22 The tunable AuNCs have unique optical and electrical properties due to their ability to be sized close to the Fermi wavelength of electrons.23–25 However, in the preparation of fluorescent AuNCs, strong reducing and protective agents are required to prevent condensation. Bovine serum albumin (BSA), a natural biomolecule with high reducing and biocompatible properties, can effectively assist in the synthesis and stabilization of AuNCs.26 In addition, BSA-stabilized AuNCs have other unexpected advantages such as good biocompatibility, low toxicity, high stability, tunable fluorescence size, large stokes drift, and high quantum yield.27–29 Manganese dioxide (MnO2) has attracted much attention in recent years due to its attractive properties, including low toxicity, high specific surface area, and remarkable light-absorbing ability.30–32 Previous work reported that MnO2 can be used as a fluorescence quencher and combined with fluorescent probes for bioanalysis.33 Related work is based on the fact that MnO2 nanosheets can be generated by the redox reaction of KMnO4 and BSA, in which BSA can be used as both a reducing agent and a template.34
Enlightened by the above-mentioned development, we used BSA as a reducing agent and template for the facile green one-step synthesis of AuNC-anchored MnO2 (AuNCs/MnO2) nanosheets, which are sensitive to ascorbic acid (AA). The composites synthesized by the one-step method are relatively homogeneous in morphology with obvious advantages of simple preparation, mild reaction, and low cost. Since MnO2 has a strong light-absorbing ability, it can significantly quench the fluorescence of AuNCs in situ grown on its surface. However, when AA is added to the reaction system, it can react with MnO2 to form Mn2+, leading to the recovery of the quenched fluorescence of AuNCs. We further utilized this phenomenon to construct a facile fluorescence immunoassay for sensitive detection of PG I (Scheme 1). As shown in Scheme 1, after the addition of PG I to complete the sandwich immunoreaction, the alkaline phosphoric acid (ALP)-labeled antibody catalyzes the production of AA from ascorbic acid-2-phosphate (AAP), resulting in a significantly enhanced fluorescence intensity. Therefore, we can quantify the concentration of PG I by monitoring changes in fluorescence intensity.
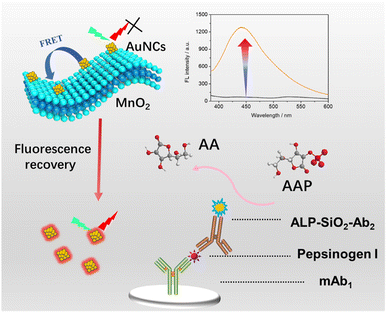 |
| Scheme 1 Schematic illustration of fluorescence immunoassay toward pepsinogen I by coupling alkaline phosphatase (ALP)-catalyzed generation of ascorbic acid (AA)-triggered decomposition of AuNCs/MnO2. | |
Experimental section
Chemicals and reagents
Anti-human PG I monoclonal antibodies, IgG (200 ng mL−1), human serum albumin (HSA, 200 ng mL−1), and PG II and PG I antigens were purchased from Nanjing OK Biotechnology Co., Ltd and Sango Biotech. Co., Ltd. (Shanghai, China). Ascorbic acid 2-phosphate (AAP, CAS no.: 66170-10-3) was supplied by Sigma-Aldrich. Ascorbic acid (C6H8O6, 99.99% metals basis), gold chloride trihydrate (HAuCl4·3H2O, ≥ 99.9% trace metals basis), bovine serum albumin (BSA), manganese nitrate solution (Mn(NO3)2, AR, 50 wt% in H2O), SiO2 nanoparticles (99.5%, 30 nm), aminopropyltriethoxysilane (APTES), and sodium hydroxide (NaOH, 97%) were obtained from Aladdin Reagent Co., Ltd. Deionized water (18.2 MΩ cm) was prepared via a Millipore water purification system (Milli-Q, Germany). Glutaraldehyde (GLD) was purchased from Tianjin Yongda Chemical Reagent Co., Ltd. (Tianjin, China).
Synthesis of gold nanocluster-anchored MnO2 (AuNCs/MnO2)
Pre-configured fresh BSA solution (76 mL, 2.8 mg mL−1) was added to a mixture containing Mn(NO3)2 solution (1.5 mL, 900 mM) and HAuCl4·3H2O solution (1.6 mL, 25 mM), while maintaining vigorous stirring. Subsequently, NaOH solution (0.8 mL, 5.0 M) was introduced into the solution mixture and reacted for 12 h at room temperature to form a homogeneous suspension. When the reaction was completed, the mixture was purified by commercial dialysis (MWCO 14 kDa) for 96 h to remove unreacted excess material. The dialysis reaction was carried out in a 5 L glass vessel, and the deionized water solution should be changed every 12 h. Finally, the resulting AuNCs/MnO2 solutions were stored at 4 °C or vacuum-dried for material characterization.
Preparation of ALP-SiO2-Ab2 conjugates
The synthesis of ALP-SiO2-Ab2 complexes was performed based on the cross-linking of functionalized SiO2 with the anti-PG I antibody and ALP via GLD. SiO2 was first aminated with APTES and then treated with GLD to functionalize SiO2 with aldehyde groups. ALP (50 μg mL−1, 350 μL) and Ab2 (16 μg mL−1, 350 μL) were subsequently injected into an aldehyde-functionalized SiO2 solution (350 μL) and vigorously shaken for 60 min at 37 °C. Following this, the mixture was centrifuged five times to completely remove unbound Ab2 and ALP before being redispersed in 350 μL of PBS solution. The non-specific binding site was blocked with 120 μL of blocking solution at 37 °C for 2 h. After five centrifugations and washes, ALP-SiO2-Ab2 was reconcentrated in 350 μL PBS and stored at 4 °C for additional use.
Fluorescence immunoassay for PG I detection
The PG I antibody (50 μg mL−1, 50 μL) is added to the microtiter plate and allowed to stand at 4 °C overnight (at least >16 h). 200 μL of blocking solution is continuously injected into the wells to block the unspecific sites at 37 °C for 2 h. Different concentrations of PG I standard solution (100 μL) were then injected into the wells and incubated at 37 °C for 1 h to complete the antigen–antibody reaction. After washing, 100 μL of ALP-SiO2-Ab2 was added and incubated for 1 h to complete the sandwich immunoreaction. After three washings, 15 mM AAP was added to the incubation cell and incubated at 37 °C for 40 min. The enzymatic product (AA, 50 μL) was mixed with AuNCs/MnO2 (50 μL) and reacted for 10 min at room temperature to degrade the MnO2 nanosheets. The emission from the resulting suspensions was recorded using a fluorescence spectrophotometer at an excitation wavelength of 470 nm. All measurements were performed in three parallel determinations at room temperature.
Results and discussion
Detailed characterization of AuNCs/MnO2
Proteins that play a key role in biomimetic mineralization for the synthesis of inorganic nanomaterials have a variety of functional groups such as –COOH, –NH2, and –SH. This excellent property makes them great templates for guiding the formation of various inorganic nanostructures. In our synthesis strategy, BSA anchors Mn2+ and [AuCl4]− in solution by virtue of the abundance of carboxyl and thiol groups on its surface to form BSA-Mn/BSA–Au complexes. The nucleation and growth of MnO2 and Au NC were then triggered by adding NaOH to the above solution. To verify the above growth process, we first characterized the synthesized products using high-resolution transmission electron microscopy (HRTEM). Fig. 1A–C show the HRTEM images of AuNCs/MnO2 at different magnifications. Biomimetic mineralization synthesized MnO2 sheets with size scales above the micrometer level, and densely packed AuNCs (3–5 nm) appeared on their surfaces. Notably, no scattering of AuNCs was found in the peripheral blanks, confirming the BSA to be a good anchoring mediator. The elemental valence and composition of the composites were further confirmed using X-ray photoelectron spectroscopy (XPS). The XPS spectra of AuNCs/MnO2 showed the presence of significant peaks of Mn 2p, O 1s, N 1s, C 1s, and Au 4f (Fig. 1D). Two peaks are located at Au 4f7/2 (84.00 eV) and Au 4f5/2 (88.72 eV), representing metallic Au species on the MnO2 surface.35–37 Meanwhile, two characteristic peaks occurred at 653.96 and 642.56 eV, attributed to the Mn 2p3/2 and Mn 2p1/2 spin orbitals of MnO2, respectively.38–40 Notably, the appearance of the N 1s peak correlates with BSA.
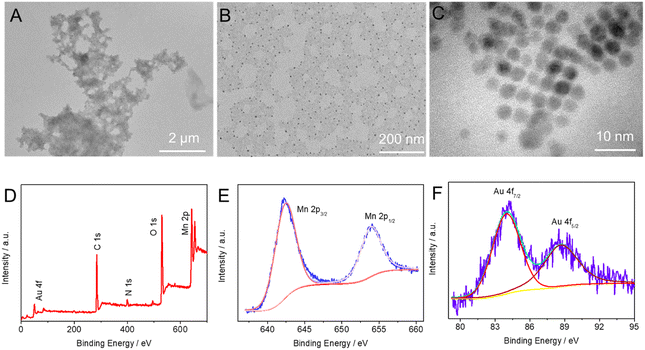 |
| Fig. 1 (A–C) HRTEM images with different magnifications. (D) XPS survey spectra and high-resolution XPS spectra of (E) Mn 2p and (F) Au 4f of AuNCs/MnO2. | |
Feasibility evaluation of the portable PEC immunosensing
As described above, enzyme-catalyzed in situ formation of ascorbic acid triggered by the target induced changes in AuNCs/MnO2 fluorescence intensity. With respect to this point, we next analyzed the change in fluorescence intensity in the presence or absence of the target in the immunoassay system. As shown in curve a of Fig. 2, the solution system in the absence of the target PG I showed no fluorescence signal. When 200 ng mL−1 PG I was introduced into the solution system, a significant enhancement of the fluorescence signal located near 450 nm could be found (curve b in Fig. 2). In addition, the introduction of 200 ng mL−1 PG I caused the solution to change from brown to colorless as can be seen in the corresponding inset. Collectively, these results confirm that the enzymatic reaction induced by the target will degrade MnO2, ultimately causing recovery of the fluorescence signal.
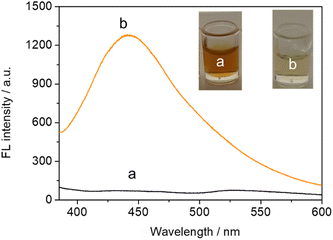 |
| Fig. 2 Fluorescence spectra of AuNCs–MnO2 with (a) 0 ng mL−1 and (b) 200 ng mL−1 PG I immunoassay systems (the inset is a photograph of the corresponding system). | |
Optimization of experimental conditions
To obtain superior analytical performance, we optimized the substrate AAP concentration and the time of the ALP-catalyzed substrate on the antibody in our experiments, using 200 ng mL−1 PG I as a model. As shown in Fig. 3A, the fluorescence intensity showed a good positive correlation with the AAP concentration between 3 and 15 mM, but no longer enhanced significantly beyond 15 mM. To save the cost of the experiment, 15 mM of AAP was chosen as the optimized concentration of the substrate. Similarly, the time of ALP-catalyzed AAP on the antibody was optimized in the region of 10 to 50 min. As shown in Fig. 3B, the fluorescence intensity first increased with the ALP catalytic time up to 40 min and then reached a plateau. Extending the catalytic time did not cause a significant increase in fluorescence intensity. Therefore, 40 min was used as the optimized ALP catalytic time.
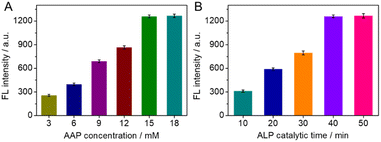 |
| Fig. 3 Effects of (A) AAP concentrations, and (B) catalytic time of ALP toward AAP on the fluorescence intensity of the AuNCs–MnO2. | |
Analytical performance of AuNCs/MnO2-based fluorescence immunoassay
The developed fluorescence immunoassay was used to detect different concentrations of PG I standards (0 ng mL−1–200 ng mL−1) under the above-optimized conditions. As the concentration of PG I increased, the immuno-sandwiching and ALP-catalyzed production of more ascorbic acid degraded the MnO2 nanosheets, which promoted fluorescence enhancement. As shown in Fig. 4A, the recorded fluorescence signals were linearly enhanced with the logarithm of PG I in the range of 0.05 to 200 ng mL−1. The linear regression equation was F (a.u.) = 473.20 + 321.23 × log
CPG I (ng mL−1) (Fig. 4B). The calculated limit of detection (LOD) for PG I was 0.013 ng mL−1 (S/N = 3), which is superior to different types of PG I sensors currently reported (Table 1).
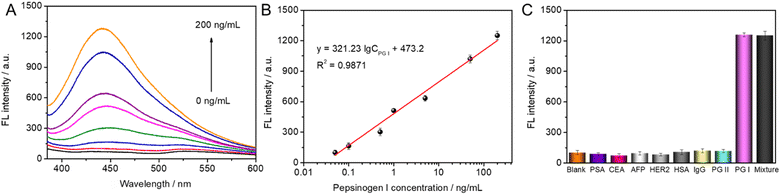 |
| Fig. 4 (A) Fluorescence intensity of the AuNCs–MnO2-based fluorescence immunoassay toward target PG I; (B) calibration plots between fluorescence intensity (a.u.) and the logarithm of PG I (ng mL−1); (C) the anti-interference ability of the AuNCs/MnO2-based fluorescence immunoassay. | |
Table 1 Comparison of different assays for PG I determination
Methods |
Linear range |
LOD |
Ref. |
Janus nanomotor-based lateral flow immunoassay |
5–500 ng mL−1 |
2.2 ng mL−1 |
4
|
Centrifugal microfluidic-based immunoassay |
1–150 ng mL−1 |
0.75 ng mL−1 |
6
|
Point-of-care chemiluminescence immunoassay |
3–200 ng mL−1 |
0.048 ng mL−1 |
41
|
Cu3Pt/MoS2-based electrochemical immunoassay |
0.5–400 ng mL−1 |
0.167 ng mL−1 |
7
|
AuNCs/MnO2-based fluorescence immunoassay |
0.05–200 ng mL−1 |
0.013 ng mL−1 |
This work |
The intra-assay relative standard deviations (RSDs) were 5.63%, 4.96%, and 5.77% (n = 4), and the inter-assay RSDs were 7.69%, 8.12%, and 7.82% (n = 4) with respect to the target PG I of 0.1 ng mL−1, 1.0 ng mL−1, and 200 ng mL−1, respectively, demonstrating ideal reproducibility. In addition, the stability of AuNCs/MnO2 was evaluated over one year. The fluorescence signal of PG I for 1.0 ng mL−1 was maintained by 96.39%, 93.27%, 91.86%, and 90.41% after the 4th, 8th, 9th, and 12th months of storage, respectively. Therefore, the above experimental results indicate that the developed AuNCs/MnO2-based fluorescence immunoassay has favorable reproducibility and prolonged stability.
Since the designed fluorescence immunoassay is employed for the requirements of real sample analysis, the specificity of the assay should be considered. In this case, carcinoembryonic antigen (CEA, 200 ng mL−1), prostate-specific antigen (PSA, 200 ng mL−1), alpha-fetoprotein (AFP, 200 ng mL−1), and human epidermal growth factor receptor 2 (HER2, 200 ng mL−1), IgG (200 ng mL−1), human serum albumin (HSA, 200 ng mL−1), and PG II (200 ng mL−1) were chosen as interfering substances. As can be seen in Fig. 4C, the fluorescence intensity response was higher in the presence of PG I. The fluorescence intensity for the other interfering substances was comparable to that of the blank, indicating that the AuNCs/MnO2-based fluorescence immunoassay has a satisfactory specificity for the detection of PG I.
Analysis of human serum specimens
To estimate the reliability and practicality of the constructed fluorescent immunoassay for the detection of PG I, a series of human serum samples (4 healthy and 1 diseased donor) were analyzed using the developed fluorescent immunoassay as well as a commercially available PG I ELISA kit. The accuracy of the two methods was established by the t-test and the detailed results are summarized in Table 2. The experimental results showed that all calculated experimental values (texp) were lower than 2.78 (tcrit[0.05,4] = 2.78), indicating that the results of the constructed fluorescence immunoassay model showed no significant difference from those of the ELISA kits. Therefore, the proposed fluorescence immunoassay is a feasible method for the detection of PG I in real samples. Additionally, compared to the mature radioimmunoassay (RIA), time resolved fluorescence immunoassay (TRFIA), and ELISA methods, the proposed sensing model exhibits higher sensitivity while maintaining the advantages of contamination-free and moderate cost (Table 3).
Table 2 Comparison of the results obtained from two methods
Sample no. |
Method; concentration: mean ± SD (ng mL−1, n = 3) |
t
exp
|
By fluorescence immunoassay |
By ELISA kit |
1 |
3.66 ± 0.12 |
3.58 ± 0.16 |
0.79 |
2 |
8.79 ± 0.33 |
8.62 ± 0.28 |
0.32 |
3 |
95.69 ± 6.63 |
92.01 ± 5.06 |
1.37 |
4 |
9.69 ± 0.44 |
9.98 ± 0.39 |
1.16 |
5 |
13.46 ± 0.62 |
13.56 ± 0.71 |
1.84 |
Table 3 Mature methods for PG1 detection
Methods |
Safety |
Sensitivity |
Cost |
RIA |
Radiation contamination |
High |
Moderate |
TRFIA |
Pollution-free |
High |
Expensive |
ELISA |
Pollution-free |
Low |
Moderate |
This work |
Pollution-free |
High |
Moderate |
Conclusions
In conclusion, AuNCs/MnO2 was synthesized based on a one-step biomimetic mineralization method, and a split fluorescence immunoassay was successfully constructed for PG I detection. The uniform formation and assembly of AuNCs/MnO2 were well guided using BSA as a template with no strong oxidizing agents, toxic surfactants, and organic solvents. Benefiting from the easy and efficient synthesis, the fluorescence intensity of AuNCs/MnO2 maintained more than 90% of the signal over more than one year of storage. Taking advantage of the amplification effect of the ALP-catalyzed reaction and the outstanding sensitivity of AA to MnO2, the constructed fluorescence immunoassay has excellent sensitivity. In the case of easy biomimetic preparation of nanocomposites, the present platform exhibits several advantages such as ease of use, environmental friendliness, and cost-effectiveness, which suggests that this practical platform is expected to be a candidate for next-generation biomolecular diagnostics.
Ethical statement
All the experiments were performed in accordance with the Guidelines of The Fifth Hospital of Xiamen, and approved by the ethics committee at The Fifth Hospital of Xiamen (China). Informed consent was obtained from human participants of this study.
Author contributions
Huanzong Zhang: conceptualization, methodology, writing – original draft. Binhuang Cai: visualization, investigation, writing – review & editing. Fan Cai: writing – review & editing, project administration. Mingzhe Lian: methodology, funding acquisition, supervision, writing – review & editing, project administration. Yinghui Wang: visualization, investigation, writing – review & editing.
Conflicts of interest
There are no conflicts to declare.
Acknowledgements
This research was funded by the Natural Science Foundation of Fujian Province (grant no. 2021D009).
References
- M. Alsina, V. Arrazubi, M. Diez and J. Tabernero, Nat. Rev. Gastroenterol. Hepatol., 2023, 20, 155–170 CrossRef CAS PubMed.
- K. G. Yeoh and P. Tan, Nat. Rev. Cancer, 2022, 22, 71–84 CrossRef CAS PubMed.
- Y. Cao, Z. Chen, X. Li, Z. Li, G. Lin, T. Liu and Y. Wu, Anal. Chim. Acta, 2022, 1218, 339998 CrossRef CAS PubMed.
- J. Guo, Y. Li, B. Wang, W. Chen, S. Chen, S. Liu, X. Ma and J. Guo, Microchim. Acta, 2022, 189, 468 CrossRef CAS PubMed.
- H. Ohkuma, K. Abe, Y. Kosaka and M. Maeda, Anal. Chim. Acta, 1999, 395, 265–272 CrossRef CAS.
- Y. Shi, J. Guo and J. Guo, Sens. Actuators, B, 2023, 376, 133048 CrossRef CAS.
- S. Wei, S. Li, H. Xiao, F. Zhao, J. Zhu, Z. Chen and L. Cao, Nanoscale Adv., 2023, 5, 133–141 RSC.
- W. Wei, W. Zhang, C. Li, H. Kong, Z. Guo, Z. Zhang, F. Bastien, Y. Gong, H. Wang and L. Zhou, Biosens. Bioelectron., 2019, 129, 231–237 CrossRef CAS PubMed.
- R. Zeng, H. Gong, Y. Li, Y. Li, W. Lin, D. Tang and D. Knopp, Anal. Chem., 2022, 94, 7442–7448 CrossRef CAS PubMed.
- R. Zeng, M. Qiu, Q. Wan, Z. Huang, X. Liu, D. Tang and D. Knopp, Anal. Chem., 2022, 94, 15155–15161 CrossRef CAS PubMed.
- R. Zeng, W. Wang, M. Chen, Q. Wan, C. Wang, D. Knopp and D. Tang, Nano Energy, 2021, 82, 105711 CrossRef CAS.
- Z.-L. Lei and B. Guo, Adv. Sci., 2022, 9, 2102924 CrossRef CAS PubMed.
- R. Zeng, J. Xu, L. Lu, Q. Lin, X. Huang, L. Huang, M. Li and D. Tang, Chem. Commun., 2022, 58, 7562–7565 RSC.
- R. Zeng, J. Xu, T. Liang, M. Li and D. Tang, ACS Sens., 2023, 8, 317–325 CrossRef CAS PubMed.
- Y. Zhao, K. Yavari and J. Liu, TrAC, Trends Anal. Chem., 2022, 146, 116480 CrossRef CAS.
- R. Mohammadi, H. Naderi-Manesh, L. Farzin, Z. Vaezi, N. Ayarri, L. Samandari and M. Shamsipur, J. Pharm. Biomed. Anal., 2022, 212, 114628 CrossRef CAS PubMed.
- C. Guo, A. C. Sedgwick, T. Hirao and J. L. Sessler, Coord. Chem. Rev., 2021, 427, 213560 CrossRef CAS PubMed.
- J. Chen, D. Huang, M. She, Z. Wang, X. Chen, P. Liu, S. Zhang and J. Li, ACS Sens., 2021, 6, 628–640 CrossRef CAS PubMed.
- S. I. Reja, M. Minoshima, Y. Hori and K. Kikuchi, Chem. Sci., 2021, 12, 3437–3447 RSC.
- H. He, C. Wu, M. Saqib and R. Hao, Anal. Bioanal. Chem., 2023, 415, 3655–3669 CrossRef CAS PubMed.
- Y. Hang, J. Boryczka and N. Wu, Chem. Soc. Rev., 2022, 51, 329–375 RSC.
- Y. Zheng, J. Wu, H. Jiang and X. Wang, Coord. Chem. Rev., 2021, 431, 213689 CrossRef CAS.
- R. Zeng, Y. Tang, L. Zhang, Z. Luo and D. Tang, J. Mater. Chem. B, 2018, 6, 8071–8077 RSC.
- Z.-J. Guan, J.-J. Li, F. Hu and Q.-M. Wang, Angew. Chem., Int. Ed., 2022, 61, e202209725 CrossRef CAS PubMed.
- Y. Guo, H. T. N. N. Amunyela, Y. Cheng, Y. Xie, H. Yu, W. Yao, H.-W. Li and H. Qian, Food Chem., 2021, 335, 127657 CrossRef CAS PubMed.
- L. Hu, S. Han, S. Parveen, Y. Yuan, L. Zhang and G. Xu, Biosens. Bioelectron., 2012, 32, 297–299 CrossRef CAS PubMed.
- L. Dong, M. Li, S. Zhang, J. Li, G. Shen, Y. Tu, J. Zhu and J. Tao, Small, 2015, 11, 2571–2581 CrossRef CAS PubMed.
- M. Zhang, Y.-Q. Dang, T.-Y. Liu, H.-W. Li, Y. Wu, Q. Li, K. Wang and B. Zou, J. Phys. Chem. C, 2013, 117, 639–647 CrossRef CAS.
- H.-W. Li, Y. Yue, T.-Y. Liu, D. Li and Y. Wu, J. Phys. Chem. C, 2013, 117, 16159–16165 CrossRef CAS.
- Z. Lin, M. Li, S. Lv, K. Zhang, M. Lu and D. Tang, J. Mater. Chem. B, 2017, 5, 8506–8513 RSC.
- D. Tang, Y. Lin and Q. Zhou, Microchim. Acta, 2018, 185, 476 CrossRef PubMed.
- Y. Lin, Q. Zhou, D. Tang, R. Niessner and D. Knopp, Anal. Chem., 2017, 89, 5637–5645 CrossRef CAS PubMed.
- Y. Du, H. Liu, J. Liang, D. Zheng, J. Li, S. Lan, M. Wu, A. Zheng and X. Liu, Talanta, 2020, 209, 120524 CrossRef CAS PubMed.
- Y. Wang, Y. Song, G. Zhu, D. Zhang and X. Liu, Chin. Chem. Lett., 2018, 29, 1685–1688 CrossRef CAS.
- R. Zeng, Z. Luo, L. Su, L. Zhang, D. Tang, R. Niessner and D. Knopp, Anal. Chem., 2019, 91, 2447–2454 CrossRef CAS PubMed.
- Y. Li, R. Zeng, W. Wang, J. Xu, H. Gong, L. Li, M. Li and D. Tang, ACS Sens., 2022, 7, 1593–1601 CrossRef CAS PubMed.
- R. Zeng, L. Zhang, L. Su, Z. Luo, Q. Zhou and D. Tang, Biosens. Bioelectron., 2019, 133, 100–106 CrossRef CAS PubMed.
- H. Lv, Y. Song, Z. Qin, M. Zhang, D. Yang, Q. Pan, Z. Wang, X. Mu, J. Meng, X. Sun and X.-X. Liu, Chem. Eng. J., 2022, 430, 133064 CrossRef CAS.
- R. Zeng, Y. Li, X. Hu, W. Wang, Y. Li, H. Gong, J. Xu, L. Huang, L. Lu, Y. Zhang, D. Tang and J. Song, Nano Lett., 2023, 23, 6073–6080 CrossRef CAS PubMed.
- R. Zeng, L. Lin, H. Gong, Y. Li, J. Xu, L. Huang, W. Wang, S. Lin, D. Tang and S. Guo, Chem Catal., 2023, 3, 100514 CrossRef CAS.
- F. Liu, J. Zou, X. Luo, Y. Liu, C. Huang, X. He and Y. Wang, Anal. Bioanal. Chem., 2021, 413, 4493–4500 CrossRef CAS PubMed.
Footnote |
† These authors contributed equally to this work. |
|
This journal is © The Royal Society of Chemistry 2024 |
Click here to see how this site uses Cookies. View our privacy policy here.