Solvent-controlled synthesis of hydrophilic and hydrophobic carbon dots†
Received
4th September 2023
, Accepted 25th November 2023
First published on 8th December 2023
Abstract
Hydrophilicity and hydrophobicity are of paramount importance in surface chemistry. In this study, a solvent-controlled synthesis of hydrophilic and hydrophobic carbon dots (CDs) was prepared via a solvothermal process using pentafluorobenzyl alcohol as the carbon source in either deionized water or N,N-dimethylformamide (DMF) medium. By simply varying the reaction solvent to control the doping of nitrogen and fluorine elements, the hydrophilicity or hydrophobicity of the CDs could be regulated. Hydrophobic and hydrophilic CDs showed blue and green light under a UV lamp, respectively. Besides, we regulated the volume ratio of water/DMF (1
:
2, 1
:
1 and 2
:
1) in the reaction solvent to prepare amphiphilic CDs and further studied their hydrophilicity and hydrophobicity. Furthermore, the sensitivity of hydrophobic CDs to water was investigated. In water detection, the photoluminescent intensity of the blue peak and green peak showed high linearity within the water content of 4–80% and 10–80%, respectively (limit of detection = 0.08%, v/v, in DMF).
1. Introduction
Carbon dots (CDs) are nanomaterials with a size of less than 10 nm,1,2 which possess low toxicity, good biocompatibility, and strong light resistance.3 To precisely regulate the luminescence properties of CDs and develop new applications, various methods have been developed to modulate their physical and chemical properties,4–6 such as doping with heteroatoms,7 covalent modifications, and non-covalent modifications. This has enabled the expansion of the application of CDs in sensors,8–10 biological imaging,11,12 photoelectric catalysis,13–15 fluorometric probe,16–18 and optoelectronic devices.19,20
The tuning of hydrophilicity and hydrophobicity of CDs is important in surface manipulation. On one hand, hydrophilicity stabilizes CDs in an aqueous solution and provides a medium for hydrophilic reactions, which is beneficial for applications in the fields of fluorescence probes, biochemistry, diagnostics, and drug delivery.21 On the other hand, hydrophobicity with liposolubility facilitates their transfer into the cell interior, providing better results for bio-imaging, antitumoral drug vehicles, and phototherapeutic agents. What's more, amphiphilic CDs expand their utilities owing to both hydrophilic and hydrophobic characteristics. Nandi et al. fabricated CDs enveloped in an amphiphilic hydrocarbon layer,22 facilitating their application in multicolor microscopic imaging of cellular membranes and live cells. These amphiphilic CDs notably enabled the visualization of membrane disruption induced by the beta-amyloid peptide. Omer et al. prepared amphiphilic CDs as aqueous/organic-based probes for the detection of nitrite and tetracycline, both in aqueous and organic solutions.23
One of the main methods for synthesizing hydrophilic and hydrophobic CDs is the hybridization of CDs and surfactants. Long-chain organic molecules are used as surface passivation/capping agents, such as hexadecylamine,24 octadecylamine,25 and organosilane.26 The amphiphilic structure of surfactants facilitates the affinity to water or lipid of hybrids.27,28 Liu et al. prepared amphiphilic CDs with blue and yellow dual emission, which enhanced the device performance of WLEDs.27 Sarkar et al. prepared hydrophobically modified CDs with different amine terminated small molecules as a surface modifier, and achieved the modulation of the cationic reverse micelle microstructure.29 However, this synthesis method required complex post-treatment and toxic organic solvents, which were unfriendly to the environment. Mao et al. reported another way to synthesize hydrophilic and hydrophobic CDs.30 The approach involved a facile method using BmimPF6 as the sole carbon source in the H3PO4–ethanol medium. H3PO4 provides H+ to partially decompose hydrophobic PF6− into volatile HF and PF5, controlling the hydrophilicity and/or hydrophobicity of the produced CDs.
In this paper, we presented a simple and efficient method to prepare two kinds of fluorine-doped CDs with hydrophilicity and hydrophobicity by one-step hydrothermal synthesis. Pentafluorobenzyl alcohol was used as the carbon source, and water or N,N-dimethylformamide (DMF) was used as the solvent. A simple control of the water/DMF ratio facilitated the regulation of the hydrophilicity and hydrophobicity of the produced CDs. The morphology, microstructure, and composition of the CDs were characterized by X-ray diffraction (XRD), transmission electron microscopy (TEM), X-ray photoelectron spectroscopy (XPS), and Fourier infrared spectroscopy (FTIR). The fluorine-related hydrophobic groups and nitrogen-related hydrophilic groups regulated the hydrophilicity and hydrophobicity of the produced CDs. Additionally, the sensitivity of the CDs to water was tested.
2. Experimental
2.1 Materials
Pentafluorobenzyl alcohol, ethanol, and acetonitrile (AN) were the products of Aladdin Reagents Co. (Shanghai, China). N,N-Dimethylformamide (DMF) was obtained from Sinopharm Chemical Reagent (Shanghai, China). Tetrahydrofuran (THF), formamide, propan-2-ol (IPA), ethyl acetate (EA), ethylene glycol (EG), methanol, and dichloromethane (DCM) were received from Hangzhou Gaojing Fine Chemical Co., Ltd (Hangzhou, China). Dimethyl sulfoxide (DMSO) was obtained from Tianjin Kemio Chemical Reagent Co., Ltd (Tianjin, China). N-Methylpyrrolidone (NMP) was purchased from Senhao Fine Chemical Co., Ltd (Shanghai, China). All the reagents used were at least of analytical reagent grade unless otherwise specified. Deionized water (DI water) of 18 MΩ cm−1 was used throughout.
2.2 Characterizations
UV-vis absorption spectra of the obtained CDs were recorded on a UV-1900i UV-vis spectrophotometer (Shimadzu Corporation, Japan). An RF-6000 spectrofluorophotometer (Shimadzu Corporation, Japan) was employed to record photo-luminescence behaviors. X-ray diffraction (XRD) patterns were recorded on a Rigaku Ultimate IV powder X-ray Cu Ka radiation diffractometer (Rigaku, Japan) with Cu-Kα irradiation (λ = 1.5418 Å, 40 kV and 40 mA) in the range of 2θ from 10 to 80°. Fourier transform infrared spectroscopy (FT-IR) was performed by a Thermo Scientific Nicolet iS20 (Thermo Fisher Scientific, USA). Transmission electron microscopy (TEM) images were recorded on a JEM-2100 high-resolution transmission electron microscopy (JEOL, Japan). XPS analysis was performed on a Thermo Scientific K-Alpha (Thermo Fisher Scientific, USA). Lifetime and quantum yield (QY) assays were conducted by using an FLS980 (Edinburgh Instruments, England).
2.3 Preparation of hydrophilic and hydrophobic CDs
In a typical procedure, 2 g pentafluorobenzyl alcohol was dissolved in 30 mL DI water and then stirred magnetically for 20 minutes at room temperature. The reaction solution was then transferred into a 50 mL stainless steel autoclave lined with Teflon and heated at 160 °C for 6 h in an oven. After the hydrothermal reaction, the autoclave was removed and naturally cooled to room temperature. After centrifugation at 9000 rpm for 30 min, the supernatant was collected followed by insertion into a 500 Da dialysis bag and dialyzed against water for two days, during which the water was changed every 4 hours to remove unreacted reagents and fluorophores. The yellow gelatinous droplets obtained were hydrophobic CDs. The hydrophobic CDs were stored at 4 °C for further use.
The synthesis process of hydrophilic CDs was similar to that of hydrophobic CDs with DMF being used instead of DI water. The obtained CDs could be centrifugated by water and the deposit could be redissolved in DMF. Similarly, amphiphilic CDs were obtained by varying the volume ratio of water and DMF (1
:
2, 1
:
1 and 2
:
1).
3. Results and discussion
3.1 Photoluminescence properties of the CDs
The hydrophobic and hydrophilic CDs were synthesized through a one-step hydrothermal method utilizing pentafluorobenzyl alcohol as the carbon source and either water or DMF as the solvent. As depicted in the optical images (Fig. 1), the hydrophobic CDs exhibit stratification with a yellow lower layer. Conversely, the hydrophilic CDs in DMF appear black under sunlight. Fig. 1 provides a comprehensive representation of the UV-vis absorption and photoluminescence (PL) spectra for the prepared hydrophobic and hydrophilic CDs. The hydrophobic CDs in DMF solution had three absorption peaks at 332, 500, and 539 nm. The hydrophilic CDs were diluted with DI water, and a wide absorption band at around 370 nm was observed. Notably, the baseline rose, which may indicate aggregated CDs. The absorption bands at 332 and 370 nm were attributed to the n–π* transitions of C
O in the carbon structure or C
N lone pairs.31–33 The absorption peaks at 500 and 539 nm were due to the interaction of other functional groups on the surface of the hydrophobic CDs.31
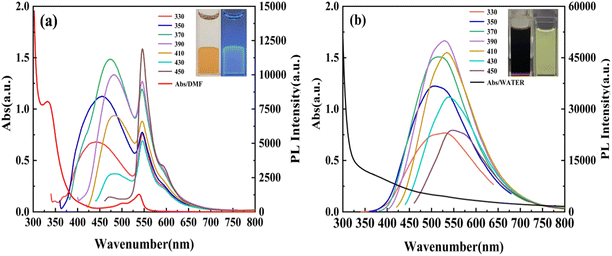 |
| Fig. 1 UV-vis absorption and PL spectra for (a) hydrophobic CDs and (b) hydrophilic CDs. The insets are pictures of hydrophobic and hydrophilic CDs with and without UV lamp irradiation. | |
The PL spectra indicated that hydrophobic CDs exhibited excitation-dependent blue emission and excitation-independent green emission, respectively. And hydrophilic CDs emitted excitation-dependent green light. The PLE spectra in Fig S1 (ESI†) reveal that the hydrophilic and hydrophobic CDs display optimal excitation wavelengths at 366 nm and 385 nm, respectively. The absolute fluorescence quantum yield of hydrophobic and hydrophilic CDs was determined to be 8.18% and 1.2% under 375 nm excitation, respectively. And the related measurement ranges were from 389.5 to 623.0 nm and from 482.0 to 728.0 nm, respectively. Additionally, the lifetime of hydrophobic and hydrophilic CDs was fitted by double-exponential and triple-exponential functions under the same excitation wavelengths, respectively. As shown in Fig. S2 (ESI†), the PL lifetime of the hydrophobic and hydrophilic CDs was tested at 475 nm, 545 nm, and 490 nm under 375 nm excitation, respectively. The calculated average lifetime was 5.0 ± 0.06 ns, 5.0 ± 0.07 ns, and 4.6 ± 0.15 ns, respectively (Table S1, ESI†). These excitation-dependent emissions with multiple pathways may originate from the trap states both in hydrophobic and hydrophilic CDs.33 Notably, the optimal excitation wavelengths and the decay curves for the dual-emission of hydrophobic CDs are almost the same, which may indicate that the two fluorescences are from the same electronic transition processes. For further investigation, the PL of hydrophobic and hydrophilic CDs in different solvents was recorded in Fig. S3 (ESI†). Hydrophobic CDs were found to be undispersed in water and could be effectively dispersed in organic solvents such as ethanol, DMF, AN, EA, DCM, DMSO, THF, EG, IPA, methanol, formamide and NMP. Hydrophilic CDs were dispersed in water, ethanol, NMP, DMF, and THF, undispersed in EG and DMSO, and partially dispersed in EA, DCM, methanol, formamide, IPA and AN. The peak position of hydrophobic and hydrophilic CDs changed with different solvents, ranging from 427 to 481 nm and from 458 to 492 nm, respectively. It is worth noting that hydrophobic CDs exhibit dual-emission in DMF, formamide, DMSO, NMP and AN, and single-emission in other solvents. This phenomenon may be attributed to solvatochromism, which still needs to be further studied in detail.
3.2 Characterization of the structure of CDs
The morphology and size of CDs were characterized by the TEM test. As seen in Fig. 2a, hydrophobic CDs had a quasi-spherical structure with a relatively uniform particle size and a high degree of dispersion. The statistical data indicated that the size of the hydrophobic CDs was 2.84 ± 0.24 nm, distributed from 1 to 5 nm. In Fig. 2b, several hydrophilic CDs aggregated together but dispersed overall. The aggregation phenomena can be confirmed by the rising baseline in the absorption spectrum and will be discussed later. The hydrophobic and hydrophilic CDs both exhibited a lattice structure, with an inter-planar spacing of 0.210 nm, which is attributed to the presence of a (102) crystal face of graphite.34,35
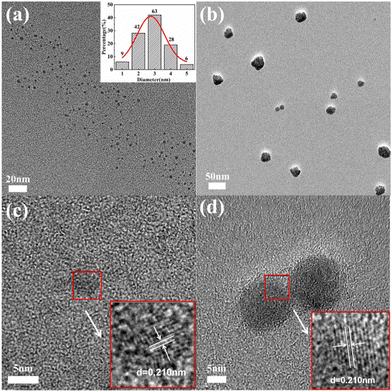 |
| Fig. 2 TEM image of (a) hydrophobic CDs (inset: size distribution) and (b) hydrophilic CDs. HRTEM image of (c) hydrophobic CDs and (d) hydrophilic CDs. | |
The XRD spectra (Fig. S4, ESI†) showed that both the hydrophilic CDs and hydrophobic CDs exhibited broad diffraction peaks, providing evidence for the presence of graphitic carbon (002) crystal planes in the CDs.36 The broad peaks in the range of 20°–30° were typically associated with amorphous carbon-based organic materials.37
FT-IR spectra were used to characterize the chemical structure of hydrophobic and hydrophilic CDs (Fig. 3). Broadbands spanning from 3125 cm−1 to 3710 cm−1 and 2780 cm−1 to 3010 cm−1 were observed, indicating the presence of O–H stretching vibration and N–H stretching vibration, respectively. The peak at 1667 cm−1 corresponded to the stretching vibration of C
O bonds, signifying the presence of carbonyl groups. The peak at 1500 cm−1 confirmed the characteristic vibration of conjugate C
C bonds, typically associated with aromatic rings. The absorption band at 1390 cm−1 was attributed to H–C–H bonds. Peaks at 1306 cm−1 and 1250 cm−1 suggested the presence of C–O and C–N. The absorption peaks around 1050 cm−1 and 1120 cm−1 indicated C–F stretching vibration. The appearance of a peak at 912 cm−1 represented the presence of C
C bending vibration. Lastly, the absorption at 660 cm−1 indicated the presence of N–H wagging vibration.38
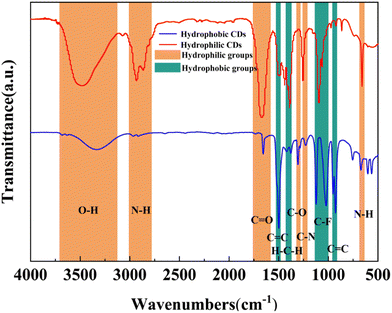 |
| Fig. 3 FT-IR spectra of hydrophobic and hydrophilic CDs. | |
As shown in the FT-IR spectra, the two types of CDs are both amphiphilic CDs. For the sake of facilitating our discussion in this work, they were named hydrophilic CDs and hydrophobic CDs based on their varying water dispersibility. The proportion of hydrophobic and hydrophilic groups affects the water dispersion of CDs. The hydrophilic groups are mainly O–H, N–H, C
O, and C–N and the hydrophobic groups include C–F,39 hydrocarbon groups,40 and aromatic rings.41 As can be seen from the spectra, both kinds of CDs exhibited hydrophobic groups, while the N-related groups were only found in hydrophilic CDs. And the O-related groups in the hydrophilic CDs were much stronger than that in the hydrophobic CDs. It can be deduced that the hydrophilic N- and O-related groups were mainly derived from the DMF solvent as the synthesis environment.42 Compared with hydrophilic CDs, the hydrophobic bands assigned to C–F and aromatic rings became wider in hydrophobic CDs. Fluorine-related groups provided the possibility for the hydrophobicity of the CDs, while the doping of nitrogen element from DMF increased the proportion of hydrophilic groups, thereby adjusting the hydrophilicity and hydrophobicity of the CDs.
In addition, XPS was used to explore the element composition of CDs. It was demonstrated that the binding energy of hydrophobic CDs at 284.1, 532.1, and 687.1 eV was mainly attributed to C1s, O1s, and F1s, respectively (Fig. 4). The composition analysis of hydrophobic CDs showed the elemental composition: C (37.8%), O (22.3%), and F (4.5%). A high-resolution spectrum of C1s indicated the presence of C
C (284.8 eV), C–O (286.0 eV), and C–F (287.4 eV) bonds on the surface of hydrophobic CDs.43,44 The dominant C
C content at 284.8 eV composing the core structure implied that the hydrophobic CDs generally consisted of a carbogenic structure.45 The spectrum of O1s showed three dominant peaks, which were assigned to C–OH (531.1 eV), C–O (532.5 eV), and O
C–O (533.5 eV).38 The F1s high-resolution spectrum of the hydrophobic CDs showed one dominant peak which was assigned to C–F (687.7 eV).44
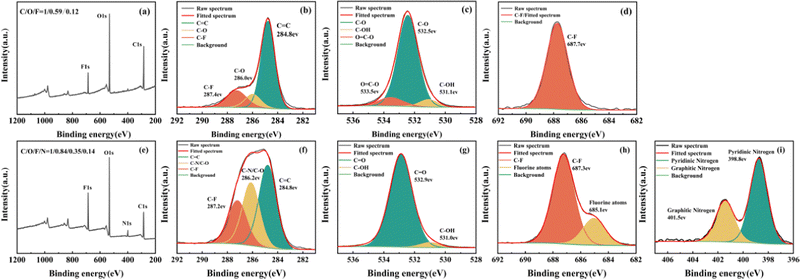 |
| Fig. 4 (a) XPS survey of hydrophobic CDs; (b)–(d) high-resolution XPS spectra of C1s, O1s, and F1s; (e) XPS survey of hydrophilic CDs; (f)–(i) high-resolution XPS spectra of C1s, O1s, F1s, and N1s. | |
The hydrophilic CDs were also investigated by means of XPS. The results demonstrated that the prepared hydrophilic CDs were mainly composed of C, O, N, and F, with binding energies located at 285.1, 533.1, 399.1, and 687.1 eV, respectively (Fig. 4e). The elemental composition analysis of the hydrophilic CDs revealed the following percentages: C (34.8%), O (29.2%), N (4.7%) and F (12.2%), indicating significant doping of F. As shown in Fig. 4f, the C1s spectrum displayed three distinct peaks at 284.8, 286.2, and 287.2 eV, corresponding to C
C, C–N/C–O, and C–F, respectively. Meanwhile, the O1s spectrum exhibited two prominent peaks at 531.0 eV and 532.9 eV originating from C–OH and C
O, respectively (Fig. 4g). The F1s spectrum of hydrophilic CDs could be divided into two peaks with the binding energy of 687.3 eV and 685.1 eV attributed to the C–F and physically trapped fluorine atoms,46 respectively (Fig. 4h). Lastly, the N1s spectrum showed two peaks that were attributed to pyridinic nitrogen (398.8 eV) and graphitic nitrogen (401.5 eV), respectively (Fig. 4i).47
3.3 Amphiphilic regulation of CDs
In order to investigate the impact of varying water and DMF contents in the reaction solvent on the resultant CDs, three types of amphiphilic CDs were synthesized by adjusting the volume ratio of water to DMF (1
:
2, 1
:
1, and 2
:
1). It is noteworthy that the CD solution prepared with a water/DMF ratio of 2
:
1 exhibited stratification, while solutions prepared with ratios of 1
:
1 and 1
:
2 displayed homogeneous dispersion. Under sunlight, the supernatant of the reaction solution with a water/DMF ratio of 2
:
1 appeared transparent, similar to the reaction solution with water/DMF ratios of 1
:
1 and 1
:
2. All of these CDs emitted green light when excited at 365 nm. Besides, the solution in a lower layer, prepared with a water/DMF ratio of 2
:
1, appeared dark brown under sunlight, and its fluorescence was relatively weak. Fig. 5 illustrates the PL spectra and optical images of the CDs obtained by adjusting the volume ratio of water and DMF. These spectra revealed excitation-dependent emission properties, with the emission wavelength redshifted with the increase of the excitation wavelength.
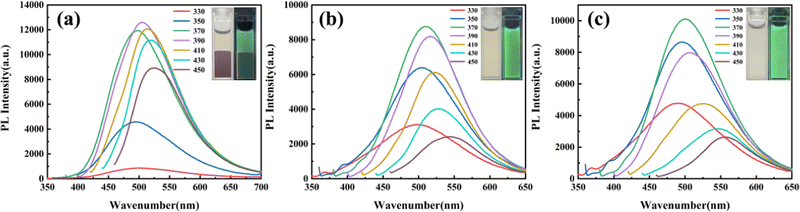 |
| Fig. 5 PL spectra for regulated CDs (a) water/DMF = 2 : 1, (b) water/DMF = 1 : 1, (c) water/DMF = 1 : 2. The insets are pictures of regulated CDs with and without UV lamp irradiation. | |
The amphiphilicity of the produced CDs was controlled by adjusting the water/DMF volume ratio. As shown in the spectra (Fig. S5, ESI†), the transmittance of the O–H, N–H, and C
O bonds increased gradually as the water/DMF volume ratio decreased. This indicated that the hydrophilicity of the prepared CDs increased with the increase of the DMF content. In other words, higher introduced N element resulted in greater hydrophilicity of the CDs.
In addition, the XPS analysis of the CDs was conducted by altering the water/DMF volume ratio (Fig. 6). The element ratio of N/C increased with the increase of the DMF content, further confirming that N related groups are derived from DMF. In addition, the graphitic nitrogen peak in the N1s spectra also became stronger correspondingly, preserving the graphene lattice's symmetry and electron conjugation. Interestingly, the element ratio of F/C also increased with the growing DMF content. The presence of N element may enhance the doping of F atoms in CDs through the cooperative coupling effect between the heteroatoms. Besides, N-doping increases the surface-state defects of CDs and improves the surface chemical reactivity, trapping more fluorine atoms physically.
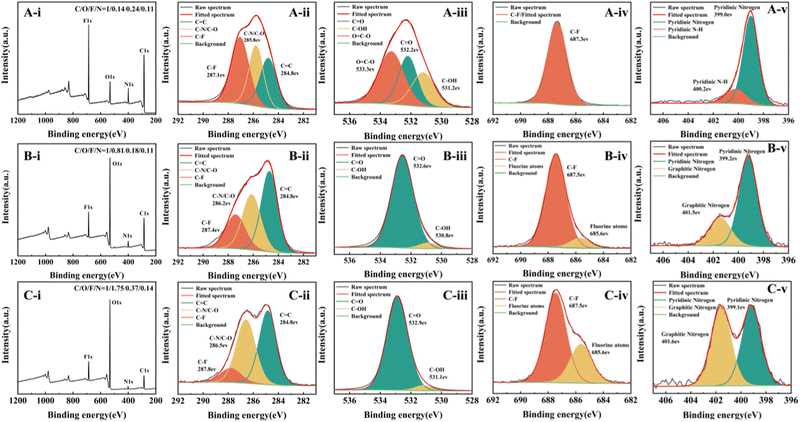 |
| Fig. 6 XPS spectra for the regulated CDs. (A) water/DMF = 2 : 1, (B) water/DMF = 1 : 1, (C) water/DMF = 1 : 2. (i) Survey scan, and high-resolution spectra of (ii) C1s, (iii) O1s, (iv) F1s, and (v) N1s. | |
According to the FT-IR and XPS spectra discussed above, the CDs prepared in pure DMF solvent are amphiphilic with both hydrophilic and hydrophobic groups on the surface. By increasing the volume ratio of water/DMF, the N- and O-related hydrophilic groups reduce. The CDs exhibit enhanced hydrophobicity and tend to aggregate in aqueous solution due to the increasing surface tension (Fig. 7(a)). When the ratio of water/DMF reaches 1
:
1, the increasing surface tension is still not large enough to form aggregates, as shown in the absorption baseline of CDs (Fig. 8(a) and (b)). As the ratio of water/DMF further increases to 2
:
1 or higher, aggregated CDs form an undispersed droplet as the separation phase from the solvent.
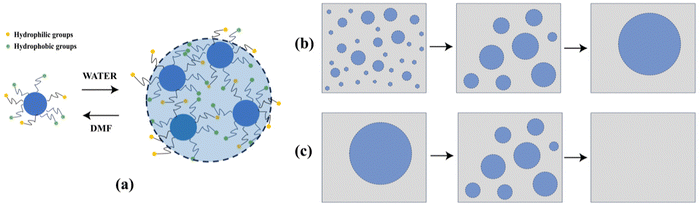 |
| Fig. 7 Schematic diagram of (a) the aggregation and dispersion of CDs in different solvents, (b) the Ostwald ripening process and (c) the shrinking process of the droplets. | |
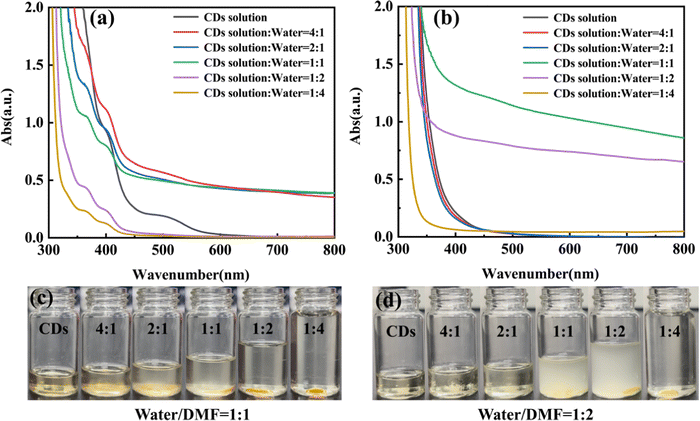 |
| Fig. 8 UV-vis absorption spectra of CDs dispersed with various water contents: (a) water/DMF = 1 : 1, (b) water/DMF = 1 : 2. Images of CDs dispersed with various water contents under sunlight: (c) water/DMF = 1 : 1, (d) water/DMF = 1 : 2. | |
Interestingly, a similar phenomenon occurred when water is further added into the CD solution in the case of 1
:
1 and 1
:
2 (Video S1, ESI†). Taking CDs prepared with water/DMF ratio of 1
:
1 for example, phase separation occurs with an Ostwald ripening process as more water is added.48 Firstly, a large number of small droplets were formed in the solution by adding water. With further addition of water, these small droplets shrunk and large droplets grew, and the mixed solution became turbid. The corresponding baseline of the absorption spectra was greatly raised, which also indicated the aggregation process of CDs. When adding water to the CD solution over a ratio of 1
:
1, a large droplet formed finally at the bottom of the solution (Fig. 7(b)), and the upper layer returned to clear. Accordingly, the absorption spectrum of the supernatant showed a decrease in the baseline. The process was clearly demonstrated by the UV-vis absorption spectra of CDs and the corresponding pictures before and after adding water in Fig. 8. Furthermore, the droplet could be dispersed again in DMF (Fig. 7(c) and Video S2, ESI†). With the decreasing surface tension of the CDs in DMF, the separation phase of the aggregated CDs redissolves back into the solvent, and shrinks until it disappears.
3.4 Water detection by hydrophobic CDs
As a common impurity in organic solvents, water can induce hydrolysis and the formation of irrelevant oxidation substances, which contaminates the solvents during storage.49 Thus, it is necessary to detect the water content in organic solvents during applications.50,51 Quantitative water monitoring via analytical fluorescence sensors is essential both chemically and industrially.52,53
Hydrophobic CDs were selected as fluorescent probes for detecting water content in DMF. The PL intensity of the CDs gradually weakened as the water content increased from 2 to 90% (v/v,%) (Fig. 9a). The change of PL intensity of the CDs (ln(F0/F), where F is the PL intensity of the blue or green peaks and F0 is the maximum PL intensity of the blue or green peaks) towards water content (Q) was analyzed to clarify the fluorescence quenching performance. Notably, the PL intensity of both the blue and green peaks exhibited linear behavior (R2 = 0.994 and 0.998) within the water content range of 4–80% and 10–80%, respectively (Fig. 9b and c). The limit of detection (LOD) in DMF was found to be 0.08%, which is an order of magnitude lower than previously reported values in the literature.54,55
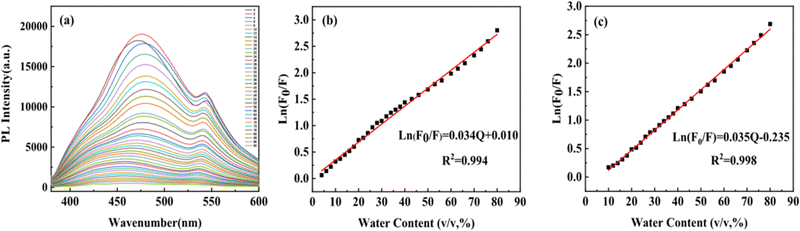 |
| Fig. 9 (a) PL spectra of hydrophobic CDs with different water content in DMF. Effect of water content on the PL intensity of hydrophobic CDs: (b) blue peak and (c) green peak. | |
4. Conclusion
In summary, solvent-controlled hydrophobic and hydrophilic CDs through one-step hydrothermal synthesis in water or DMF were achieved. In an aqueous medium, carbon–fluorine groups facilitated the hydrophobicity of the CDs, while the DMF solvent provided hydrophilic carboxyl and amino groups to offer the hydrophilicity. The amphiphilicity of CDs can be further regulated by varying the water/DMF volume ratio in the reaction system. What's more, hydrophobic CDs were applied in water content detection. This study provided a new approach for the regulation of hydrophilicity/hydrophobicity of CDs, offering great potential for sensing applications.
Author contributions
The manuscript was written through the contributions of all authors. All authors have given approval to the final version of the manuscript. Chenhan Zhang: formal analysis, data curation, writing-original draft, visualization. Zhihua Ying: conceptualization, methodology. Yuan Jiang: project administration, writing-review & editing, validation. Haiyang Wang: investigation. Xuebin Zhou: software. Weipeng Xuan: resources, funding acquisition. Peng Zheng: supervision.
Conflicts of interest
There are no conflicts to declare.
Acknowledgements
The work was supported by Zhejiang Province Key R & D programs No. 2023C01192.
References
- L. Natrayan, D. Veeman and P. P. Patil,
et al., Surface State Treatment of Carbon Dots Using Sulphur Dioxide Isotherm, Adsorpt. Sci. Technol., 2022, 2022, 7387409 Search PubMed.
- J. Gogoi and D. Chowdhury, Calcium-modified carbon dots derived from polyethylene glycol: fluorescence-based detection of Trifluralin herbicide, J. Mater. Sci., 2020, 55(25), 11597–11608 CrossRef CAS.
- F. Huo, W. Li and Y. Liu,
et al., Review of long wavelength luminescent carbon-based nanomaterials: preparation, biomedical application and future challenges, J. Mater. Sci., 2021, 56(4), 2814–2837 CrossRef CAS.
- M. Zhang, X. Zhai and M. Sun,
et al., When rare earth meets carbon nanodots: mechanisms, applications and outlook, Chem. Soc. Rev., 2020, 49(24), 9220–9248 RSC.
- C. Li, B. Lu and X. Wang,
et al., A feasible and universal one-step method for functionalizing carbon dots efficiently via in-situ free radical polymerization, J. Lumin., 2021, 238, 118246 CrossRef CAS.
- T. Luo, Y. Nie and J. Lu,
et al., Iron doped carbon dots based nanohybrids as a tetramodal imaging agent for gene delivery promotion and photothermal-chemodynamic cancer synergistic theranostics, Mater. Des., 2021, 208, 109878 CrossRef CAS.
- S. Miao, K. Liang and J. Zhu,
et al., Hetero-atom-doped carbon dots: Doping strategies, properties and applications, Nano Today, 2020, 33, 100879 CrossRef CAS.
- S. Borna, R. E. Sabzi and S. Pirsa, Synthesis of carbon quantum dots from apple juice and graphite: investigation of fluorescence and structural properties and use as an electrochemical sensor for measuring Letrozole, J. Mater. Sci.: Mater. Electron., 2021, 32(8), 10866–10879 CrossRef CAS.
- X. C. Fu, J. Zhang and W. Gan,
et al., A highly sensitive visible-light photoelectrochemical sensor for pentachlorophenol based on synergistic effect of 2D TiO2 nanosheets and carbon dots, J. Electrochem. Soc., 2020, 167(4), 046513 CrossRef CAS.
- L. J. Mohammed and K. M. Omer, Carbon dots as new generation materials for nanothermometer, Nanoscale Res. Lett., 2020, 15(1), 182 CrossRef PubMed.
- H. Ali, S. Ghosh and N. R. Jana, Fluorescent carbon dots as intracellular imaging probes, Wiley Interdiscip. Rev.: Nanomed. Nanobiotechnol., 2020, 12(4), e1617 CAS.
- S. Paul, A. Bhattacharya and N. Hazra,
et al., Yellow-Emitting Carbon Dots for Selective Fluorescence Imaging of Lipid Droplets in Living Cells, Langmuir, 2022, 38(29), 8829–8836 CrossRef CAS PubMed.
- Y. Ru and S. Lu, Carbonized polymer dots: a novel single-particle organic-inorganic hybrid system, Acta Polym. Sin., 2022, 53(7), 812–827 CAS.
- X. Wang, W. Zhao and H. Lin,
et al., Facet-dependent photocatalytic and photoelectric properties of CQDs/TiO2 composites under visible irradiation, J. Alloys Compd., 2022, 920, 165896 CrossRef CAS.
- H. Sun, G. Yang and B. Yang, Synthesis, Structure Control and Applications of Carbon Dots, Chem. J. Chin., 2021, 42(2), 349–365 CAS.
- K. M. Omer and M. Sartin, Dual-mode colorimetric and fluorometric probe for ferric ion detection using N-doped carbon dots prepared via hydrothermal synthesis followed by microwave irradiation, Opt. Mater., 2019, 94, 330–336 CrossRef CAS.
- K. M. Omer, B. Al-Hashimi and S. Mohammadi,
et al., Carbon nanodots as sensitive and selective nanomaterials in pharmaceutical analysis, J. Mater. Sci., 2022, 57(30), 14217–14245 CrossRef CAS.
- A. H. Alshatteri and K. M. Omer, Smartphone-based fluorescence detection of bilirubin using yellow emissive carbon dots, Anal. Methods, 2022, 14(17), 1730–1738 RSC.
- L. Chen, J. Zheng and Q. Du,
et al., Orange-emissive carbon dot phosphors for warm white light-emitting diodes with high color rendering index, Opt. Mater., 2020, 109, 110346 CrossRef CAS.
- Y. Huang, H. Lin and J. Qiu,
et al., High color rendering indices of white light-emitting diodes based on environmentally friendly carbon and AIZS nanoparticles, J. Mater. Chem. C, 2020, 8(23), 7734–7740 RSC.
- F. Noun, J. Manioudakis and R. Naccache, Toward uniform optical properties of carbon dots, Part. Part. Syst. Charact., 2020, 37(8), 2000119 CrossRef CAS.
- S. Nandi, R. Malishev and K. P. Kootery,
et al., Membrane analysis with amphiphilic carbon dots, Chem. Commun., 2014, 50(71), 10299–10302 RSC.
- K. M. Omer, S. A. Idrees and A. Q. Hassan,
et al., Amphiphilic fluorescent carbon nanodots as a selective nanoprobe for nitrite and tetracycline both in aqueous and organic solutions, New J. Chem., 2020, 44(13), 5120–5126 RSC.
- G. Kubheka, A. O. Adeola and P. B. C. Forbes, Hexadecylamine functionalised graphene quantum dots as suitable nano-adsorbents for phenanthrene removal from aqueous solution, RSC Adv., 2022, 12(37), 23922–23936 RSC.
- Q. Wu, X. Hou and H. Lv,
et al., Synthesis of octadecylamine-derived carbon dots and application in reversed phase/hydrophilic interaction liquid chromatography, J. Chromatogr. A, 2021, 1656, 462548 CrossRef CAS PubMed.
- S. Mura, R. Ludmerczki and L. Stagi,
et al., Integrating sol-gel and carbon dots chemistry for the fabrication of fluorescent hybrid organic-inorganic films, Sci. Rep., 2020, 10(1), 1–12 CrossRef PubMed.
- Y. Liu, D. Sun and Z. Zhang,
et al., Amphiphilic Carbon Dots with Excitation-Independent Double-Emissions, Part. Part. Syst. Charact., 2020, 37(7), 2000146 CrossRef CAS.
- Y. N. Wu, Y. Li and M. J. Cao,
et al., Preparation and stabilization mechanism of carbon dots nanofluids for drag reduction, Pet. Sci., 2020, 17(6), 1717–1725 CrossRef CAS.
- S. Sarkar, K. Das and P. K. Das, Hydrophobically tailored carbon dots toward modulating microstructure of reverse micelle and amplification of lipase catalytic response, Langmuir, 2016, 32(16), 3890–3900 CrossRef CAS PubMed.
- Q. X. Mao, W. J. Wang and X. Hai,
et al., The regulation of hydrophilicity and hydrophobicity of carbon dots via a one-pot approach, J. Mater. Chem. B, 2015, 3(29), 6013–6018 RSC.
- X. Yang, L. Sui and B. Wang,
et al., Red-emitting, self-oxidizing carbon dots for the preparation of white LEDs with super-high color rendering index, Sci. China: Chem., 2021, 64(9), 1547–1553 CrossRef CAS.
- F. Mocci, L. de Villiers Engelbrecht and C. Olla,
et al., Carbon nanodots from an in silico perspective, Chem. Rev., 2022, 122(16), 13709–13799 CrossRef CAS PubMed.
- M. Langer, M. Paloncýová and M. Medveď,
et al., Progress and challenges in understanding of photoluminescence properties of carbon dots based on theoretical computations, Appl. Mater. Today, 2021, 22, 100924 CrossRef.
- L. Tian, D. Ghosh and W. Chen,
et al., Nanosized carbon particles from natural gas soot, Chem. Mater., 2009, 21(13), 2803–2809 CrossRef CAS.
- Y. Hu, J. Yang and L. Jia,
et al., Ethanol in aqueous hydrogen peroxide solution: Hydrothermal synthesis of highly photoluminescent carbon dots as multifunctional nanosensors, Carbon, 2015, 93, 999–1007 CrossRef CAS.
- C. S. Castro, M. C. Guerreiro and L. C. A. Oliveira,
et al., Iron oxide dispersed over activated carbon: support influence on the oxidation of the model molecule methylene blue, Appl. Catal., A, 2009, 367(1–2), 53–58 CrossRef CAS.
- H. Yang, B. Zhou and Y. Zhang,
et al., Valorization of expired passion fruit shell by hydrothermal conversion into carbon quantum dot: physical and optical properties, Waste Biomass Valorization, 2021, 12, 2109–2117 CrossRef CAS.
- L. Jiang, H. Ding and M. Xu,
et al., UV–Vis–NIR full-range responsive carbon dots with large multiphoton absorption cross sections and deep-red fluorescence at nucleoli and in vivo, Small, 2020, 16(19), 2000680 CrossRef CAS PubMed.
- Y. Itoh, S. Chen and R. Hirahara,
et al., Ultrafast water permeation through nanochannels with a densely fluorous interior surface, Science, 2022, 376(6594), 738–743 CrossRef CAS PubMed.
- S. Ghosh, A. Ray and N. Pramanik, Self-assembly of surfactants: An overview on general aspects of amphiphiles, Biophys. Chem., 2020, 265, 106429 CrossRef CAS PubMed.
- J. Liu, G. Shi and P. Guo,
et al., Blockage of water flow in carbon nanotubes by ions due to interactions between cations and aromatic rings, Phys. Rev. Lett., 2015, 115(16), 164502 CrossRef PubMed.
- M. M. Heravi, M. Ghavidel and L. Mohammadkhani, Beyond a solvent: triple roles of dimethylformamide in organic chemistry, RSC Adv., 2018, 8(49), 27832–27862 RSC.
- N. Wang, H. Fan and J. Sun,
et al., Fluorine-doped carbon nitride quantum dots: Ethylene glycol-assisted synthesis, fluorescent properties, and their application for bacterial imaging, Carbon, 2016, 109, 141–148 CrossRef CAS.
- S. Kundu, R. M. Yadav and T. N. Narayanan,
et al., Synthesis of N, F and S co-doped graphene quantum dots, Nanoscale, 2015, 7(27), 11515–11519 RSC.
- H. J. Yoo and B. E. Kwak, Interparticle distance as a key factor for controlling the dual-emission properties of carbon dots, Phys. Chem. Chem. Phys., 2020, 22(36), 20227–20237 RSC.
- S. Ha, C. Lim and Y. S. Lee, Fluorination methods and the properties of fluorinated carbon materials for use as lithium primary battery cathode materials, J. Ind. Eng. Chem., 2022, 111, 111 CrossRef.
- P. Lazar, R. Mach and M. Otyepka, Spectroscopic fingerprints of graphitic, pyrrolic, pyridinic, and chemisorbed nitrogen in N-doped graphene, J. Phys. Chem. C, 2019, 123(16), 10695–10702 CrossRef CAS.
- T. Sakai, K. Kamogawa and K. Nishiyama,
et al., Molecular diffusion of oil/water emulsions in surfactant-free conditions, Langmuir, 2002, 18(6), 1985–1990 CrossRef CAS.
- L. Xu, N. Yao and H. Ding,
et al., Facile Synthesis of Carbon Dots from Biomass Material and Multi-Purpose Applications, J. Fluoresc., 2022, 32(2), 783–789 CrossRef CAS PubMed.
- Y. Zhang, C. Liang and S. Jiang, A solvatochromic cyanostilbene derivative as an intensity and wavelength-based fluorescent sensor for water in organic solvents, New J. Chem., 2017, 41(16), 8644–8649 RSC.
- N. I. Georgiev, P. V. Krasteva and V. B. Bojinov, A ratiometric 4-amido-1, 8-naphthalimide fluorescent probe based on excimer-monomer emission for determination of pH and water content in organic solvents, J. Lumin., 2019, 212, 271–278 CrossRef CAS.
- H. Song, Y. Zhou and C. Xu,
et al., A dual-function fluorescent probe: sensitive detection of water content in commercial products and rapid detection of hypochlorite with a large Stokes shift, Dyes Pigm., 2019, 162, 160–167 CrossRef CAS.
- K. P. Wang, Y. Lei and J. P. Chen,
et al., The coumarin conjugate: synthesis, photophysical properties and the ratiometric fluorescence response to water content of organic solvent, Dyes Pigm., 2018, 151, 233–237 CrossRef CAS.
- D. Chao, W. Lyu and Y. Liu,
et al., Solvent-dependent carbon dots and their applications in the detection of water in organic solvents, J. Mater. Chem. C, 2018, 6(28), 7527–7532 RSC.
- Y. Qin, Y. Bai and P. Huang,
et al., Dual-emission carbon dots for ratiometric fluorescent water sensing, relative humidity sensing, and anticounterfeiting applications, ACS Appl. Nano Mater., 2021, 4(10), 10674–10681 CrossRef CAS.
|
This journal is © the Owner Societies 2024 |
Click here to see how this site uses Cookies. View our privacy policy here.