DOI:
10.1039/D4MA00077C
(Paper)
Mater. Adv., 2024,
5, 5543-5553
Observation of cluster spin glass behavior in thermally decomposed nanocrystalline Sn0.5Fe0.5O2−δ†
Received
26th January 2024
, Accepted 16th May 2024
First published on 16th May 2024
Abstract
We report the structural and magnetic properties of nanocrystalline Sn0.5Fe0.5O2−δ prepared by thermal decomposition of hexameric organotin ferrocenecarboxylate. The X-ray diffraction study revealed that Fe doping retains the tetragonal rutile structure of SnO2. FTIR shows the presence of O–Sn–O stretching vibration. Microstructural investigation by FESEM and HRTEM indicated nanoparticle agglomeration and different orientations of lattice fringes with an interplanar spacing of 0.332 nm. The XPS analysis unveiled the incorporation of Fe3+ ions into the SnO2 matrix. Mössbauer spectroscopy suggested the presence of Fe3+ ions in a distorted octahedral environment. Bulk magnetization and ac susceptibility studies assert the presence of competing ferromagnetic and antiferromagnetic interactions and a cluster spin glass state (Tg = 72 K) at low temperatures.
Introduction
Dilute magnetic semiconductors (DMS) possessing good semiconducting and magnetic properties at room temperature have attracted intense interest due to their potential practical applications.1–3 However, the origin of magnetic interactions in DMS is ambiguous.4–8 Most models are based on the interactions among magnetic impurities mediated via free carriers or oxygen ions. Defects as well as non-magnetic dopant induced ferromagnetism have also been reported.7,8 DMS are created by partially incorporating transition metal atoms such as V, Cr, Mn, Fe, Co, Ni and so on into the semiconductors. Among oxide semiconductors, tin oxide (SnO2), a wide band gap semiconductor owing to its high transparency, chemical stability and electrical conductivity with interesting applications in solar cells, catalysis and gas sensing, is widely explored as a promising candidate in DMS research. Therefore, room temperature ferromagnetism in transition metal-doped SnO2 was investigated by many groups.9–26 In particular, partially Fe doped Fe–SnO2 has displayed large magnetic moments24 resulting in magnetic properties suitable for spintronic applications. Both p- and n-type behaviour has been observed in Fe–SnO2 which depends on the Fe content and operating temperature.27 More specifically, in Fe-doped SnO2 systems, the addition of iron induces changes in SnO2 properties, such as the band gap value, optical transmittance, grain size, grain boundary properties, electrical and magnetic behaviors, etc. Furthermore, apart from the interesting magnetic properties, Fe-doped SnO2 was used to detect ethanol,27 carbon monoxide,28,29 hydrogen sulphide,30 methane,31 acetone32 and humidity33 and degrade rhodamine B in aqueous solutions.34
The physical properties of Fe–SnO2 can be tailored by altering its structure, composition and particle/grain size and it is sensitive to growth conditions. Various synthesis methods were employed to prepare Fe–SnO2 samples in bulk, nanocrystalline and thin film forms.13,15,19,24,27–50 First principles calculations suggested that the ferromagnetic state is energetically favourable compared to the antiferromagnetic state in Fe doped SnO2.10 Bulk Fe–SnO2 exhibited room temperature ferromagnetism.16 Nanocrystalline Sn1−xFexO2 prepared by a cost-effective sol–gel route35–38 also exhibited room temperature ferromagnetism. The maximum iron doping accomplished is 20% but the magnetic nature is unknown.38 A polymeric modified Pechini process39 with Sn(II)/Fe(II) and Sn(IV)/Fe(III) as starting reactants showed that Sn(IV)/Fe(III) was effective to get pure nanocrystalline Sn1−xFexO2 (x = 0.1). A one-step co-precipitation method40–42 was successfully employed to get an x = 0.15 sample wherein the system displayed a combination of paramagnetism and weak ferromagnetism. A similar inference was obtained in the nanocrystalline Sn1−xFexO2−δ (x = 0.1) sample prepared using the high-energy ball milling method.43 Nanocrystalline samples prepared using the hydrothermal method34,44,45 also exhibited ferromagnetic behaviour. Room temperature ferromagnetism (Tc ∼ 610 K) in Sn0.95Fe0.05O2 thin films47 prepared using the pulsed laser deposition (PLD) method was explained using the ferromagnetic coupling of ferric ions via an electron trapped in oxygen vacancy (F-center exchange mechanism).5 The magnetic moment reduces drastically as x approaches a percolation threshold of 0.25.5 Other studies on Fe–SnO2 thin films prepared by PLD also reported the role of oxygen vacancies near Fe atoms48 or the importance of the double exchange mechanism26 in Sn1−xFexO2 (x ≤ 0.4) in establishing the ferromagnetism in the system. In contrast, nanocrystalline Sn0.8Fe0.2O2 films had a Fe2O3 impurity phase that displayed excitonic features due to 2p–3d hybridization.49 The changes in electronic structure and magnetism of Fe–SnO2 thin films grown by radio frequency magnetron sputtering50,51 were attributed to bound magnetic polarons. Sn1−xFexO2 thin films deposited by the spray pyrolysis method52 exhibited secondary phases like β-Fe2O3 and β-Fe3Sn as x changes from 0.078 to 0.396.
The increase in iron doping creates more oxygen vacancies in the SnO2 structure. It stimulates the possibility of displaying an interesting magnetic behavior. To date, most of the studies on the Sn1−xFexO2 system were concentrated around x ≤ 0.2 in the bulk wherein the occurrence of ferromagnetic behaviour was ascribed to impurity band exchange or competing double and super exchange interactions or secondary phase or isolated magnetic impurity (ferric ion) interactions. A large x (∼0.4) was attempted in thin films and ferromagnetic behaviour was retained26 unlike the spin glass nature predicted by the donor-impurity model at such high doping. More so, neither higher Fe doping in nanocrystalline Fe–SnO2 was attempted nor the nature of magnetism and underlying magnetic interactions were investigated. To the best of our knowledge, no report on x ≈ 0.5 exists for this compound. To this end, using the thermal decomposition of the hexameric organotin drum [nBuSn(O)O2CFc]6 (Fc = ferrocenyl), we prepared nanocrystalline Fe doped SnO2 wherein Fe and Sn are in equal proportions. In the present work, we report the structural, microstructural, X-ray photoelectron spectroscopy, Mössbauer spectroscopy, bulk magnetization and ac susceptibility studies on the nanocrystalline Sn0.5Fe0.5O2−δ system.
Experimental details
Synthesis of the Sn0.5Fe0.5O2−δmaterial
We chose the already reported, organostannoxane-supported hexaferrocene carboxylate, [nBuSn(O)O2CFc]6 (Fc = C5H5FeC5H4, ferrocenyl), 1 as the starting precursor for this work.53 Because the Sn
:
Fe ratio is 1
:
1, achieving 50% Fe-doped SnO2 is possible. Recently, we have successfully prepared tin(IV) oxide from the thermal decomposition of the hexameric organotin drum [nBuSn(O)O2CR]6 (R = 9-xanthenyl) by heating it to a temperature range of 600–900 °C.54 This method has been adopted to prepare 50% Fe-doped SnO2. Another reason for choosing 1 is that the presence of this molecule can be simply confirmed by the NMR (1H and 119Sn) technique, and also the molecular structure is already established. The interesting aspect of 1 is that it comprises six ferrocenecarboxylate moieties attached to a central stannoxane Sn6O6 core that appears to be a drum-like structure. Thermogravimetric analysis (TGA) performed provides information about the thermal stability of the obtained compound. The thermogram shows that the drum compound is stable up to 300 °C (Fig. S1, ESI†). Above this temperature, 50% decomposition occurs and after ∼600 °C, the compound shows stable behavior. Based on these results, the drum compound was heated at 600 °C for 2 h using a muffle furnace (Scheme 1). An orange solid obtained after thermal decomposition was crushed and powdered for further analysis.
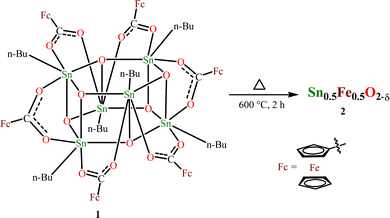 |
| Scheme 1 Synthesis of 50% Fe doped SnO2. | |
Material characterization
FT-IR spectra were recorded on a PerkinElmer instrument in the range 4000–400 cm−1 using KBr pellets. The FT-IR technique was used to get information about metal–oxygen stretch. The FT-IR spectrum band at 593 cm−1 can be related to the stretching mode vibration55 of O–Sn–O/Sn–O–Sn/Sn–O (Fig. S2, ESI†). Thermal studies for compound 1 were carried out using an STA 6000 (PerkinElmer) instrument under a nitrogen atmosphere (20 mL min−1) for 5.982 mg of sample in the temperature range of 30–700 °C. Furthermore, structural analysis with X-ray diffraction measurements was conducted in the reflection mode on an Empyrean PANalytical diffractometer (45 kV, 30 mA with Cu-Kα radiation with λ = 0.15406 nm). X-ray diffraction data were collected at a scanning rate of 0.0313° per second in the 2θ range from 10° to 80°. To record the X-ray diffraction (XRD) pattern, 10 mg of crushed sample was taken on zero-background silicon. The morphology of the material was examined by FE-SEM, and EDX analysis was performed using a Carl Zeiss (Ultra 55) instrument. The sample was carbon coated for FE-SEM analysis. HRTEM studies were carried out using a Tecnai G2 20 S-TWIN [FEI] instrument operated at an accelerating voltage of 200 keV. The sample was drop cast on a Cu grid for HR-TEM analysis. Oxidation states and chemical environment of elements were determined by XPS studies performed on a Kratos Axis Ultra instrument using monochromatic Mg Kα radiation. The Mössbauer spectrum of the material was recorded using a Mössbauer spectrometer operated in constant acceleration mode (triangular wave) in transmission geometry at room temperature. The source employed was 57Co in the Rh matrix of strength 2 mCi. Calibration of the velocity scale was done by using an enriched α-57Fe metal foil. The line width (inner) of calibration spectra was 0.26 mm s−1. The isomer shift (δ) values are relative to the Fe metal foil (δ = 0.0 mm s−1). Temperature and field dependent dc magnetic measurements were performed using a physical property measurement system (PPMS) (Dynacool, Quantum Design) with a vibrating sample magnetometer option (VSM). AC susceptibility measurements as a function of temperature at different frequencies were carried out using an ACMS (AC measurement system) option attached to the same PPMS setup.
Results and discussion
Fig. 1(a) shows the Rietveld refinement of room temperature powder XRD pattern of Sn0.5Fe0.5O2−δ in the 2θ range 10°–80°. From the Rietveld refinement, it is confirmed that the sample crystallizes in a tetragonal structure (space group P42/mnm) with the lattice constants a = b = 4.734 Å and c = 3.173 Å and has a minor secondary phase of Fe3−xSnxO4. A quantitative phase analysis through the Rietveld refinement estimated the amount of the impurity phase (Fe3−xSnxO4) to be less than 2% and the impurity peak is marked with (*) in Fig. 1(a).
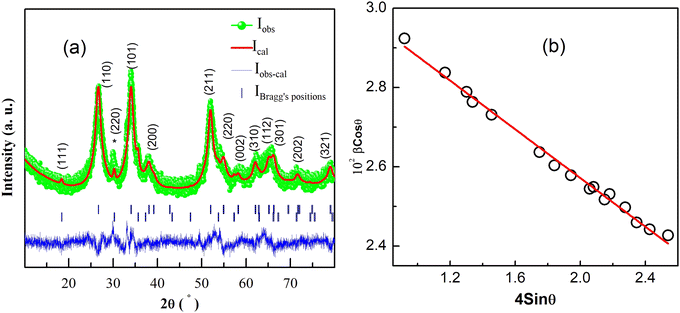 |
| Fig. 1 (a) Rietveld refinement of room temperature XRD pattern for the Sn0.5Fe0.5O2−δ. (220) plane of Fe3−xSnxO4 is indicated by (*). (b) Williamson–Hall plot. | |
The average crystallite size (d) and lattice strain (ε) were estimated by employing the Williamson–Hall (WH) plot based on the equation βFWHM Cos
θ = kλ/d + 4ε Sin
θ, where θ is Bragg's angle, βFWHM is the full width at half-maximum corresponding to Bragg's peak, k is a constant equal to 0.89 and λ is the wavelength of Cu-Kα X-ray radiation (λ = 0.154 nm). The W–H plot for Sn0.5Fe0.5O2−δ is shown in Fig. 1(b) which gives an average crystallite size (d) of 4.30 nm and a compressive micro-strain (lattice strain ε) of 3 × 10−3.
The crystal structure (Fig. 2) was constructed using the crystallographic information file (.cif) obtained by Rietveld refinement in 3D view with the help of VESTA software. It reveals that Sn/Fe atoms occupy the body center and corner positions of the unit cell. Every Sn/Fe atom is bonded with six oxygen atoms at octahedral positions and forms an octahedron as shown in Fig. 2b. Every unit cell consists of nine Sn/FeO6 octahedra, out of which one is inside, and the remaining eight are at the corners of the unit cell.
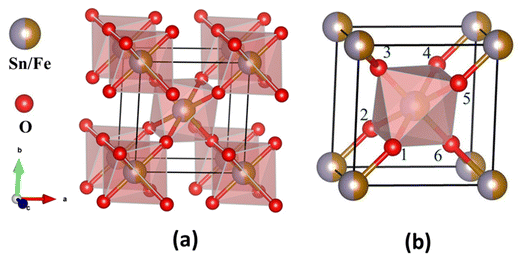 |
| Fig. 2 Structural model of Sn0.5Fe0.5O2−δ. (a) Octahedra present in the tetragonal lattice and (b) octahedron formed by coordination of one Sn/Fe atom and six oxygen atoms. | |
Table 1 reports the structural parameters obtained from the Rietveld analysis of X-ray diffraction data collected at room temperature. In the refinement, cation occupancies were determined to be the same as the nominal values. The negligible iron oxide impurity phase in the XRD pattern implies that the magnetism and the underlying magnetic interactions are related to the properties of the host system and its oxygen off-stoichiometry, if any.
Table 1 Structural parameters and agreement indices obtained by Rietveld refinement of the room temperature X-ray powder diffraction pattern for Sn0.5Fe0.5O2−δ
Parameter |
Result |
Space group |
P42/mnm |
Space group number |
136 |
Crystal system |
Tetragonal |
No. formula units per unit cell |
2 |
Cell parameters |
a = b (Å) |
4.734 |
c (Å) |
3.173 |
Volume (Å3) |
71.10 |
Density (g cm−3) |
5.570 |
Atom coordinates |
Sn/Fe (x, y, z) |
0.0000, 0.0000, 0.0000 |
O (x, y, z) |
0.2721, 0.2721, 0.0000 |
Occupancy |
Sn |
0.50 |
Fe |
0.49 |
O |
1.78 |
Bond distance (Fig. 2b) |
Sn–O(1) (Å) |
2.20 |
Sn–O(2) (Å) |
2.20 |
Sn–O(3) (Å) |
1.82 |
Sn–O(4) (Å) |
2.20 |
Sn–O(5) (Å) |
2.20 |
Sn–O(6) (Å) |
1.82 |
Bond angles |
O(1)–Sn–O(2) (°) |
92.24 |
O(1)–Sn–O(3) (°) |
90.00 |
O(1)–Sn–O(4) (°) |
180.00 |
O(1)–Sn–O(5) (°) |
87.76 |
O(1)–Sn–O(6) (°) |
90.00 |
χ
2
|
1.7 |
R
p
|
19.7 |
R
wp
|
19.0 |
R
e
|
14.2 |
FE-SEM image was recorded to gain knowledge about the surface morphology of the material (Fig. 3a). Film-like morphology of the prepared material can be observed from the FE-SEM images. The TEM image shows the presence of agglomerated circular particles having an average diameter of ∼8.5 nm (Fig. 3b). A concentric ring pattern in the selected area electron diffraction (SAED) image indicates the polycrystalline nature of the material (Fig. 3c). Lattice fringes present in the HR-TEM image (Fig. 3d) show an interplanar spacing of 0.332 nm which corresponds to the (110) plane57 of SnO2. Lattice fringes are rotated in different directions to show the random orientation of particles. The chemical composition revealed by EDX spectra yields Fe/(Fe + Sn) ≅ 0.5.
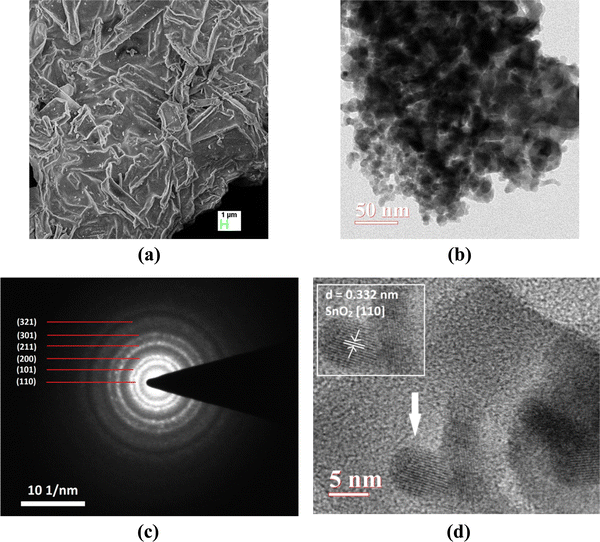 |
| Fig. 3 (a) FE-SEM image, (b) TEM image, (c) SAED pattern, and (d) HR-TEM image of the synthesized material (the inset shows the calculated interplanar spacing). | |
X-ray photoelectron spectroscopy (XPS)
XPS spectra of the sample were recorded to know the oxidation states of elements. A survey scan of the sample shows the presence of mainly carbon C(1s), oxygen O(1s), tin Sn(3d) and iron Fe(2p) peaks (Fig. 4a). A C–C bond peak was observed at 284.6 eV which is considered a reference peak (Fig. S4, ESI†). The peak at 529.4 eV in O(1s) is due to the presence of the Fe–O bond58 and the peak at 532.9 eV may occur due to H2O adsorbed59 on the Fe doped SnO2 surface (Fig. 4b). In the Sn(3d) spectrum, a doublet with peaks at 489.1 and 497.6 eV for (3d5/2) and (3d3/2) with a peak separation (spin–orbit splitting) of ∼8.5 eV respectively suggests the presence60 of the Sn4+ state of Fe/SnO2. Another less intense deconvoluted doublet occurred at 485.8 eV and 494.1 eV assigned56,61,62 to (3d5/2) and (3d3/2) respectively, indicating the presence of the Sn4+ oxidation state in different chemical environments (Fig. 4c). Other than these peaks, a single peak at 716.3 eV also occurred in the spectrum which belongs to Sn(3p3/2)63,64 of the Sn4+ state. In the Fe(2p) spectrum, the presence of Fe3+ was confirmed due to the presence of peaks65 at 713.5 eV and 726.6 eV due to (2p3/2) and (2p1/2) lines respectively, with a separation (2p1/2–2p3/2) of 13.1 eV. A satellite peak66 for Fe3+ was also observed at 719.5 eV which appears highly intense due to association with the Sn 3p3/2 peak (Fig. 4d). A broad peak around 58.5–60 eV was assigned to the Fe3+ (3p) state (Fig. S5, ESI†). Additionally, a shoulder-type peak is also observed at about 53–56 eV. However, the absence of a doublet corresponding to Fe 2p in 709–710 eV67 (Fig. 4) confirms no Fe2+ or Fe3O4 impurity. The oxygen deficiency content using the standard protocol turns out to be δ = 0.18 which is slightly less than δ = 0.25 expected from the charge neutrality of the nominal composition Sn0.5Fe0.5O2−δ.
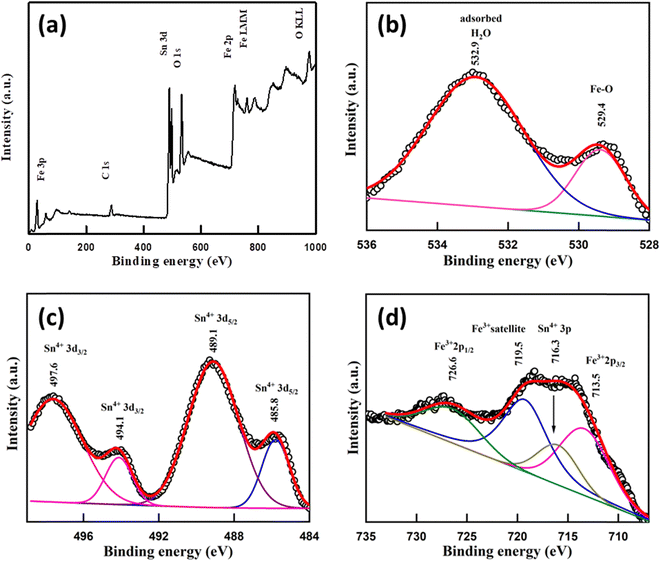 |
| Fig. 4 XPS spectra: (a) survey scan, (b) O 1s, (c) Sn 3d and (d) Fe 2p. | |
Mössbauer spectroscopy
Knowledge of the local environment around the dopant atom is necessary to understand the underlying mechanism leading to magnetic order in the system. Therefore, a Mössbauer spectroscopy study was done to ascertain the oxidation state and local environment of Fe. The room temperature Mössbauer spectrum of Sn0.5Fe0.5O2−δ shown in Fig. 5 exhibits a well-defined doublet indicating that the incorporated Fe-ions are paramagnetic and in the 3+ oxidation state in an octahedral environment. The presence of Fe2+ ions was not observed in the system. The Mössbauer spectrum measured even at a higher velocity (±11.5 mm s−1) shows no sextet (six lines Zeeman splitting pattern) related to magnetically ordered Fe3+ ions but a doublet. The values of spectral parameters such as δIS – the isomer shift (relative to Fe metal foil), ΔEQ – the quadrupole splitting, Γ – the full width at half maximum, RA – the relative spectral area and the co-ordination number of the Fe ion are provided in Table 2. Larger electric quadrupole splitting suggests a different Fe environment and more distorted sites in the rutile structure. This is the case of the Fe3+ ions in the octahedral cage of oxygen ions with relatively large concentrations of oxygen vacancies leading to a distorted environment. The data quality of the Mössbauer spectrum is poor due to the very old (low strength) Mössbauer source used for the experiment and the nano-nature of the sample. Therefore, the impurity phase of Fe3−xSnxO4 (∼2%) is not visible, which should show two sextets (tetrahedral and octahedral sites) with broader line widths in the Mössbauer spectrum.
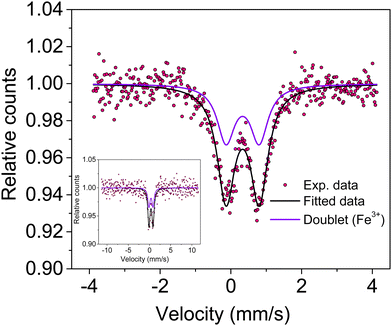 |
| Fig. 5 Mössbauer spectrum of Sn0.5Fe0.5O2−δ showing a doublet due to Fe3+ ions present in the octahedral site. The inset shows the full-scale Mössbauer spectrum. | |
Table 2 Mössbauer spectral parameters of the Sn0.5Fe0.5O2−δ sample at room temperature
Sample code |
δ
IS (mm s−1) |
ΔEQ (mm s−1) |
Γ (mm s−1) |
R
A (%) |
Fe-site (doublet) |
Fitting quality (χ2) |
Sn0.5Fe0.5O2−δ |
0.332 ± 0.006 |
0.938 ± 0.01 |
0.603 ± 0.016 |
100 |
Doublet-Fe3+(VI) |
1.16 |
Magnetic properties
Fig. 6 shows the thermal evolution of magnetization in the temperature range of 2 K to 390 K measured in zero-field cooled (ZFC) and field-cooled (FC) modes. It can be seen that magnetization does not attain zero value even at 390 K suggesting the presence of magnetic order much above 390 K. It is interesting to see that FC magnetization at 2 K is 3 times the magnetization at 390 K. Magnetizations in ZFC and FC modes overlap with each other above a certain value of temperature, the so-called irreversibility temperature (Tir ∼ 252 K) while deviating from each other below Tir. This irreversibility suggests the presence of magnetic anisotropy in the system. Irreversibility in magnetization is observed in ferromagnets, spin-glass (SG), and superparamagnetic (SPM) systems. ZFC magnetization (MZFC) exhibits a peak at Tp = 72 K and decreases continuously with temperature. This type of feature in MZFC is generally seen in SG and SPM systems. On the other hand, FC magnetization (MFC) increases continuously below Tp suggesting the presence of antiferromagnetic interactions at low temperatures.
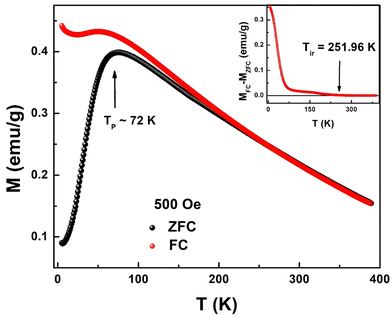 |
| Fig. 6 Field-cooled (FC) and zero-field-cooled (ZFC) temperature dependent magnetizations at 500 Oe for Sn0.5Fe0.5O2−δ. Inset: MFC–MZFC as a function of temperature. | |
In Fig. 7, M(H) hysteresis loops of the Sn0.5Fe0.5O2−δ sample measured at 10 K, 50 K, and 100 K are shown. The inset shows the magnified view of MH data around the zero field. Finite coercivity (Hc) at these temperatures reflects the ferromagnetic (FM) character of the Sn0.5Fe0.5O2−δ system. However, non-saturating MH loops at a magnetic field as high as 80 kOe suggest the presence of antiferromagnetic (AFM) correlations in the system. As the temperature increases from 10 K to 50 K, both remanent magnetization (Mr) and Hc values reduced drastically from 0.56 emu g−1 to 0.1 emu g−1 and 1500 Oe to 82 Oe, which eventually reach values of 0.02 emu g−1 and 22 Oe, respectively at 100 K. This is in contrast to nanocrystalline Sn0.95Fe0.05O2 whose Hc increased with temperature11 which was attributed to freezing of superparamagnetic domains embedded in the antiferromagnetic matrix at low temperatures. Therefore, it can be inferred that both FM and AFM interactions are present in Sn0.5Fe0.5O2−δ. The saturation magnetization at 10 K in H = 80 kOe reduces from 0.21 μB/Fe to 0.05 μB/Fe at 100 K.
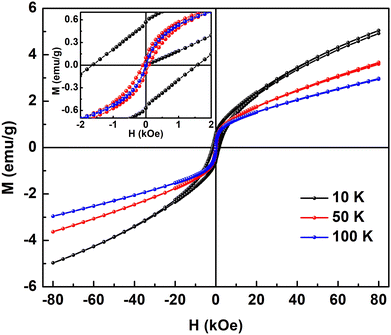 |
| Fig. 7
M–H loops of Sn0.5Fe0.5O2−δ at different temperatures and the inset shows the magnified view of MH loops at low magnetic fields. | |
To gain more insights into the ground state of Sn0.5Fe0.5O2−δ, ac susceptibility measurements were carried out. As shown in Fig. 8a, the real part of ac susceptibility shows a frequency dependent peak which is typical in spin glass (SG), cluster spin glass (CSG), and SPM systems. Generally, the Mydosh parameter, δ = ΔTf/Tf[Δlog
f], which quantifies relative peak temperature shift per decade of frequency is employed to distinguish canonical spin glass (CG) (0.005), SG (0–0.01), CSG (0.01–0.1) and SPM (0.1–1) systems.69–72 The estimated Mydosh parameter for Sn0.5Fe0.5O2−δ is 0.06(1) which suggests the system to be a cluster spin glass. This value is larger than 0.0189 found in nanocrystalline Sn0.95Sn0.05O211 and 0.05(1) for Sn0.9Fe0.1O2−δ12 which reported SG behaviour. Furthermore, the frequency dependent peak temperature (TP) is analyzed in terms of the critical slowing down (CSD) model,69
where τ0 is the relaxation time, Tf is the glass temperature and zv is the critical exponent. As shown in Fig. 8b, the CSD model describes TP(ω) data well yielding τ0 = 4.7(3) × 10−9 s, Tf = 67.9(8) K, and zv = 9.2(5). For canonical SG: τ0 = 10−12–10−13 s; for conventional spin glass: τ0 = 10−10–10−13 s (zv = 4–12) while τ0 = 10−7–10−10 s for cluster spin glass.70–73,75,76 Therefore, Sn0.5Fe0.5O2−δ is a cluster spin glass at low temperatures.
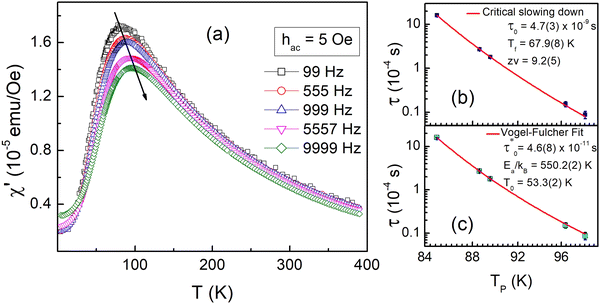 |
| Fig. 8 (a) Real part of the ac susceptibility as a function of temperature at a fixed driving field amplitude and different frequencies. Frequency-dependent peak temperature TP fit using (b) the critical slowing down model and (c) the Vogel–Fulcher law for Sn0.5Fe0.5O2−δ. | |
Moreover, fit based on the Vogel–Fulcher law,69,70
, where Ea/kB is the activation energy per Boltzmann constant and T0 is the coupling strength, yield
Ea/kB = 550.2(2) K and T0 = 53.3(2) K reconfirming the glassiness of the system (Fig. 8c).
is comparable to τ0 = 3 × 10−10 s found for Sn0.9Fe0.1O2−δ12 wherein Fe exists in +2 and +3 oxidation states. For T0 = 0, this law reduces to the Arrhenius law73 often employed for SPM systems which clearly fails in the current context. Assuming the cluster's size to be related to their coupling strength, the ratio of Ea/kB and T0 is used as a measure of coupling/clustering strength among the dynamic entities that freeze at Tf. Ea/(kBT0) < 1 signifies the strong coupling between the magnetic entities while Ea/(kBT0) > 1 indicates the weak coupling68,74 In our case, Ea/kB ∼ 10T0, suggests a weak coupling among the clusters and Ea/(kBT0) > 1 had been observed in cluster SG systems75,76 which is consistent with our system. In view of the above observations, the cluster spin glass state of Sn0.5Fe0.5O2−δ at low temperatures results due to the competing AFM and FM interactions. The FM character of the clusters is reflected in the finite values of Hc.
When a trivalent iron (Fe3+:
3d5, S = 5/2) replaces a tetravalent tin in the crystallographic structure, charge neutrality ensures the creation of one oxygen vacancy per two iron ions in the lattice. Furthermore, lower ionic radii Fe3+ (0.069 nm) substituting for Sn4+ (0.083 nm) retains the rutile structure of pristine SnO2 but disturbs the arrangement of the oxygen octahedral cage wherever it replaces the Sn ion. This is reflected in the large electric quadrupole splitting. The chemical formula based on XPS analysis can be written as Sn4+0.5Fe3+0.5O2−2−δ where δ is the oxygen off-stoichiometry associated with the presence of oxygen vacancies. In epitaxial thin films of Sn1−xFexO2 (x = 0–0.4),26 it was argued that a substantial fraction of Fe led charge compensation results in oxygen vacancies and holes. Room temperature saturation moment (coercivity) increased from 0.12 μB/Fe (300 Oe) for x = 0.1 to 0.71 μB/Fe (800 Oe) for x = 0.4. The origin of ferromagnetism26 was attributed to dominant FM double exchange (Fe2+−O2−−Fe3+ and Fe3+−O2−−Fe2+) interactions over AFM superexchange (Fe3+−O2−−Fe3+ and Fe2+−O2−−Fe2+) interactions. On the other hand, the donor impurity band exchange mechanism5 was proposed to explain the existence of large moments and ferromagnetism in dilute ferromagnetic oxides. In particular, in the case of the Fe–SnO2 system, F-center exchange mechanism47 was invoked wherein F-centers created due to oxygen vacancies (□) trap spin polarized electrons leading to ferromagnetic Fe3+−□−Fe3+ interactions that dominate over existing antiferromagnetic Fe3+−O2−−Fe3+ superexchange interactions. These shallow donor electrons form bound magnetic polarons coupling the adjacent magnetic cations within their hydrogenic radius (ν). For large enough ν, δ > δp (polaron percolation threshold) and x < xp (cation percolation threshold), this model5 predicts the occurrence of room temperature ferromagnetism. For the Sn1−xTMxO2 system, δp = 0.001 and xp = 0.25 where TM is the magnetic transition metal. When x > xp, antiferromagnetic or ferrimagnetic behavior is predicted as there are continuous paths throughout the lattice joining nearest-neighbor cations so much so that the net magnetic moment drastically vanishes. This impurity band model fails when δ increases, as the donor state merges with the bottom of the conduction band and the system behaves as a spin glass system whose description can be understood in terms of the Ruderman–Kittel–Kasuya–Yosida (RKKY) exchange interaction. In the present case of highly doped Sn0.5Fe0.5O2−δ (x = 0.5 and δ = 0.25), the Fe3+−Fe3+ interaction pathways are nearest neighbour (AFM Fe3+−O2−−Fe3+ and FM Fe3+−□−Fe3+) and next-nearest neighbour (Fe3+−O2−−Sn4+−O2−−Fe3+, Fe3+−□−Sn4+−O2−−Fe3+, Fe3+−O2−−Sn4+−□−Fe3+ and Fe3+−□−Sn4+−□−Fe3+) interactions. Among them, the nearest neighbour interactions (antiferromagnetic Fe3+−O2−−Fe3+ and ferromagnetic Fe3+−□−Fe3+) play a pivotal role in governing the magnetic behavior of the dilute Fe-doped SnO2 system (x < xp). However, in the Sn0.5Fe0.5O2−δ (x > xp and δ > δp) system, neither the donor impurity band exchange nor RKKY exchange mechanisms could explain the observed cluster spin glass behavior at low temperatures. More so, the contributions from next-nearest neighbour interactions, however small, become significant in addition to the existing nearest-neighbour (AFM Fe3+−O2−−Fe3+ and FM Fe3+−□−Fe3+) interactions as the probability of finding an Sn4+ ion as the nearest neighbor to Fe3+ ion is 1/2. Eventually, these competing interactions lead to the cluster glass behavior at low temperatures. The finite coercivity at temperatures T < Tg corresponds to those of the FM clusters embedded in the AFM matrix. The dominant AFM correlations of the matrix are reflected in the upturn in magnetization at low temperatures. We believe that as temperature increases above Tg, the AFM state survives and may transform into a paramagnetic state above 390 K.
Conclusions
A thermally decomposed nanocrystalline Sn0.5Fe0.5O2−δ sample was characterized by XRD, FTIR, FESEM, HRTEM, XPS, Mössbauer spectroscopy, bulk magnetization and ac susceptibility measurements. The Rietveld refined XRD data showed the single-phase compound (≈98%) exhibiting a tetragonal rutile structure and an average crystallite size of 4.3 nm. FTIR confirmed the presence of stretching vibration mode of the O–Sn–O group at ∼593 cm−1. FESEM displayed a platelet-like surface while HRTEM showed nanoparticle agglomeration and reconfirmed polycrystallinity through the SAED pattern. EDX confirmed the presence of Fe and Sn in equal proportions. XPS and Mössbauer studies verified the trivalent nature of Fe and its distorted oxygen environment due to oxygen vacancies. The magnetic study (M–H, M–T and ACS) showed evidence for (i) competing ferromagnetic and antiferromagnetic interactions involving Fe3+ ions and (ii) cluster spin glass state at low temperatures and magnetic ordering temperature much above room temperature.
Author contributions
A. S.: investigation, formal analysis, validation, and visualization. K. D.: investigation. M. V.: investigation, visualization, and formal analysis. S. S. M.: investigation, formal analysis, and visualization. Y. B.: investigation, formal analysis, methodology, visualization, and validation. R. T.: conceptualization, methodology, resources, supervision, and funding acquisition. All the authors contributed to writing the manuscript.
Conflicts of interest
There are no conflicts of interest to declare.
Acknowledgements
AS is thankful to CURAJ for the University Fellowship. KD & MV gratefully acknowledge UGC for Senior Research Fellowships. RT thanks the Council of Scientific and Industrial Research, India (grant no. 01(2970)/19/EMR-II dated: 20th June 2019 to Ramalingam Thirumoorthi). We are thankful to IISc Bangalore for FE-SEM and XPS analysis, MNIT Jaipur for HR-TEM, BARC Mumbai for Mössbauer spectroscopy, and CURAJ for instrumental facilities (PXRD and PPMS).
References
- G. A. Prinz, Magnetoelectronics, Science, 1998, 282, 1660–1663 CrossRef CAS PubMed.
- Y. Matsumoto, M. Murakami, T. Shono, H. Hasegawa, T. Fukumura, M. Kawasaki, P. Ahmet, T. Chikyow, S. Koshihara and H. Koinuma, Room-Temperature Ferromagnetism in Transparent Transition Metal–Doped Titanium Dioxide, Science, 2001, 291, 854–856 CrossRef CAS PubMed.
- S. A. Wolf, D. D. Awschalom, R. A. Buhrman, J. M. Daughton, S. V. Molnar, M. L. Roukes, A. Y. Chtchelkanova and D. M. Treger, Spintronics: A Spin-Based Electronics Vision for the Future, Science, 2001, 294, 1488–1495 CrossRef CAS PubMed.
- I. S. Elfimov, A. Rusydi, S. I. Csiszar, Z. Hu, H. H. Hsieh, H. J. Lin, C. T. Chen, R. Liang and G. A. Sawatzky, Magnetizing Oxides by Substituting Nitrogen for Oxygen, Phys. Rev. Lett., 2007, 98, 137202 CrossRef CAS PubMed.
- J. M. D. Coey, M. Venkatesan and C. B. Fitzgerald, Donor impurity band exchange in dilute ferromagnetic oxides, Nat. Mater., 2005, 4, 173 CrossRef CAS PubMed.
- A. S. Risbud, N. A. Spaldin, Z. Q. Chen, S. Stemmer and R. Seshadri, Magnetism in polycrystalline cobalt-substituted zinc oxide, Phys. Rev. B: Condens. Matter Mater. Phys., 2003, 68, 205202 CrossRef.
- M. Venkatesan, C. B. Fitzgerald, J. G. Lunney and J. M. D. Coey, Anisotropic ferromagnetism in substituted zinc oxide, Phys. Rev. Lett., 2004, 93, 177206 CrossRef CAS PubMed.
- I. S. Elfimov, S. Yunoki and G. A. Sawatzky, Possible Path to a New Class of Ferromagnetic and Half-Metallic Ferromagnetic Materials, Phys. Rev. Lett., 2002, 89, 216403 CrossRef CAS PubMed.
- K. Nomura, Magnetic properties and oxygen defects of dilute metal doped tin oxide based semiconductor, Croat. Chem. Acta, 2015, 88, 579–590 CrossRef CAS.
- X. L. Wang, Z. X. Dai and Z. Zeng, Search for ferromagnetism in SnO2 doped with transition metals (V, Mn, Fe, and Co), J. Phys.: Condens.Matter, 2008, 20, 045214 CrossRef.
- K. C. Verma and R. K. Kotnala, Realizing ferromagnetic ordering in SnO2 and ZnO nanostructures with Fe, Co, Ce ions, Phys. Chem. Chem. Phys., 2016, 18, 17565 RSC.
- C. E. R. Torres, A. F. Cabrera and F. H. Sánchez, Magnetic behavior of nanoclusters of Fe-doped SnO2, Physica B, 2007, 389, 176–179 CrossRef CAS.
- D. Manikandan and R. Murugan, Genesis and tuning of ferromagnetism in SnO2 semiconductor nanostructures: Comprehensive review on size, morphology, magnetic properties and DFT investigations, Prog. Mater. Sci., 2022, 130, 100970 CrossRef CAS.
- S. J. Liu, C. Y. Liu, J. Y. Juang and H. W. Fang, Room-temperature ferromagnetism in Zn and Mn codoped SnO2 films, J. Appl. Phys., 2009, 105, 013928 CrossRef.
- X. L. Wang, Z. X. Dai and Z. Zeng, Search for ferromagnetism in SnO2 doped with transition metals (V, Mn, Fe, and Co), J. Phys.: Condens. Matter, 2008, 20, 045214 CrossRef.
- C. B. Fitzgerald, M. Venkatesan, A. P. Douvalis, S. Huber, J. M. D. Coey and J. Bakas, SnO2 doped with Mn, Fe or Co: Room temperature dilute magnetic semiconductors, J. Appl. Phys., 2004, 95, 7390–7392 CrossRef CAS.
- J. Zhang, R. Skomski, L. P. Yue, Y. F. Lu and D. J. Sellmyer, Structure and magnetism of V-doped SnO2 thin films: effect of the substrate, J. Phys.: Condens. Matter, 2007, 19, 256204 CrossRef.
- N. H. Hong, J. Sakai, W. Prellier and A. Hassini, Transparent Cr-doped SnO2 thin films: ferromagnetism beyond room temperature with a giant magnetic moment, J. Phys.: Condens.Matter, 2005, 17, 1697–1702 CrossRef CAS.
- A. Punnoose, J. Hays, A. Thurber, M. H. Engelhard, R. K. Kukkadapu, C. Wang, V. Shutthanandan and S. Thevuthasan, Development of high-temperature ferromagnetism in SnO2 and paramagnetism in SnO by Fe doping, Phys. Rev. B: Condens. Matter Mater. Phys., 2005, 72, 054402 CrossRef.
- A. Punnoose, J. Hays, V. Gopal and V. Shutthanandan, Room-temperature ferromagnetism in chemically synthesized Sn1−xCoxO2 powders, Appl. Phys. Lett., 2004, 85, 1559–1561 CrossRef CAS.
- S. B. Ogale, R. J. Choudhary, J. P. Buban, S. E. Loftland, S. R. Shinde, S. N. Kale, V. N. Kulkarni, J. Higgins, C. Lanci, J. R. Simpson, N. D. Browning, S. D. Sarma, H. D. Drew, R. L. Greene and T. Venkatesan, High Temperature Ferromagnetism with a Giant Magnetic Moment in Transparent Co-doped SnO2-δ, Phys. Rev. Lett., 2003, 91, 077205 CrossRef CAS PubMed.
- N. H. Hong, A. Ruyter, W. Prellier, J. Sakai and N. T. Huong, Magnetism in Ni-doped SnO2 thin films, J. Phys.: Condens.Matter, 2005, 17, 6533–6538 CrossRef CAS.
- S. Harbeck, A. Szatvanyi, N. Barsan, U. Weimar and V. Hoffmann, DRIFT studies of thick film un-doped and Pd-doped SnO2 sensors: temperature changes effect and CO detection mechanism in the presence of water vapour, Thin Solid Films, 2003, 436, 76–83 CrossRef CAS.
- C. V. Komen, A. Thurber, K. M. Reddy, J. Hays and A. Punnoose, Structure–magnetic property relationship in transition metal (M = V, Cr, Mn, Fe, Co, Ni) doped SnO2 nanoparticles, J. Appl. Phys., 2008, 103, 07D141 CrossRef.
- T. Sabergharesou, T. Wang, L. Ju and P. V. Radovanovic, Electronic structure and magnetic properties of sub-3
nm diameter Mn-doped SnO2 nanocrystals and nanowires, Appl. Phys. Lett., 2013, 103, 012401 CrossRef.
- H.-S. Kim, L. Bi, G. F. Dionne, C. A. Ross and H. J. Paik, Structure, magnetic and optical properties, and Hall effect of Co- and Fe-doped SnO2 films, Phys. Rev. B: Condens. Matter Mater. Phys., 2008, 77, 214436 CrossRef.
- K. Galatsis, L. Cukrov, W. Wlodarski, P. McCormick, K. Kalantarzadeh, E. Comini and G. Sberveglieri, p- and n-type Fe-doped SnO2 gas sensors fabricated by the mechanochemical processing technique, Sens. Actuators, B, 2003, 93, 562–565 CrossRef CAS.
- G. Sberveglieri, C. Perego, F. Parmigiani and G. Quattroni, Sn1-xFexOy: a new material with high carbon monoxide sensitivity, Sens. Actuators, B, 1994, 20, 163–167 CrossRef CAS.
- X.-T. Yin and X.-M. Guo, Selectivity and sensitivity of Pd-loaded and Fe-doped SnO2 sensor for CO detection, Sens. Actuators, B, 2014, 200, 213–218 CrossRef CAS.
- M. V. Vaishampayan, R. G. Deshmukh, P. Walke and I. S. Mulla, Fe-doped SnO2 nanomaterial: A low temperature hydrogen sulfide gas sensor, Mater. Chem. Phys., 2008, 109, 230–234 CrossRef CAS.
- S. Bose, S. Chakraborty, B. K. Ghosh, D. Das, A. Sen and H. S. Maiti, Methane sensitivity of Fe-doped SnO2 thick films, Sens. Actuators, B, 2005, 105, 346–350 CrossRef CAS.
- J. Hu, Y. Wang, W. Wang, Y. Xue, P. Li, K. Lian, L. Chen, W. Zhang and S. Zhuiykov, Enhancement of the acetone sensing capabilities to ppb detection level by Fe-doped three-dimensional SnO2 hierarchical microstructures fabricated via a hydrothermal method, J. Mater. Sci., 2017, 52, 11554–11568 CrossRef CAS.
- D. Toloman, A. Popa, M. Stan, C. Socaci, A. R. Biris, G. Katona, F. Tudorachec, I. Petrilad and F. Iacomi, Reduced graphene oxide decorated with Fe doped SnO2 nanoparticles for humidity sensor, Appl. Surf. Sci., 2017, 402, 410–417 CrossRef CAS.
- W. B. H. Othmen, N. Sdiri, H. Elhouichet and M. Férid, Study of charge transport in Fe-doped SnO2 nanoparticles prepared by hydrothermal method, Mater. Sci. Semicond. Process., 2016, 52, 46–54 CrossRef.
- J. Kaur, J. Shah, R. K. Kotnala and K. C. Verma, Raman spectra, photoluminescence and ferromagnetism of pure, Co and Fe doped SnO2 nanoparticles, Ceram. Int., 2012, 38, 5563–5570 CrossRef CAS.
- S. Sambasivam, B. C. Choi and J. G. Lin, Intrinsic magnetism in Fe doped SnO2 nanoparticles, J. Solid State Chem., 2011, 184, 199–203 CrossRef CAS.
- M. S. Pereira, T. S. Ribeiro, F. A. S. Lima, L. P. M. Santos, C. B. Silva, P. T. C. Freire and I. F. Vasconcelos, Synthesis and properties of Sn1−xFexO2 nanoparticles obtained by a proteic sol–gel method, J. Nanopart. Res., 2018, 20, 212 CrossRef.
- W. B. H. Othmen, Z. B. Hamed, B. Sieber, A. Addad, H. Elhouichet and R. Boukherroub, Structural and optical characterization of p-type highly Fe-doped SnO2 thin films and tunneling transport on SnO2:Fe/p-Si heterojunction, Appl. Surf. Sci., 2018, 434, 879–890 CrossRef.
- J. J. Beltran, L. C. Sánchez, J. Osorio, L. Tirado, E. M. Baggio-Saitovitch and C. A. Barrero, Crystallographic and magnetic properties of Fe-doped SnO2 nanopowders obtained by a sol–gel method, J. Mater. Sci., 2010, 45, 5002–5011 CrossRef CAS.
- K. K. Pandimeena, M. C. Robert and S. Saravanakumar, Energy gap tuning, ferromagnetic switching, visualization of electron density and bonding in dilute magnetic semiconductor Fe-doped SnO2, Optik, 2023, 287, 171091 CrossRef CAS.
- R. Adhikari, A. K. Das, D. Karmakar, T. V. C. Rao and J. Ghatak, Structure and magnetism of Fe-doped SnO2 nanoparticles, Phys. Rev. B: Condens. Matter Mater. Phys., 2008, 78, 024404 CrossRef.
- S. Ferrari, L. G. Pampillo and F. D. Saccone, Magnetic properties and environment sites in Fe doped SnO2 Nanoparticles, Mater. Chem. Phys., 2016, 177, 206–212 CrossRef CAS.
- M. S. Pereira, G. M. S. L. Mendes, T. S. Ribeiro, M. R. Silva and I. F. Vasconcelos, Influence of Thermal-Treatment Effects on the Structural and Magnetic Properties of Sn1−xFexO2 Nanopowders Produced by Mechanical Milling, J. Supercond. Novel Magn., 2020, 33, 1721–1728 CrossRef CAS.
- W. B. H. Othmen, B. Sieber, C. Cordier, H. Elhouichet, A. Addad, B. Gelloz, M. Moreau, A. Barras, M. Férid and R. Boukherroub, Iron addition induced tunable band gap and tetravalent Fe ion in hydrothermally prepared SnO2 nanocrystals: Application in photocatalysis, Mater. Res. Bull., 2016, 83, 481–490 CrossRef.
- W. B. H. Othmen, B. Sieber, H. Elhouichet, A. Addad, B. Gelloz, M. Moreau, S. Szunerits and R. Boukherroub, Effect of high Fe doping on Raman modes and optical properties of hydrothermally prepared SnO2 nanoparticles, Mater. Sci. Semicond. Process., 2018, 77, 31–39 CrossRef.
- L. Peng and T. Liu, Effects of Fe Doping on Defects, Structural Disorder, and Optical and Magnetic Properties of SnO2 Nanoparticles, J. Supercond. Novel Magn., 2021, 34, 1287–1296 CrossRef CAS.
- J. M. D. Coey, A. P. Douvalis, C. B. Fitzgerald and M. Venkatesan, Ferromagnetism in Fe-doped SnO2 thin films, Appl. Phys. Lett., 2004, 84, 1332–1334 CrossRef CAS.
- C. E. R. Torres, L. Errico, F. Golmar, A. M. M. Navarro, A. F. Cabrera, S. Duhalde, F. H. Sánchez and M. Weissmann, The role of the dopant in the magnetism of Fe-doped SnO2 films, J. Magn. Magn. Mater., 2007, 316, e219–e222 CrossRef CAS.
- W. Yu, K. Jiang, J. Wu, J. Gan, M. Zhu, Z. Hu and J. Chua, Electronic structures and excitonic transitions in nanocrystalline iron-doped tin dioxide diluted magnetic semiconductor films: an optical spectroscopic study, Phys. Chem. Chem. Phys., 2011, 13, 6211–6222 RSC.
- M. K. Jaiswal, R. Kumar, D. Kanjilal, C. L. Dong, C. L. Chen, K. Asokan and S. Ojha, Studies of dense electronic excitation-induced modification in crystalline Fe-doped SnO2 thin films, Appl. Surf. Sci., 2015, 332, 726–735 CrossRef CAS.
- Y. Fu, N. Sun, L. Feng, S. Wen, Y. An and J. Liu, Local structure and magnetic properties of Fe-doped SnO2 films, J. Alloys Compd., 2017, 698, 863–867 CrossRef CAS.
- M. M. B. Mohagheghi, N. Shahtahmasebi, M. R. Alinejad, A. Youssefi and M. S. Saremi, Fe-doped SnO2 transparent semi-conducting thin films deposited by spray pyrolysis technique: Thermoelectric and p-type conductivity properties, Solid State Sci., 2009, 11, 233–239 CrossRef.
- V. Chandrasekhar, S. Nagendran, S. Banzal, M. A. Kozee and D. R. Powell, An Iron Wheel on a Tin Drum: A Novel Assembly of a Hexaferrocene Unit on a Tin–Oxygen Cluster, Angew. Chem., Int. Ed., 2000, 39, 1833–1835 CrossRef CAS PubMed.
- V. Chandrasekhar, R. Thirumoorthi, K. Dhanwant and A. Saini, Photophysical studies of organostannoxane supported hexafluorophore assemblies, Inorg. Chim. Acta, 2021, 522, 120378 CrossRef CAS.
- S. P. Chand, Effect of pH Values on the Structural, Optical and Electrical Properties of SnO2 Nanostructures, Optik, 2019, 181, 768–778 CrossRef.
- Y. Wang, Y. Deng, L. Fan, Y. Zhao, B. Shen, D. Wu, Y. Zhou, C. Dong, M. Xing and J. Zhang, In situ strategy to prepare PDPB/SnO2 p–n heterojunction with a high photocatalytic activity, RSC Adv., 2017, 7, 24064–24069 RSC.
- H. Ji, Y. Fan, J. Yan, Y. Xu, X. She, J. Gu, T. Fei, H. Xu and H. Li, Construction of SnO2/graphene-like g-C3N4 with enhanced visible light photocatalytic activity, RSC Adv., 2017, 7, 36101–36111 RSC.
- L. Li, P. Ma, S. Hussain, L. Jia, D. Lin, X. Yin, Y. Lin, Z. Cheng and L. Wang, FeS2/carbon hybrids on carbon cloth: a highly efficient and stable counter electrode for dye-sensitized solar cells, Sustainable Energy Fuels, 2019, 3, 1749–1756 RSC.
- V. Kumar, V. Kumar, S. Som, J. H. Neethling, M. Lee, O. M. Ntwaeaborwa and H. C. Swart, The role of surface and deep-level defects on the emission of tin oxide quantum dots, Nanotechnology, 2014, 25, 135701 CrossRef PubMed.
- M. Wang, G.-M. Weng, G. Yasin, M. Kumar and W. Zhao, A high-performance tin phosphide/carbon composite anode for lithium-ion batteries, Dalton Trans., 2020, 49, 17026–17032 RSC.
- W. Li, F. Yang, B. Huang, Z. Liu, H. Meng, J. Zheng, B. Zeng, J. Yang, Y. Li, S. Xiao, Q. Chen and X. Zhao, Monodisperse SnO2/Co3O4 nanocubes synthesized via phase separation and their advantages in electrochemical Li-ion storage, Ionics, 2020, 26, 6125–6132 CrossRef CAS.
- Q. Gao, P. Li, S. Ding, H. He, M. Cai, X. Ning, Y. Cai and M. Zhang, Cu2Se-ZnSe heterojunction encapsulated in carbon fibers for high-capacity anodes of sodium-ion batteries, Ionics, 2020, 26, 5525–5533 CrossRef CAS.
- X. Liu, J. Zhang, X. Guo, S. Wang and S. Wu, Core–shell α–Fe2O3@SnO2/Au hybrid structures and their enhanced gas sensing properties, RSC Adv., 2012, 2, 1650–1655 RSC.
- X. Zhao, T. Wen, J. Zhang, J. Ye, Z. Ma, H. Yuan, X. Ye and Y. Wang, Fe-Doped SnO2 catalysts with both BA and LA sites: facile preparation and biomass carbohydrates conversion to methyl lactate MLA, RSC Adv., 2017, 7, 21678–21685 RSC.
- Q. Zhang, Y. Fan, W. Wang, N. Liu and J. Guan, Enhanced Water Oxidation Activity by Introducing Gallium into Cobalt-Iron Oxide System, ChemElectroChem, 2020, 7, 118–123 CrossRef CAS.
- P. Nag, S. Banerjee, Y. Lee, A. Bumajdad, Y. Lee and P. S. Devi, Sonochemical Synthesis and Properties of Nanoparticles of FeSbO4, Inorg. Chem., 2012, 51, 844–850 CrossRef CAS PubMed.
- A. Rajan, M. Sharma and N. K. Sahu, Assessing magnetic and inductive thermal properties of various surfactants functionalised Fe3O4 nanoparticles for hyperthermia, Sci. Rep., 2020, 10, 15045 CrossRef PubMed.
- P. Bag, P. R. Baral and R. Nath, Cluster spin-glass behavior and memory effect in Cr0.5Fe0.5Ga, Phys. Rev. B, 2018, 98, 144436 CrossRef CAS.
- Y. Bitla, S. N. Kaul and L. F. Barquín, Nonlinear susceptibilities as a probe to unambiguously distinguish between canonical and cluster spin glasses, Phys. Rev. B: Condens. Matter Mater. Phys., 2012, 86, 094405 CrossRef.
- G. Benka, A. Bauer, P. Schmakat, S. Säubert, M. Seifert, P. Jorba and C. Pfleiderer, Interplay of itinerant magnetism and spin-glass behavior in FexCr1−x, Phys. Rev. Mater., 2022, 6, 044407 CrossRef CAS.
- M. R. Chowdhury, M. S. Seehra, P. Pramanik, S. Ghosh, T. Sarkar and B. Weise, S. Thota. Antiferromagnetic short-range order and cluster spin-glass state in diluted spinel ZnTiCoO4, J. Phys.: Condens. Matter, 2022, 34, 275803 CrossRef CAS PubMed.
- S. K. Jena, T. Sarkar, M. RoyChowdhury, B. Weise, Y. Qi and S. Thota, Slow spin dynamics of cluster spin-glass spinel Zn(Fe1−xRux)2O4: role of Jahn–Teller active spin-1/2 Cu2+ ions at B-sites, J. Phys.: Condens. Matter, 2022, 34, 405801 CrossRef CAS PubMed.
- R. Kumar, P. Yanda and A. Sundaresan, Cluster-glass behavior in the two-dimensional triangular lattice Ising-spin compound Li2Mn3O7, Phys. Rev. B, 2021, 103, 214427 CrossRef CAS.
- S. Shtrikman and E. P. Wohlfarth, The theory of the Vogel-Fulcher law of spin glasses, Phys. Lett. A, 1981, 85, 467–470 CrossRef.
- M. Roy-Chowdhury, M. S. Seehra and S. Thota, Optimized analysis of the AC magnetic susceptibility data in several spin-glass systems using the Vogel–Fulcher and Power laws, AIP Adv., 2023, 13, 115020 CrossRef CAS.
- S. Ghosh, D. C. Joshi, P. Pramanik, S. K. Jena, S. Pittala, T. Sarkar, M. S. Seehra and S. Thota, Antiferromagnetism, spin-glass state, H–T phase diagram, and inverse magnetocaloric effect in Co2RuO4, J. Phys.: Condens. Matter, 2020, 32, 485806 CrossRef CAS PubMed.
|
This journal is © The Royal Society of Chemistry 2024 |
Click here to see how this site uses Cookies. View our privacy policy here.