DOI:
10.1039/D3PY00970J
(Paper)
Polym. Chem., 2024,
15, 22-29
Preparation of fluorinated polyimides with low dielectric constants and low dielectric losses by combining ester groups and triphenyl pyridine structures†
Received
24th August 2023
, Accepted 23rd September 2023
First published on 11th December 2023
Abstract
As 5G communication develops rapidly, the traditional interlayer insulating dielectric material polyimide (PI) can no longer meet the requirements of large-scale integrated circuits, high-velocity, high-frequency communication, and other microelectronic applications, and urgently needs modification. In this paper, we superimpose the modification effects of bulk triphenyl pyridine structure and ester group structure and combine the bulk and low polarizability groups to successfully prepare a new type of PI with a low dielectric constant (Dk) and low dielectric loss (Df) at high frequency. The research focuses on the structure–property correlation of PIs, especially the effect of fluorine content on the dielectric properties. Firstly, three bulk diamine monomers (BPAB, PBAB3F, PBAB6F) containing a triphenyl pyridine structure were designed and synthesized, which were then polymerized with the 2,2-propenylidene-4,1-phenylene-1,3-dihydro-1,3-dioxo-5-isobenzofuran carboxylic acid ester (BTPDA) to obtain three new PIs with different fluorine contents (PI-EH, PI-ECF3, PI-E2CF3, respectively). Testing results show that the dielectric properties and moisture absorption of the PIs are improved with increased fluorine content. The new PIs showed excellent properties, such as low dielectric constants (Dk = 2.84–2.74 @ 10 GHz and Df = 0.00373–0.00338 @ 10 GHz), low water absorption (0.94–0.73%), and high hydrophobicity (contact angle = 77–97°), with PI-E2CF3 having the best overall performance. The present study was carried out to provide a reference for preparing high-frequency, low dielectric constant, and low-loss interlayer dielectric materials.
1 Introduction
In recent years, with the rapid development of 5G communications, it has become increasingly challenging for traditional interlayer dielectric insulating materials to meet the demand for signal propagation at high frequencies.1 Among them, crosstalk noise, signal delay, and loss caused by the intrinsically high dielectric constants (Dk) and high dielectric losses (Df) of interlayer dielectric materials are particularly prominent.2 To improve the quality of communication, the development of new interlayer insulation dielectric materials with excellent performance of low dielectric constant (Dk ≤ 3.0) and low dielectric loss (Df) has received increasing attention.3 Polyimide (PI) is widely used in the aerospace, microelectronics, and optoelectronics industries due to its excellent thermal stability, mechanical properties, and dielectric properties.4–6 However, it is challenging for conventional PIs (Dk = 3.34@10 GHz and Df = 0.01295@10 GHz for Kapton) to meet the performance requirements for high-frequency communication, especially millimeter-wave communication. Therefore, the modification of traditional PI is urgently needed to reduce their Dk and Df under high-frequency conditions and expand their microelectronic applications.
In general, the Dk of a polymer can be expressed according to the Clausius–Mossotti equation:7
|  | (1) |
where
Dk is the dielectric constant,
Pm is the molar polarizability and
Vm is the molar volume. From
eqn (1), the molar polarizability (
Pm) and molar volume (
Vm) are the two main factors affecting the
Dk of a material. Based on this theory, many ways of reducing
Dk can be deduced, such as (1) introducing unstable structures in the polymer chain to produce porosity or doping low-
Dk fillers
8,9 or (2) introducing low-polarity groups
7,10,11 and large-volume structural units.
12
Chan Hwang13 and others prepared porous PI (PPI) membranes using unstable dibutyl phthalate (a porogenic agent) and amino-capped oligo silsesquioxane (NH2-POSS) as the nucleating agent. The results show that the PIs present low dielectric properties (at least 2 at 1 MHz) as well as high thermal stability (Td5% = 519–559 °C) over a wide frequency and temperature range, among others. Moreover, the pore size of the PPI films can be modulated by NH2-POSS, which is a complicated process and is difficult to use widely. In addition to introducing unstable porogenic agents to prepare low-dielectric PIs, intrinsic low-dielectric PIs can also be designed by structural modulation. Common methods include introducing trifluoromethyl,7,14 bulky or twisted structure monomers,12,15 ester groups,16,17 and ether bonds.14 Liu18 and others designed and prepared a novel fluorinated polyimide film FPTTPI, which has an ultra-low Dk (1.52@1 kHz) attributed to the bulky structure as well as the dual effect of fluorine and the fluorinated PI film. In our previous work,12 we prepared three bulky diamine monomers containing a triphenyl pyridine structure, PDA, tPDA, and nPDA. We prepared them with 4,4′-(hexafluoroisopropanol) phthalic anhydride (6FDA) by solution polymerization to obtain PIs, and systematically investigated the relationship between the structure and properties of the PIs. The results showed that the molar polarizability per unit volume decreases with the increase of the side group volume, and thus the Dk and Df of PI decrease gradually. However, the rigid structure leads to a brittle PI. Therefore, it is an excellent choice to reduce the brittleness of PI thin films by introducing flexible groups such as ether bonds14 and ester groups.16,17 However, introducing flexible groups may weaken the restriction of polar group movement and thus enhance the Df of the resin.19 In summary, either trifluoromethyl, bulky side groups, or ether bonds/ester groups can help to reduce the Dk of PI, and the key lies in how to balance the relationship between them.
More and more researchers are recognizing the importance of interlayer insulating dielectric materials Df in the microelectronics industry. The equation for the signal transmission loss rate indicates that the magnitude of the signal transmission loss is directly proportional to the square root of Dk and linearly proportional to Df in terms of material properties.20 Consequently, it is also significant to reduce the dielectric loss of resins to improve the performance of resins in the microelectronics industry. Unfortunately, the relationship between the microstructure of the resin and its Df is unclear. Previous research in the literature has found that new resins with low Dk and low Df can be obtained by reducing the dipole content of the resin and restricting the dipole motion.20–22 Hence, an attempt is made in this paper to superimpose the combined modification effects of bulky triphenyl pyridine structures, a long-chain ester-based structure, and low-polarity trifluoromethyl structures with the expectation of preparing novel PIs with low Dk and low Df at high frequencies and investigating the correlation between their microstructures and macroscopic performances. Firstly, three bulk diamine monomers (BPAB, PBAB3F, PBAB6F) containing triphenyl pyridine structures with different fluorine contents were designed and synthesized based on the research foundation in the laboratory and polymerized with homemade long-chain ester-based anhydride 2,2-propylenebis-4,1-phenylene 1,3-dihydro-1,3-dioxo-5-isobenzofuran carboxylic acid ester (BTPDA) to obtain three novel PIs with different fluorine contents (PI-EH, PI-ECF3, PI-E2CF3). Among them, the coordination of diamine monomers containing triphenyl pyridine structures with varying contents of fluorine and long-chain ester-based anhydrides helps to reduce the molar polarizability of PI molecules and thus achieve the desired target. Based on the above strategies, new PI resins with excellent overall performance, such as low Dk, low Df, and low moisture absorption, were successfully prepared, and the findings are of some practical significance.
2 Experimental
2.1. Synthesis of the 2,2-propenylidene-4,1-phenylene-1,3-dihydro-1,3-dioxo-5-isobenzofuran carboxylate (BTPDA)
An anhydride containing an ester group was designed and prepared in this paper as follows (as shown in Scheme 1). Firstly, 1,3-dioxo-1,3-dihydroisobenzofuran-5-chloride (20 g, 39.5 mmol) and acetonitrile (60 ml) were placed in a 250 ml three-necked flask fitted with a mechanical stirrer, dissolved with stirring and cooled down to 5 °C. Subsequently, bisphenol A (9 g, 94.9 mmol) and pyridine (8 g, 0.1 mol) were dissolved in acetonitrile (18 ml) and slowly added dropwise to the flask using a constant pressure funnel. At the end of the dropwise addition, stirring was continued for 3 h. The progress of the reaction was monitored using TLC. When the reaction was finished, 72 ml of acetic acid was added to the flask and stirred vigorously to halt the reaction, distilled under reduced pressure to obtain a white slurry, filtered, and washed twice with acetonitrile to furnish a white powder, yielding the crude 2,2-propenyldi-4,1-phenylene-1,3-dihydro-1,3-dioxo-5-isobenzofuran carboxylic ester (16.2 g, yield: 70.4%). Lastly, the crude product was recrystallized using acetic anhydride (m(crude product)
:
V(acetic anhydride) = 1
:
1, recrystallization temperature: 140 °C) to give 15 g of pure anhydride in 92.5% yield. 1H NMR (500 MHz, DMSO-d6) δ = 8.39 (d, 2H), 8.31 (d, J = 10 Hz, 2H), 7.85 (d, J = 10 Hz, 2H), 7.36 (d, J = 15 Hz, 4H), 7.26 (d, J = 10 Hz, 4H), 1.72 (s, 6H); 13C NMR (126 MHz, DMSO-d6) δ = 168.78, 167.75, 164.89, 148.45, 138.77, 132.81, 131.17, 130.25, 128.87, 128.15, 121.79, 43.90, 31.58; FTIR (KBr): 1787 cm−1 and 1737 cm−1 (C
O), 1503 cm−1 and 711 cm−1 (benzene ring backbone vibration), 1355 cm−1 (methyl), 1231 cm−1 (C–O); HRMS (APCl, m/z): [M + H]+ calculated for C33H20O10+, 576.1056; found, 576.1065.
 |
| Scheme 1 Synthetic route to BTPDA anhydride. | |
2.2. Synthesis of PIs
Polyamide acids (PAA) were prepared by solution polymerization of novel diamine monomers containing triphenyl pyridine structures14 (PBAB, PBAB3F, PBAB6F) with different fluorine contents and long-chain ester-based anhydride (BTPDA) was made in-house as described in the previous section, and PIs were obtained by thermal imidization and were named as PI-EH-PAA, PI-ECF3-PAA, PI-E2CF3-PAA, PI-EH, PI-ECF3, PI-E2CF3, respectively. The synthetic routes for the three PIs were similar (as shown in Scheme 2), and PI-EH is presented below as an example. First, PBAP (2 g, 5.93 mmol) and DMAC were added to a 100 ml three-necked flask under a nitrogen atmosphere, and BTPDA (3.4147 g, 5.93 mmol) was added followed by mechanical stirring until complete dissolution and the polymer solid content was adjusted to 20 wt% with DMAC. The reaction was stirred at room temperature for 12 h, and then the insoluble impurities were filtered out and the solution was left to stand for 1 h to remove air bubbles. Then, the polymer solution was cast on a glass plate, and the film was scraped out with a spatula and dried in an oven at 80 °C for 2 h to remove solvents. Finally, the glass plate loaded with the polymer film was placed into a muffle furnace with the following temperature program to increase the temperature for thermal iminidation: 30–90 °C (3 °C min−1, holding time 50 min), 90–150 °C (3 °C min−1, holding time 50 min), 150–200 °C (3 °C min−1, holding time 50 min), 200–250 °C (3 °C min−1, holding time 50 min), 250–320 °C (3 °C min−1, holding time 50 min). At the end of the program, the glass plate was immersed in cold water until the PI film fell off naturally.
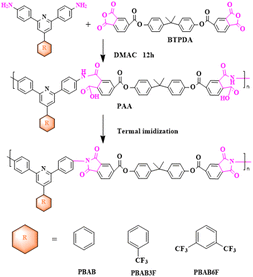 |
| Scheme 2 Synthesis route for PIs. | |
3 Results and discussion
3.1. Synthesis and characterization of PIs
The molecular weight of the resin has a significant influence on the performance of the resin.23 Not only is the molecular weight of the resin an influencing factor for the film-forming property of the PI, but it is also a key factor in ensuring the performance of the PI. The results of performing a GPC test on the three kinds of PAA are shown in Table 1. The results show that the number average molecular weights of the three PAAs are above 29
000, which is sufficient to meet the requirement of the film-forming property of PI.
Table 1 GPC data for the PAAs
PAAs |
Molecular weight |
M
n a × 104 |
M
w b × 104 |
M
w/Mn |
M
n: number-averaged molecular weight.
M
w: weight-averaged molecular weight.
|
PI-EH-PAA |
3.8 |
9.8 |
2.53 |
PI-ECF3-PAA |
2.9 |
6.3 |
2.18 |
PI-E2CF3-PAA |
3.2 |
7.0 |
2.16 |
The Fourier transform infrared spectra (FTIR) of PAA and PI were obtained, and the results are shown in Fig. 1. From the infrared spectra of PAA (Fig. 1(a)), it can be seen that amide shows N–H telescopic vibration absorption peaks at 3300 cm−1 and symmetric telescopic vibration peaks of C
O, C
N, C–O, and C–F appearing at 1727 cm−1, 1599 cm−1, 1205 cm−1, and 1132 cm−1, respectively.24,25 In addition, the skeletal vibrational absorption peaks of the benzene ring as well as the characteristic absorption peaks of the methyl group appeared at 1526 cm−1 and 1386 cm−1. They indicate that the PAA was successfully prepared according to the expected design. Infrared tests were performed on the PI, and the results are shown in Fig. 1(b). The characteristic absorption peak of amide at 3300 cm−1 (N–H) disappeared, and symmetric or asymmetric stretching vibration peaks of C
O and C–N bending vibrational stretching peaks appeared at 1775 cm−1, 1715 cm−1, and 716 cm−1, respectively, indicating the generation of imide ring.261H NMR was performed on PAA and the results are shown in Fig. S2.† From the results, it can be seen that all the obtained PAA showed carboxyl peaks at 10.7 ppm, as well as amino peaks at 6.7 ppm, and all the other main chain structures of hydrogen, which are represented in Fig. S2.†
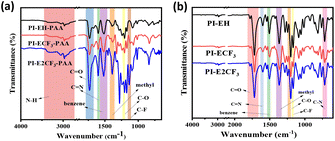 |
| Fig. 1 (a) FTIR plots of PAAs; (b) ATR-FTIR plots of PIs. | |
The WAXD test results for the PIs are shown in Fig. 2. The results show that all three PIs exhibit broad diffraction peaks, indicating amorphous nature. All three PIs show broad peaks at 2
θ = 6.5–33.2°, which represent the stacking of molecular chains.27 By comparison, it was found that 2
θ decreases gradually as the content of trifluoromethyl in PI increases. Bragg's equation25 shows an inverse relationship between 2
θ and the average spacing between molecular chains. It indicates that introducing trifluoromethyl causes an increase in the average spacing between molecular chains. This is attributed to the large size of trifluoromethyl as well as the strong electronegativity, which reduces the molecular chain stacking, and the strong electronegativity attenuates the interaction between the imide ring and the benzene ring, which then shifts in the direction of the small angle.
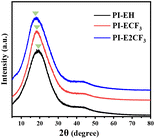 |
| Fig. 2 XRD curves of PI films. | |
3.2. Dielectric properties
The obtained PI films were subjected to dielectric tests, and the results are shown in Fig. 3 and Table 2. The results show that the Dk of PI is in the range of 2.84–2.74 @ 10 GHz, and the Df is in the range of 0.0037–0.0034@10 GHz. The dielectric properties of PI are improved to a great extent relative to those of commercial PI Kapton (Dk = 3.34@10 GHz, Df = 0.01295@10 GHz).28 It is evident that introducing more trifluoromethyl groups into the molecular chain reduces the Dk and Df of PIs. For the Dk, the introduction of a large volume of diamine monomers can firstly reduce the stacking of molecular chains and increase the average spacing between molecular chains, thus decreasing the Dk of PI. Secondly, the introduction of ester groups16,17 not only generates torsion angles due to their flexible characteristics, increasing the molar volume, but also the long-chain structure of the ester groups reduces the density of the polar structure (acrylamide ring) per unit volume, thus decreasing the molar polarizability. Furthermore, the introduction of a trifluoromethyl group in the molecular chain not only reduces the stacking of the molecular chain but also reduces the density of dipoles per unit volume, thus lowering the Dk of the PI.29 The primary factor determining the Df in a material is widely recognized to be the dissipation of energy resulting from the polarization of dipoles induced by alignment under an applied electric field. Firstly, the sizeable conjugated system of the triphenyl pyridine structure in the molecular skeleton reduces the density of the electron cloud in the molecule and reduces the internal consumption of the electron cloud due to the motion under high frequency. Secondly, the introduction of long-chain anhydride reduces the density of the dipole in the molecule. Finally, the introduction of electronegative trifluoromethyl not only limits the motion of the electron cloud and enhances the stability of the conjugated system by its strong electron-absorbing ability, but as a low-polarity group itself it also reduces the dipole density, which has a specific contribution to the reduction of the Df of PI. In conclusion, based on the above strategy of this paper, new PI resins with low Dk and low Df have been successfully prepared, among which PI-E2CF3 exhibits the lowest Dk (2.74@10 GHz) and Df (0.00338@10 GHz), which shows their great potential for application as interlayer dielectric materials.
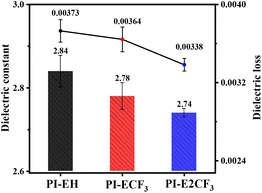 |
| Fig. 3 Dielectric properties of PI films. | |
Table 2 Optical properties, dielectric properties and moisture absorption of PI films
PIs |
λ0 a (nm) |
Transmittance b (%) |
D
k c |
D
f d × 10−4 |
M
a e (%) |
C
a f(°) |
Cutoff wavelength.
Transmittance at 550 nm.
D
k: dielectric constant.
D
f: dielectric loss (the Dk and Df of the PI films at 10 GHz are reported as averages of three experiments at room temperature).
M
a: moisture absorption.
C
a: water contact angle (film thickness: ∼50 μm).
|
PI-EH |
402 |
74 |
2.84 ± 0.04 |
37.3 ± 1.19 |
0.94 ± 0.014 |
77 ± 1.0 |
PI-ECF3 |
412 |
77 |
2.78 ± 0.03 |
36.4 ± 1.21 |
0.82 ± 0.0071 |
93 ± 1.0 |
PI-E2CF3 |
413 |
82 |
2.74 ± 0.01 |
33.8 ± 3.62 |
0.73 ± 0.028 |
97 ± 1.5 |
Furthermore, in order to further characterize the dielectric properties of the PIs, dielectric tests were performed under humid conditions (temperature: 30 °C, humidity: 86%). The test results show that the Dk and Df of the PIs are increased compared to under dry conditions (as shown in Fig. S4†), but are much better than those of the commercially available Kapton (Dk = 3.34@10 GHz, Df = 0.01295@10 GHz). It is noteworthy that due to the fact that the present hydrophobic structure trifluoromethyl can effectively block the water molecules from entering into the PI resin, the increase of both Dk and Df is decreased significantly when the fluorine content is gradually increased.
3.3. Absorbent properties
Hygroscopicity is one of the critical factors affecting the application value of interlayer dielectric materials.21,28,30 In the natural state, water molecules with larger Dk can easily enter into the PI film to increase the Dk of the material, thus affecting signal conduction at high frequencies. The PIs were tested for water absorption and contact angle, and the test results are shown in Fig. 4 and Table 2. From the water absorption test in Fig. 4(a), it can be seen that the water absorption of the resulting PIs is in the range of 0.94–0.73%, with PI-E2CF3 exhibiting the lowest absorption (0.73%), which is much lower than that of commercial PIs (1.5%).21 The contact angle results in Fig. 4(b) show that the contact angles of the PI films are all greater than 77°, with PI-E2CF3 having a contact angle as high as 97°. The resulting three PIs have low moisture absorption, with PI-E2CF3 being particularly outstanding. This is attributed to the high density of intrinsically hydrophobic aromatic rings31 in the main chain of the PI and the conjugated system formed by the triphenyl pyridine structure, which reduces the electron cloud density in the molecule and weakens the interaction with water molecules. Next, the introduction of the ester group32 in the anhydride reduces the hygroscopicity of the PI due to its intrinsic hydrophobicicity, but also the long-chain structure of the ester group reduces the density of the dipole per unit volume of the molecule and further reduces the water absorption of the material. Finally, the intrinsic hydrophobicity of trifluoromethyl33 is also a key reason for reducing the hygroscopicity of the material. It is easy to see that the hygroscopicity of the PIs gradually decreases with increasing fluorine content. In summary, the novel PI resins prepared based on the present strategy all have low hygroscopicity.
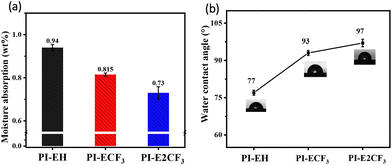 |
| Fig. 4 (a) Plot of water absorption results for PI films. (b) Plot of contact angle results for PI films. | |
3.4. Optical properties
Intramolecular and intermolecular formation of the charge transfer effect (CTC) is an essential reason for the yellow coloration of traditional aromatic PI.34–36 The UV-visible spectra of the prepared PI films were tested, and the results are shown in Fig. 5 and Table 2, which show that the cutoff wavelength (λ0) of the PI films is greater than 400 nm and the transmittance at 550 nm is greater than 74%, indicating excellent optical transparency. A comparison shows that, with the increase of the fluorine content, the λ0 of the PIs moves to the direction of a small wavelength, and the transmittance gradually increases. The reason for this is, firstly, the bulk diamine containing a triphenyl pyridine ring can increase the spacing of the molecular chains and reduce the formation of CTC between molecules. Secondly, the strong electron-absorbing ability of the trifluoromethyl group and the bulk structure also have a significant contribution. The strong electron-absorbing ability of trifluoromethyl can limit the charge transfer within the molecule, and the large volume can increase the average spacing between molecules and reduce the CTC effect between PI molecules. Consequently, the λ0 of the PI films gradually decreases, and the transmittance gradually increases with increasing fluorine content. However, the ester group in the anhydride also has a strong electron-absorbing ability, which increases the charge transfer in the molecule and leads to a certain yellow color for all the resulting PIs.
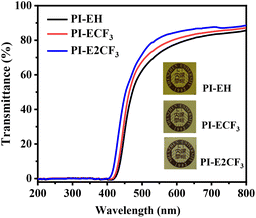 |
| Fig. 5 UV-vis spectra of PI films. | |
3.5. Mechanical properties
A Zwick tensile machine was used to test the mechanical properties of the PI films, and the results are shown in Fig. 7(a) and Table 3. The tensile strength of the three kinds of PI films is 33.4–53.8 MPa, tensile modulus in the range of 1.71–2.02 GPa, and elongation at break in the range of 7.0–10.1%. It was found that the tensile strength, elongation at break, and tensile modulus of the PI films decreased to different degrees with increasing fluorine content. The two main processes of polymer stretching are molecular chain straightening and molecular chain slip.37,38 The main chain structures of the PIs in this paper are all obtained by polycondensation of triphenyl pyridine-containing structural diamines with long-chain ester-based anhydrides. The polymers have similar molecular weights (as shown in Table 1), so the different side groups are the main reason for the various mechanical properties. As the side groups change from –H to –CF3 and –2CF3, the fluorine content in the PI molecular chain gradually increases. Thanks to the large volume and strong electronegativity of trifluoromethyl, the average spacing between molecular chains and the interaction force between molecular chains gradually decreases.39 During the pre-stretching phase (as shown in Fig. 6), the straightening of the molecular chains is dominant. Under the premise of maintaining the consistency of the main chain, the more trifluoromethyl, the larger the average spacing of molecular chains, the less interaction force between molecular chains, and the easier it is to straighten, so the tensile strength and tensile modulus of PI show a decreasing trend with the increase of the fluorine content. Late in the stretching period, the relative slippage of molecular chains dominates. When the molecular chain becomes taut from the relaxed state, the side group becomes the main factor hindering the intermolecular chain slip. Thus, when the side group changes from –H to –2CF3, the volume of the side group increases, the restriction of molecular chain slip is enhanced, and the elongation at fracture of PI shows a decreasing trend.
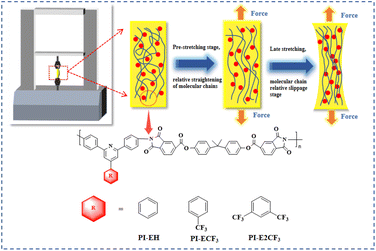 |
| Fig. 6 Schematic diagram of the stretching process of PI films. | |
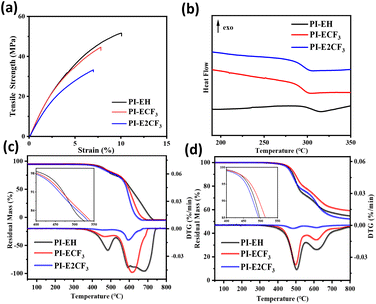 |
| Fig. 7 (a) Stress–strain curves for the PI films. (b) DSC curves of PIs. (c) TGA curves of PIs under air. (d) TGA curves of PIs under nitrogen. | |
Table 3 Mechanical properties of PI films
PIs |
Elongation at break (%) |
Tensile strength (MPa) |
Tensile modulus (MPa) |
PI-EH |
10.1 |
53.8 |
2024.1 |
PI-ECF3 |
7.8 |
46.5 |
2018.4 |
PI-E2CF3 |
7.0 |
33.4 |
1707.1 |
3.6. Thermal properties
DSC and TGA were used to test the thermal properties of the PIs, and the results are shown in Fig. 7 and Table 4. Table 4 shows that the glass transition temperatures (Tg) of the three kinds of PIs are above 294 °C. Under a nitrogen atmosphere, the 5 wt% weight loss temperatures of all three PIs are between 454 and 471 °C, and the residual carbon rates are all above 50%. Under air atmosphere, the 5 wt% weight loss temperatures of the three PIs are 433–448 °C. Taken together, the resulting PIs all show good thermal stability.
Table 4 Thermal properties of PIs
PIs |
T
g a (°C) |
In N2 |
In air |
T
d5% b (°C) |
T
d10% c (°C) |
C
y d (%) |
T
d5% e (°C) |
T
d10% f (°C) |
T
g was measured by DSC and the temperature rate was 20 °C min−1.
The 5% weight decomposition temperatures were measured by TGA in N2.
The 10% weight decomposition temperatures were measured by TGA in N2.
Char yield at 800 °C.
The 5% weight decomposition temperatures were measured by TGA in air.
The 10% weight decomposition temperatures were measured by TGA in air.
|
PI-EH |
305 |
464 |
484 |
55 |
448 |
475 |
PI-ECF3 |
296 |
471 |
494 |
59 |
439 |
473 |
PI-E2CF3 |
294 |
454 |
454 |
52 |
433 |
466 |
3.7. Solubility
Solubility tests were performed and the results are shown in Table 5. The results show that PI-ECF3 and PI-E2CF3 are soluble in DMF, NMP, and THF at room temperature; PI-EH is only soluble in DMF at room temperature. On the one hand, the large volume of the triphenyl pyridine structure trifluoromethyl disrupts the regularity of the molecular chains, increasing the spacing of the molecular chains, facilitating the diffusion of intermolecular solvent molecules into the chains,40,41 so that the PI-ECF3 and PI-E2CF3 exhibit better solubility; on the other hand, the ester-containing anhydride in the –OR has low polarity, weakening the interaction of the PI molecules with the solvent so that PI-EH has the worst solubility.
Table 5 Solubility tests of PIs
PIs |
DMF |
DMAC |
DMSO |
NMP |
THF |
Methylbenzene |
+: soluble at 30 °C; −: insoluble. The solubility was determined by adding 2 mg of PI into 1 ml of solvent. |
PI-EH |
+ |
− |
− |
− |
− |
− |
PI-ECF3 |
+ |
− |
− |
+ |
+ |
− |
PI-E2CF3 |
+ |
+ |
− |
+ |
+ |
− |
4. Conclusions
Traditional PIs were modified to meet the requirements for applications of PIs in the microelectronics industry. Based on the Clausius–Mossotti equation, a new strategy combining three modifications, namely, a triphenyl pyridine structure, a long-chain ester-based anhydride, and trifluoromethyl, is proposed in this paper for the preparation of PIs with excellent overall performance. Of the successfully prepared PIs, PI-2CF3 showed the best overall performance, presenting the advantages of low dielectric constant (Dk = 2.74 @ 10 GHz), low dielectric loss (Df = 0.00338 @ 10 GHz), low hygroscopicity (Ma = 0.73%, Ca = 97°), and better thermal stability (Tg = 297 °C, Td5% = 454 °C). PI-2CF3 possesses high transparency (λ0 = 402, transmittance = 82%) and outstanding solubility, and can be dissolved in NMP, DMF, DMAC, THF, etc., at room temperature. Accordingly, the PIs prepared by the present strategy exhibit great practical value for use in interlayer dielectric materials.
Author contributions
Hong Li: conceptualization, methodology, software, investigation, formal analysis, writing – original draft. Feng Bao: conceptualization, supervision, writing – editing. Shuanger Li, Xiyan Li, Yadong Li, Kexin Mu: data curation. Mingliang Wang: data curation, conceptualization. Caizhen Zhu: funding acquisition, supervision. Jian Xu: conceptualization, funding acquisition, resources.
Conflicts of interest
The authors have no conflicts of interest.
Acknowledgements
This work was supported by the open research fund of the National Natural Science Foundation of China (52303010), Key-Area Research and Development Program of Guangdong province (2019B010941001), Key Technology of Liquid Crystal Polymer Material for 5G/6G High-Frequency Communication (JSGGZD20220822095201003), Songshan Lake Materials Laboratory (2021SLABFK01) and the Guangdong Basic and Applied Basic Research Foundation (2021A1515110143).
References
- Z. Li, L. An, S. Khuje, J. Tan, Y. Hu, Y. Huang, D. Petit, D. Faghihi, J. Yu and S. Ren, Sci. Adv., 2021, 7, 7410 CrossRef PubMed.
- Z. Wu, J. Dong, X. Li, X. Zhao, C. Ji and Q. Zhang, Carbon, 2022, 198, 1–10 CrossRef.
- S. Dai, Y. Chu, D. Liu, F. Cao, X. Wu, J. Zhou, B. Zhou, Y. Chen and J. Huang, Nat. Commun., 2018, 9, 2737 CrossRef PubMed.
- Z. Dong, Q. He, D. Shen, Z. Gong, D. Zhang, W. Zhang, T. Ono and Y. Jiang, Microsyst. Nanoeng., 2023, 9, 31 CrossRef PubMed.
- Z. Chen, S. Liu, S. Yan, X. Shu, Y. Yuan, H. Huang and J. Zhao, Mater. Des., 2017, 117, 150–156 CrossRef CAS.
- A. I. Barzic, C. Hulubei, M. Asandulesa, G. Lisa, D. Popovici, I. Stoica, A. Nicolescu and R. M. Albu, Polym. Test., 2020, 90, 106704 CrossRef CAS.
- M. Zhong, X. Wu, C. Shu, Y. Wang, X. Huang and W. Huang, React. Funct. Polym., 2021, 169, 105065 CrossRef CAS.
- P. Zuo, J. Jiang, D. Chen, J. Lin, Z. Zhao, B. Sun and Q. Zhuang, ACS Appl. Mater. Interfaces, 2023, 15, 23792–23803 CrossRef PubMed.
- G. Qiu, W. Ma and L. Wu, Polym. Int., 2020, 69, 485–491 CrossRef.
- W.-F. Peng, H.-Y. Lei, X.-X. Zhang, L.-H. Qiu and M.-J. Huang, Chin. J. Polym. Sci., 2022, 40, 781–788 CrossRef.
- W. Peng, H. Lei, L. Qiu, F. Bao and M. Huang, Polym. Chem., 2022, 13, 3949–3955 RSC.
- S. E. Li, H. Zhu, F. Bao, X. Lan, H. Li, Y. Li, M. Ji, M. Wang, C. Zhu and J. Xu, Polymer, 2023, 283, 126245 CrossRef.
- Y. C. Hwang, S. Khim, J. M. Sohn and K.-H. Nam, Eur. Polym. J., 2023, 195, 112195 CrossRef.
- H. Li, F. Bao, X. Lan, S. Li, H. Zhu, Y. Li, M. Wang, C. Zhu and J. Xu, Eur. Polym. J., 2023, 197, 112327 CrossRef CAS.
- P. Xiao, X. He, F. Zheng and Q. Lu, Polym. Chem., 2022, 13, 3660–3669 RSC.
- Y. Watanabe, Y. Shibasaki, S. Ando and M. Ueda, J. Polym. Sci., Part A: Polym. Chem., 2004, 42, 144–150 CrossRef CAS.
- Y. Watanabe, Y. Shibasaki, S. Ando and M. Ueda, Chem. Mater., 2002, 14, 1762–1766 CrossRef CAS.
- Y. Liu, C. Qian, L. Qu, Y. Wu, Y. Zhang, X. Wu, B. Zou, W. Chen, Z. Chen, Z. Chi, S. Liu, X. Chen and J. Xu, Chem. Mater., 2015, 27, 6543–6549 CrossRef CAS.
- X. Huang, J. Wang, Q. Li, J. Lin and Z. Wang, Surf. Coat. Technol., 2019, 360, 205–212 CrossRef CAS.
- C. Qian, R. Bei, T. Zhu, W. Zheng, S. Liu, Z. Chi, M. P. Aldred, X. Chen, Y. Zhang and J. Xu, Macromolecules, 2019, 52, 4601–4609 CrossRef CAS.
- R. Bei, K. Chen, Y. He, C. Li, Z. Chi, S. Liu, J. Xu and Y. Zhang, J. Mater. Chem. C, 2023, 11, 10274–10281 RSC.
- Y. Li, T. Chen, Y. Liu, X. Liu and X. Wang, Polymer, 2022, 254, 125084 CrossRef CAS.
- H.-X. Chen, E.-S. Zhang, M. Hong, W. Liu, X.-M. Dai, Q. Chen, X.-P. Qiu and X.-L. Ji, Chin. J. Polym. Sci., 2019, 38, 629–637 CrossRef.
- X. He, S. Zhang, Y. Zhou, F. Zheng and Q. Lu, Polymer, 2022, 254, 125073 CrossRef CAS.
- X. Li, T. Liu, Y. Jiao, J. Dong, F. Gan, X. Zhao and Q. Zhang, Chem. Eng. J., 2019, 359, 641–651 CrossRef CAS.
- N. Song, K. Shi, H. Yu, H. Yao, T. Ma, S. Zhu, Y. Zhang and S. Guan, Eur. Polym. J., 2018, 101, 105–112 CrossRef CAS.
- Y. Wang, X. Zhou, X. Feng, X. Yu, S. Li, J. Zhao, Z. Fan, X. Sun, P. Zhang, J. Cui, M. Guo and B. Cheng, ACS Appl. Polym. Mater., 2022, 4, 5831–5839 CrossRef.
- I. Butnaru, M. Bruma and S. Gaan, RSC Adv., 2017, 7, 50508–50518 RSC.
- S. Wong, H. Ma, A. K. Y. Jen, R. Barto and C. W. Frank, Macromolecules, 2003, 36, 8001–8007 CrossRef CAS.
- S. R. Nagella and C. S. Ha, Nanomaterials, 2023, 13, 2090 CrossRef CAS PubMed.
- R. W. Gunasekara and Y. Zhao, Org. Lett., 2017, 19, 4159–4162 CrossRef CAS PubMed.
- D. H. Wang, R. N. McKenzie, P. R. Buskohl, R. A. Vaia and L.-S. Tan, Macromolecules, 2016, 49, 3286–3299 CrossRef CAS.
- N. Song, H. Yao, T. Ma, T. Wang, K. Shi, Y. Tian, B. Zhang, S. Zhu, Y. Zhang and S. Guan, Appl. Surf. Sci., 2019, 480, 990–997 CrossRef CAS.
- X. Yan, F. Dai, Z. Ke, K. Yan, C. Chen, G. Qian and H. Li, Eur. Polym. J., 2022, 164, 110975 CrossRef CAS.
- D. Li, C. Wang, X. Yan, S. Ma, R. Lu, G. Qian and H. Zhou, Polymer, 2022, 254, 125078 CrossRef CAS.
- J. Jun, J.-H. Lee, H.-J. Choi, S. Moon, I.-D. Kim and H. Lee, Appl. Surf. Sci., 2017, 423, 881–886 CrossRef CAS.
- C. R. Lopez-Barron, W. R. Burghardt and M. S. Kweon, ACS Macro Lett., 2020, 9, 26–31 CrossRef PubMed.
- Y. Lu, L. An, S.-Q. Wang and Z.-G. Wang, Macromolecules, 2014, 47, 5432–5435 CrossRef.
- S. Ma, S. Wang, S. Jin, Y. Wang, J. Yao, X. Zhao and C. Chen, Polymer, 2020, 210, 122972 CrossRef.
- H. Choi, I. S. Chung, K. Hong, C. E. Park and S. Y. Kim, Polymer, 2008, 49, 2644–2649 CrossRef.
- K. Hu, Q. Ye, Y. Fan, J. Nan, F. Chen, Y. Gao and Y. Shen, Eur. Polym. J., 2021, 157, 110566 CrossRef.
|
This journal is © The Royal Society of Chemistry 2024 |
Click here to see how this site uses Cookies. View our privacy policy here.