DOI:
10.1039/D3PY01183F
(Paper)
Polym. Chem., 2024,
15, 30-39
Fabrication of poly(4-vinylpyridine)-b-polystyrene-b-poly(4-vinylpyridine) triblock copolymer particles via three-dimensional soft confined self-assembly†
Received
23rd October 2023
, Accepted 23rd November 2023
First published on 23rd November 2023
Abstract
Using cetyltrimethylammonium bromide (CTAB) as a single surfactant, a series of symmetrical poly(4-vinylpyridine)-b-polystyrene-b-poly(4-vinylpyridine) (VmS24.2kVm) triblock copolymers with various molecular weights m of P4VP blocks (m = 3.0k, 6.3k, 7.9k, 13.4k) were employed to fabricate onion-like particles, spherical particles with an inner curved cylinder, convex lens (CL)-like particles with hexagonal P4VP cylinder and tulip-like particles with alternately stacked PS and P4VP lamella via three-dimensional soft confined self-assembly (3D-SCSA). Both the external shape and internal morphology of VmS24.2kVm particles were closely related to the volume fraction of the P4VP block and the concentration of CTAB. Upon increasing the volume fraction of the P4VP block, the internal phase separation structure of VmS24.2kVm particles changed from a cylinder to a lamella. Interestingly, as the concentration of CTAB increased, VmS24.2kVm particles changed from spherical (or onion-like) particles with a P4VP outmost layer to CL-like or tulip-like particles with a neutral interface, of which PS and P4VP blocks had an analogical preference to the oil/water interface, and finally into spherical particles with a PS outmost layer. The location of P4VP domains in VmS24.2kVm particles was clearly identified by selectively loading Au nanoparticles. It was the first time to observe VSV triblock copolymer particles with a neutral interface via 3D-SCSA using a single surfactant CTAB although it exhibits a preferred affinity and selective interaction with the PS block.
Introduction
During the past few decades, structure-transformable colloidal particles have drawn wide attention because of their unique shape and internal structure and have potential applications in photonic crystals,1,2 material delivery,3–5 catalysis,6,7 sensing,8etc. Block copolymers (BCPs), which can form periodic ordered microphase separation structures at the molecular chain scale, are ideal materials for self-assembly of structured particles.9–11 Compared to the self-assembly of BCPs in bulk and solution, three-dimensional soft confined self-assembly (3D-SCSA) can limit BCPs in a deformable small droplet, which is a powerful tool for fabricating polymer particles with adjustable shapes, internal phase structures and surface characteristics. Emulsification is a simple and convenient method to form 3D soft confined space.12–16 In the process of emulsion evaporation-induced 3D-SCSA, BCPs are dissolved using water-insoluble organic solvents, and the polymer solution is then emulsified into droplets in an aqueous solution containing surfactants. As the organic solvent slowly evaporates, polymer particles containing assorted assembly structures are obtained, such as spherical particles with curved cylinder, onion-like particles, pupa-like particles, tulip-like particles, convex-lens particles, Janus particles, reverse onion-like particles and so forth.17–23
Many factors like the degree of confinement,24 BCP characteristics,25 solvent,26 evaporation rate27 and surfactant properties23 would affect the formation of polymer particles by influencing the packing frustration of polymer chains and the interface interaction between the emulsion droplet and the surrounding medium.28–30 Among them, surfactants play an indispensable role in the 3D-SCSA process, as they not only stabilize emulsion droplets but also guide assembly through the interface interaction with BCPs. For example, Kim et al.18 employed asymmetrical polystyrene-b-poly(4-vinylpyridine) (PS27k-b-P4VP7k) diblock copolymers to form spherical particles with a worm-like internal morphology and a PS outmost layer using 1.0 mg mL−1 cetyltrimethylammonium bromide (CTAB) as the surfactant. Onion-like particles with a PS outmost layer were formed from the symmetrical polystyrene-b-poly(2-vinylpyridine) (PS102k-b-P2VP97k) diblock copolymer with 1.0 mg mL−1 CTAB.25 In these cases, CTAB was utilized as a single surfactant, which tended to make the PS domain remain on the particle surface due to its preferred affinity and selective interaction with the PS block.18,23,25 Generally, in order to obtain AB diblock copolymer particles with a neutral interface, of which A and B blocks have an analogical preference to the oil/water interface, the methods of using mixed surfactants and adding additives were adopted. For example, Hawker's group25 applied CTAB and hydroxylated CTAB (CTAB-OH) as mixed surfactants in 3D-SCSA. Due to the hydrophobic interaction between PS and CTAB and the hydrogen bonding between CTAB-OH and pyridine groups of the P4VP block, the boundary interaction could be precisely regulated by adjusting the mass fraction of CTAB-OH (X) in the mixed surfactants. They obtained a series of PS102k-b-P2VP97k polymer particles ranging from onion-like particles with a PS outmost layer (X = 0) to bud-like and ellipsoid-like particles with axially stacked lamella, and finally to reverse onion-like particles with a P4VP outmost layer (X = 1).25 Similarly, Kim et al.18 used poly(N-isopropylacrylamide) (PNIPAM), which has a preferred affinity toward the P4VP block, and CTAB, which has a preferred affinity toward the PS block, as mixed surfactants for the preparation of pupa-like and convex lens (CL)-like PS27k-b-P4VP7k polymer particles with a neutral wetting interface. The interaction of the oil/water interface could also be regulated by adding an additive strategy in the presence of the surfactant. Zhu et al.23 observed the formation of CL-like PS51k-b-P4VP18k (PDP)0.2 polymer particles with a hexagonal P4VP cylinder by adding 3-n-pentadecylphenol (PDP) in the presence of 3.0 mg mL−1 CTAB. The introduction of PDP enhanced the selective effect of CTAB on the P4VP (PDP) block, resulting in CTAB aqueous solution having no preference for the PS and P4VP (PDP) domains and hence the formation of CL-like polymer particles with a neutral wetting interface.23 Such CL-like and tulip-like polymer particles with a neutral interface are desirable due to their wide designability and potential applications, but generally they could be only obtained with mixed surfactants or additives.20,31–33 In particular, if a surfactant that has similar selectivity for both A and B blocks was used for 3D-SCSA, AB diblock copolymer particles with a neutral surface might be generated. Zhu et al.17 demonstrated that poly(vinyl alcohol) (PVA) has similar selectivity for PS and P4VP blocks and pupa-like PS9.8k-b-P4VP10k particles with radially alternately stacked PS and P4VP lamellae and a neutral interface were obtained via 3D-SCSA using 3.0 mg mL−1 PVA as the surfactant.
Recently, 3D-SCSA of the AB-type diblock copolymer has gained increasing attention in both experimental and theoretical studies,26,34–36 but there are still few reports on multiblock copolymer systems (e.g., ABA, ABC) and more complex polymer architectures.23,37,38 For example, Qiang et al.39 prepared ellipsoidal particles with stacked PS/PB/PMMA layers using triblock terpolymers of polystyrene-b-polybutadiene-b-poly(methyl methacrylate) (PS-b-PB-b-PMMA) with 10 mg mL−1 CTAB. The surface of such ellipsoidal particles would show a patchy topography during solvent annealing in CHCl3 with PVA as a surfactant due to the preferential interaction between PMMA and PVA.39 Steinhaus et al.40 devised block-type polymer brushes with a PS-polylactide (PLA) diblock copolymer to fabricate spherical particles with internal networks and ellipsoidal particles with stacked lamella by using CTAB as the surfactant. As a result, the design of the BCP chain structure would broaden the avenues for producing multicompartment polymer particles via 3D-SCSA. Compared with AB diblock copolymers, ABA triblock copolymers have two identically free end blocks and the movement of the middle block is restrained by end blocks at the same time. According to the previous experimental and theoretical results in bulk, AB and ABA BCPs with similar volume fractions had a similar microphase separation morphology.41–44 However, it was speculated that the impact of different polymer architectures between AB and ABA BCPs on their self-assembly structures in a physical confinement environment will be enhanced by the breakage of symmetry, the confinement effect and the selective interaction at the oil/water interface.45–47
Herein, a series of symmetrical poly(4-vinylpyridine)-b-polystyrene-b-poly(4-vinylpyridine) (P4VP-b-PS-b-P4VP, abbreviated as VSV) BCPs, denoted as VmS24.2kVm with various molecular weights of m (m = 3.0k, 6.3k, 7.9k, 13.4k), were synthesized via two-step reversible addition–fragmentation chain transfer (RAFT) polymerization. The VSV triblock copolymer particles with different shapes and internal structures were constructed via emulsion evaporation induced 3D-SCSA by using CHCl3 as a solvent and CTAB as a single surfactant. The internal microphase separation structure of VSV polymer particles changed from a cylinder to a lamella as the P4VP volume fraction increased. The effects of CTAB concentration on the shape and internal morphology of VSV polymer particles were systematically investigated. VSV triblock copolymer particles with a P4VP selective interface, a neutral wetting interface, and a PS selective interface were obtained, respectively, when using various CTAB concentrations (0.1, 0.5, 1.0 and 5.0 mg mL−1). This was the first time that CL-like and tulip-like particles with a neutral interface have been observed in VSV triblock copolymers using a single CTAB surfactant although it exhibits preferred affinity and selective interaction with the PS block. The distribution of P4VP domains in VSV particles was further clarified by loading Au nanoparticles into the P4VP domains of the particles. Furthermore, the 3D-SCSA of PS-b-P4VP (S12.2kVn, n = 3.7k, 12.0k) diblock copolymers with similar volume fractions was also studied for comparison. It was confirmed that no CL-like and tulip-like particles were formed for PS-b-P4VP diblock copolymers via 3D-SCSA in a similar concentration range of CTAB from 0.1 to 1.0 mg mL−1.
Experimental section
Materials and chemicals
Styrene (St, 99%, Sinopharm Chemical Reagent Co. Ltd) was purified by passing through a basic alumina column to remove inhibitor 4-tert-butylcatechol (TBC). 4-Vinylpyridine (4VP, 95%, Sigma-Aldrich) was passed through a neutral alumina column to remove the inhibitor hydroquinone (HQ) before use. Azobis(isobutyronitrile) (AIBN, 98%, J&K) was recrystallized from ethanol three times. The chain transfer agent 2-(dodecylthiocarbonothioylthio)-2-methylpropanoic acid (TTCA) was synthesized according to the literature.48 Ethylene glycol (EO, Sigma-Aldrich) was purchased from J&K. Cetyltrimethylammonium bromide (CTAB) was purchased from Aladdin. HAuCl4·3H2O was obtained from Sinopharm Chemical Reagent Co. Ltd and stored at 4 °C away from light. Other reagents such as N,N-dimethylformamide (DMF), chloroform, hexane, tetrahydrofuran (THF), deionized water, 4-dimethylaminopyridine (DMAP), dicyclohexylcarbodiimide (DCC) and dichloromethane (DCM) were used without further purification.
Synthesis of a two-end chain transfer agent (CTA2)
CTA2 was synthesized by esterification between EO and TTCA. DMAP (0.62 g, 5 mmol), TTCA (9.15 g, 25 mmol), EO (0.62 g, 10 mmol) and 50 mL DCM were added into a three-neck flask at 0 °C under a N2 atmosphere. DCC (5.57 g, 27 mmol) dissolved in 50 mL DCM was dropwise added into the solution under a N2 atmosphere. The reaction continued at 0 °C for 1 h under stirring and then kept overnight at room temperature under stirring. After that, the white precipitate formed was removed by suction filtration to obtain a crude product. After rotary evaporation, the crude product was further purified by column chromatography with DCM
:
hexane = 1
:
1 (v/v) as the eluent and then recrystallized. The final product was collected through filtration and dried under vacuum for 48 h.
Synthesis of CTA-PS24.2k-CTA and PS12.2k-CTA
CTA-PS24.2k-CTA was prepared via a typical RAFT procedure. As-prepared CTA2 (0.15 g, 0.2 mmol) and St (13.72 mL, 120 mmol) were added into a 25 mL Schlenk bottle under stirring and a N2 atmosphere until fully dissolved. The mixed solution was degassed by freezing–evacuation–thawing thrice and then put into an oil bath of 140 °C. After 2 h, the reaction was stopped by freezing with liquid nitrogen. The product was diluted with THF and precipitated in methanol three times. The precipitate was dried at 40 °C under vacuum overnight to give a yellow product. PS12.2k-CTA was synthesized via a similar RAFT procedure with TTCA (0.2 g, 0.5 mmol) and St (9.47 mL, 82 mmol).
Synthesis of VmS24.2kVm triblock copolymers and S12.2kVn diblock copolymers
RAFT polymerization was applied to synthesize VmS24.2kVm and S12.2kVn BCPs. For example, the macro-chain transfer agent CTA-PS24.2k-CTA (0.5 g, 0.02 mmol), 4VP (2.30 mL, 20 mmol) and 2 mL DMF were sequentially added into a Schlenk tube with stirring under N2 protection. After three freezing–evacuation–thawing cycles, the mixture was put into an oil bath at 70 °C. The reaction was ended with freezing by liquid nitrogen after preset times. The crude product was diluted with CHCl3 and precipitated three times with a hexane
:
ether mixture = 3
:
1 (v/v). The precipitate was dried at 40 °C under vacuum overnight to give a light yellow product, VmS24.2kVm. S12.2kVn was synthesized using PS12.2k-CTA as a macro chain transfer agent and 4VP as the monomer in DMF with a similar procedure.
Preparation of VmS24.2kVm and S12.2kVn BCP polymer particles via 3D-SCSA with CTAB as the single surfactant
The VmS24.2kVm or S12.2kVn BCPs were dissolved in a good solvent, CHCl3, to give 1 wt% solution. 0.1 mg mL−1, 0.5 mg mL−1, 1.0 mg mL−1 and 5.0 mg mL−1 CTAB aqueous solutions were prepared prior to use. Subsequentially, 0.1 mL BCP solution was emulsified with 1.0 mL CTAB aqueous solution by vortexing at 2400 rpm and ultrasonication. After slow evaporation of CHCl3 in ambient environment at room temperature for 48 h, polymer particles were formed and the redundant surfactants were removed by repeating centrifugation at 10
000 rpm for 20 min. The final polymer particles were redispersed in deionized water for subsequent experiment and characterization.
Loading of Au nanoparticles into P4VP domains
To further characterize the distribution of the P4VP phase in polymer particles, Au nanoparticles were introduced. An aqueous solution of the gold precursor (HAuCl4·3H2O) was added to an aqueous dispersion of polymer particles at a ratio of [Au3+]
:
[4VP] = 1
:
1 and the mixture was gently stirred overnight. The polymer particles were then purified by washing with deionized water and centrifugation three times at 10
000 rpm for 20 min. The final polymer particles with loaded Au nanoparticles were redispersed in deionized water for further characterization.
Characterization
Small angle X-ray scattering (SAXS) was performed at BL16B1 beam line in Shanghai Synchrotron Radiation Facility (SSRF). The wavelength of the X-ray was 1.24 Å and the distance between the sample and the detector was 2276 mm. Silver behenate was used as the standard sample to calibrate the scatter vector and each exposure time was 20 s. Samples for SAXS were prepared as follows: 5 wt% CHCl3 solution of VSV BCPs was slowly evaporated at room temperature for 7 days and then thermally annealed at 160 °C for 7 days under vacuum. The polydispersity index (Đ) values of VmS24.2kVm and S12.2kVn BCPs, CTA-PS-CTA and PS-CTA were determined by gel permeation chromatography (GPC) on a Waters system at 40 °C with a flow rate of 1 mL min−1. For VSV and SV BCPs, DMF was used as the eluent, whereas for CTA-PS-CTA and PS-CTA, THF was used as the eluent. 1H NMR spectra were recorded on a Bruker AVANCE400 NMR spectrometer (400 MHz) with deuterated chloroform (CDCl3) as the solvent. Transmission electron microscopy (TEM, Hitachi HT-7820 at an acceleration voltage of 120 keV) and field-emission scanning electron microscopy (FE-SEM, Hitachi S-4800) were used to observe the shape and internal structure of polymer particles. For TEM characterization, 20 μL particle dispersions were added dropwise on a copper grid and dried naturally, and the internal structure of polymer particles was characterized after staining with iodine vapor for 2 h. For the SEM sample, 10 μL particle dispersions were added dropwise on silicon wafer. After drying at room temperature, the sample was sputtered with gold before SEM observation.
Results and discussion
Synthesis and characterization of VmS24.2kVm triblock copolymers
Four VmS24.2kVm (m = 3.0k, 6.3k, 7.9k, 13.4k) BCPs with different molecular weights of P4VP blocks were synthesized via two-step RAFT polymerization. The synthetic route is presented as Scheme S1.†m was calculated from the 1H NMR spectrum of the triblock copolymer. Fig. 1a shows representative 1H NMR spectra of CTA-PS24.2k-CTA and V13.4kS24.2kV13.4k. GPC traces indicated that VmS24.2kVm triblock copolymers had narrow molecular weight distributions, as shown in Fig. 1b. The GPC trace of CTA-PS24.2k-CTA is given in Fig. S1.†
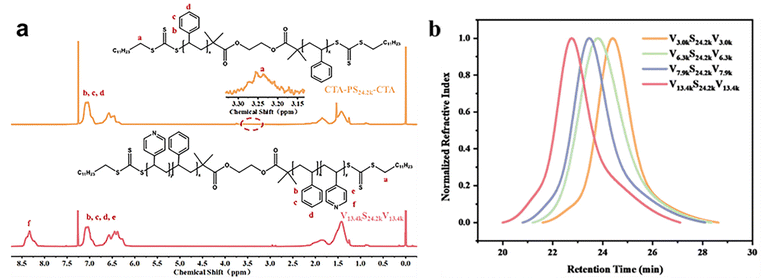 |
| Fig. 1 (a) Representative 1H NMR spectra of CTA-PS24.2k-CTA and V13.4kS24.2kV13.4k in CDCl3. (b) GPC traces of V3.0kS24.2kV3.0k, V6.3kS24.2kV6.3k, V7.9kS24.2kV7.9k and V13.4kS24.2kV13.4k triblock copolymers. | |
The microphase separation behaviour of VmS24.2kVm (m = 3.0 k, 6.3 k, 7.9 k, 13.4 k) BCPs in bulk was studied by small angle X-ray scattering (SAXS). Fig. 2 shows the SAXS profiles of V3.0kS24.2kV3.0k, V6.3kS24.2kV6.3k, V7.9kS24.2kV7.9k, and V13.4kS24.2kV13.4k. The V7.9kS24.2kV7.9k and V13.4kS24.2kV13.4k triblock copolymers showed lamellar morphologies, as indicated by the relative scattering vector (q/q* = 1
:
2
:
3: …) of the higher order peaks q and primary peak q*. The domain spacings (D) calculated using D = 2π/q* were about 27.0 nm for V7.9kS24.2kV7.9k and 32.6 nm for V13.4kS24.2kV13.4k, respectively. The V6.3kS24.2kV6.3k and V3.0kS24.2kV3.0k triblock copolymers showed cylindrical morphologies, as indicated by q/q* = 1
:
31/2
:
2
:
71/2: …, in which P4VP formed cylinders within the PS matrix. The domain spacings (D) of cylindrical structures were about 27.6 nm for V6.3kS24.2kV6.3k and 19.2 nm for V3.0kS24.2kV3.0k, respectively. The molecular weight (Mn), the volume fraction (f), the polydispersity indexes (Đ) and bulk microphase structure of VmS24.2kVm triblock copolymers are summarized in Table 1.
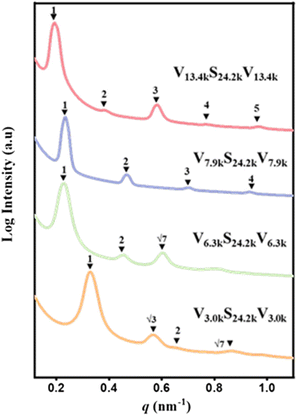 |
| Fig. 2 SAXS profiles of V3.0kS24.2kV3.0k, V6.3kS24.2kV6.3k, V7.9kS24.2kV7.9k, and V13.4kS24.2kV13.4k triblock copolymers in bulk at room temperature. | |
Table 1 Characteristics of VmS24.2kVm triblock copolymers
Sample |
M
n a (kg mol−1) |
f
PS b |
f
P4VP b |
Đ
|
Morphology in bulk |
Determined by 1H NMR.
f represented the volume fraction. The densities of PS and P4VP are 1.05 g cm−3 and 1.10 g cm−3, respectively.
Polydispersity indexes (Đs) were obtained by GPC.
|
V3.0kS24.2kV3.0k |
30.2 |
80.7 |
19.3 |
1.13 |
Cylinder |
V6.3kS24.2kV6.3k |
36.8 |
66.9 |
33.1 |
1.16 |
Cylinder |
V7.9kS24.2kV7.9k |
40.0 |
61.8 |
38.2 |
1.18 |
Lamella |
V13.4kS24.2kV13.4k |
51.0 |
46.6 |
53.4 |
1.20 |
Lamella |
Morphology and internal phase structure of VmS24.2kVm triblock copolymer particles
VmS24.2kVm polymer particles were prepared by typical evaporation-induced 3D-SCSA using vortexing and ultrasonication. A CHCl3 solution of the VSV triblock copolymer was stabilized in emulsion droplets using a single CTAB surfactant. CTAB with a long alkyl chain exhibits preferred affinity and selective interaction with PS.25 As CHCl3 slowly evaporated, glassy polymer particles were solidified and various internal phase separation structures were formed due to the strong incompatibility between PS and P4VP.49 The surface structure of VSV polymer particles under confined conditions was determined by the interfacial interaction between VSV and the surrounding medium. Fig. 3 shows the representative TEM images and corresponding SEM images of V3.0kS24.2kV3.0k particles obtained via 3D-SCSA with CTAB concentrations of 0.1, 0.5, 1.0 and 5.0 mg mL−1, respectively. The P4VP blocks of V3.0kS24.2kV3.0k formed a cylinder phase in bulk, which was consistent with the internal structure of polymer particles formed by 3D-SCSA. Under the conditions of 0.1 mg mL−1 CTAB, V3.0kS24.2kV3.0k formed spherical particles with the P4VP outmost layer and internal curved cylinders (Fig. 3a and e). The P4VP outmost layer was further verified by loading Au nanoparticles, which would be discussed later. Interestingly, when the concentration of CTAB increases to 0.5 and 1.0 mg mL−1, CL-like particles with a neutral interface and with hexagonal P4VP cylinders (Fig. 3b, f and c, g) were observed. Upon further increasing the CTAB concentration to 5.0 mg mL−1, spherical particles with a PS outmost layer and CL-like particles with a smaller size coexisted (Fig. 3d and h). It was likely that at a low concentration of 0.1 mg mL−1, the amounts of CTAB were not enough to drag the PS segments to the particle surface although CTAB had preferred affinity toward PS. The outmost surface of polymer particles was dominated by hydrophilic P4VP surrounded by aqueous solution. When the CTAB concentration reached 0.5 and 1.0 mg mL−1, the competition between the selective interaction of CTAB with the PS middle block and the polar interaction between P4VP end blocks and water molecules was balanced, leading to the formation of a neutral interface. Increasing the CTAB concentration of 5.0 mg mL−1 further strengthened the preferred interaction with the PS block, spherical particles with a PS outmost layer are thus formed in order to reduce the surface free energy of the particles. Zhu et al.23 also reported the formation of P4VP4.5k-b-PS38k-b-P4VP4.5k spherical particles with an internal curved cylinder at 3.0 mg mL−1 CTAB. The above results indicated that by simply adjusting the concentration of a single CTAB surfactant from 0.1 to 5.0 mg mL−1, spherical particles with a P4VP outermost layer, CL-like particles with a neutral interface, and spherical particles with a PS outermost layer could be fabricated for V3.0kS24.2kV3.0kvia 3D-SCSA. In particular, this was the first time, to the best of our knowledge, that CL-like particles with a neutral interface could be formed for VSV triblock copolymers using a single CTAB surfactant that even exhibits preferred affinity and selective interaction with the PS block.
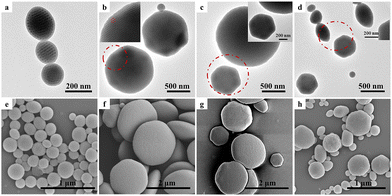 |
| Fig. 3 TEM and corresponding SEM images of V3.0kS24.2kV3.0k triblock copolymer particles obtained via 3D-SCSA with various CTAB concentrations of (a and e) 0.1, (b and f) 0.5, (c and g) 1.0, and (d and h) 5.0 mg mL−1. The TEM samples were stained with iodine vapor. | |
For V6.3kS24.2kV6.3k, which also exhibits a cylindrical structure in bulk, spherical particles with internal worm-like micellar structures and a P4VP outmost layer were formed via 3D-SCSA with a low CTAB concentration of 0.1 mg mL−1, as shown in Fig. 4a and e. Upon increasing the CTAB concentration to 0.5 mg mL−1, spherical particles with mixed lamellar and cylindrical inner structures were observed (Fig. 4b and f). For 3D-SCSA with CTAB concentrations of 1.0 and 5.0 mg mL−1, V6.3kS24.2kV6.3k spherical particles with mixed lamellar and cylindrical inner structures and the PS outmost layer were observed, as shown in Fig. 4c, d, g, and h. The polar interactions of hydrophilic P4VP blocks with the surrounding aqueous medium would be stronger than the preferred interaction between the PS block and CTAB when the CTAB concentration was 0.1 mg mL−1. As a result, P4VP blocks tended to accumulate on the surface of V6.3kS24.2kV6.3k particles. Consequently, the effective volume fraction of P4VP phases inside the particle reduced, leading to the formation of inner cylindrical P4VP phases. Upon increasing the CTAB concentration to 0.5, 1.0 or 5.0 mg mL−1, the preferred interaction between CTAB and the PS block was enhanced so that the PS block would migrate to the oil/water interface, leading to the decrease of the effective volume fraction of PS inside the polymer particles and the formation of a PS outmost layer. As a result, the inner phase structure of the surface regime of V6.3kS24.2kV6.3k particles changed from a cylinder to a lamella, forming the mixed lamellar and cylindrical inner structures. Furthermore, no CL-like particles with a neutral interface were observed for V6.3kS24.2kV6.3k in the range of CTAB concentrations from 0.1 to 5.0 mg mL−1.
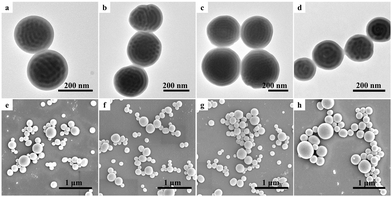 |
| Fig. 4 TEM and corresponding SEM images of V6.3kS24.2kV6.3k triblock copolymer particles obtained via 3D-SCSA with various CTAB concentrations of (a and e) 0.1, (b and f) 0.5, (c and g) 1.0, and (d and h) 5.0 mg mL−1. The TEM samples were stained with iodine vapor. | |
Fig. 5 shows the TEM and SEM images of V7.9kS24.2kV7.9k triblock copolymer particles formed via 3D-SCSA with various CTAB concentrations. Note that the V7.9kS24.2kV7.9k triblock copolymer exhibited a lamellar phase structure in bulk (cf.Fig. 2). When the CTAB concentration was low, i.e. 0.1 mg mL−1, onion-like V7.9kS24.2kV7.9k particles with a P4VP outmost layer were observed, as shown in Fig. 5a and e. Interestingly, upon increasing the CTAB concentration to 0.5 mg mL−1, the V7.9kS24.2kV7.9k triblock copolymer formed tulip-like particles with a neutral interface (Fig. 5b and f). Upon further increasing the CTAB concentration to 1.0 and 5.0 mg mL−1, reverse onion-like particles with a PS outmost layer appeared and coexisted with the tulip-like particles, as shown in Fig. 5c, d, g and h.
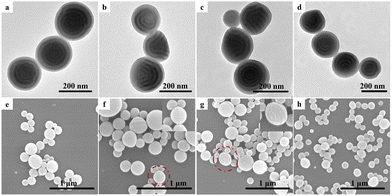 |
| Fig. 5 TEM and corresponding SEM images of V7.9kS24.2kV7.9k triblock copolymer particles obtained via 3D-SCSA with various CTAB concentrations of (a and e) 0.1, (b and f) 0.5, (c and g) 1.0, and (d and h) 5.0 mg mL−1. The TEM samples were stained with iodine vapor. | |
For the V13.4kS24.2kV13.4k triblock copolymer that also possessed a lamellar phase structure in bulk, the coexistence of onion-like and tulip-like particles with a P4VP outmost layer and axially stacked PS and P4VP lamellae was observed with a low CTAB concentration of 0.1 mg mL−1, as shown in Fig. 6a and e. For V13.4kS24.2kV13.4k with long P4VP end blocks, it tended to form low curvature tulip-like structures in order to release the packaging frustration of polymer chains.50 In such case, high curvature spherical particles were no longer the most advantageous structure. Upon increasing the CTAB concentration to 0.5, 1.0, and 5.0 mg mL−1, the V13.4kS24.2kV13.4k triblock copolymer only formed low curvature tulip-like particles with a neutral interface, as shown in Fig. 6b–d and f–h. The above results suggested that the morphology and internal phase structures of VSV triblock copolymer particles obtained via 3D-SCSA were not only dependent on the CTAB concentration but also on the composition of the triblock copolymer.
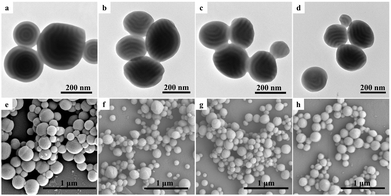 |
| Fig. 6 TEM and corresponding SEM images of V13.4kS24.2kV13.4k triblock copolymer particles obtained via 3D-SCSA with various CTAB concentrations of (a and e) 0.1, (b and f) 0.5, (c and g) 1.0, and (d and h) 5.0 mg mL−1. The TEM samples were stained with iodine vapor. | |
Loading Au nanoparticles in P4VP domains
The nitrogen atom of P4VP is capable of incorporating the inorganic guest. Therefore, the Au precursor (HAuCl4·3H2O) can be selectively loaded in P4VP domains, forming Au nanoparticles in P4VP domains after reduction,19,51 which can be used to identify the P4VP domains of VmS24.2kVm triblock copolymer particles. Fig. 7 shows the TEM images of VmS24.2kVm triblock copolymer particles formed via 3D-SCSA with CTAB concentrations of 0.1 and 1.0 mg mL−1, respectively, after loading of Au nanoparticles. For V3.0kS24.2kV3.0k, V6.3kS24.2kV6.3k, V7.9kS24.2kV7.9k and V13.4kS24.2kV13.4k spherical particles formed with a CTAB concentration of 0.1 mg mL−1, Au nanoparticles appeared on the surface of spherical particles, indicating that the outmost layer of these spherical particles was P4VP, as shown in Fig. 7a–d. For V3.0kS24.2kV3.0k CL-like particles formed with CTAB concentrations of 1.0 mg mL−1, Au nanoparticles were loaded in the hexagonal packed P4VP cylinders, as shown in Fig. 7e. Fig. 7f clearly shows that the outmost layer of V6.3kS24.2kV6.3k spherical particles formed with a CTAB concentration of 1.0 mg mL−1 was PS because no Au nanoparticles were observed on the surface of the spherical particles. For reverse onion-like V7.9kS24.2kV7.9k particles with a PS outmost layer and tulip-like V7.9kS24.2kV7.9k particles with alternately stacked PS and P4VP lamellae formed with a CTAB concentration of 1.0 mg mL−1, Au nanoparticles were clearly loaded in the P4VP lamellae, as shown in Fig. 7g. Similar results were also observed for tulip-like V13.4kS24.2kV13.4k particles with alternately stacked PS and Au nanoparticle-loaded P4VP lamellae (Fig. 7h).
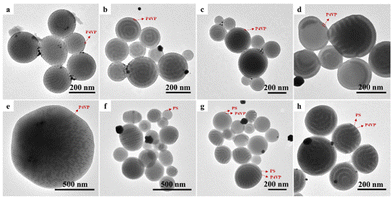 |
| Fig. 7 TEM images of (a) V3.0kS24.2kV3.0k, (b) V6.3kS24.2kV6.3k, (c) V7.9kS24.2kV7.9k, and (d) V13.4kS24.2kV13.4k triblock copolymer particles formed via 3D-SCSA with CTAB concentrations of 0.1 mg mL−1 after loading of Au nanoparticles. TEM images of (e) V3.0kS24.2kV3.0k, (f) V6.3kS24.2kV6.3k, (g) V7.9kS24.2kV7.9k, and (h) V13.4kS24.2kV13.4k triblock copolymer particles formed via 3D-SCSA with CTAB concentrations of 1.0 mg mL−1 after loading of Au nanoparticles. The TEM samples were stained with iodine vapor. | |
Morphology and internal phase structures of S12.2kVn diblock copolymer particles
For comparison, PS-b-P4VP (S12.2kVn, n = 3.7k, 12.0k) diblock copolymers, which had similar volume fractions of PS and P4VP blocks with those of V3.0kS24.2kV3.0k and V13.4kS24.2kV13.4k but half the molecular weights, were synthesized via RAFT polymerization. The GPC traces of PS12.2k-CTA, S12.2kV3.7k and S12.2kV12.0k diblock copolymers are shown in Fig. S2 and S3,† respectively. Table S1† lists the volume fractions of PS and P4VP blocks for the two diblock copolymers. An identical process of 3D-SCSA with various CTAB concentrations was applied to fabricate S12.2kV3.7k and S12.2kV12.0k diblock copolymer particles. For the S12.2kV3.7k diblock copolymer, spherical particles with inner curved P4VP cylinders were observed regardless of CTAB concentrations (0.1, 0.5 and 1.0 mg mL−1), as shown in Fig. 8a–c. For the S12.2kV12.0k diblock copolymer, onion-like spherical particles with concentric stacked lamellae were observed with CTAB concentrations of 0.1, 0.5 and 1.0 mg mL−1, as shown in Fig. 8d–f. The corresponding SEM images of S12.2kV3.7k and S12.2kV12.0k diblock copolymer particles are shown in Fig. S5.† No particles with a neutral interface (like CL-like or tulip-like particles) were observed for S12.2kV3.7k and S12.2kV12.0k diblock copolymers via 3D-SCSA with CTAB concentrations of 0.1, 0.5 and 1.0 mg mL−1. The above results were consistent with those reported in the previous works. For example, Hawker and coworkers25,35 reported the formation of PS102k-b-P2VP97k onion-like particles with concentric stacked lamellae and the PS outmost layer via 3D-SCSA with 1.0 mg mL−1 CTAB. Spherical particles with inner worm-like micellar structures and the PS outmost layer were obtained for asymmetric PS27k-b-P4VP7k with 1.0 mg mL−1 CTAB and PS51k-b-P4VP18k with 3.0 mg mL−1 CTAB.18,23 To date, no CL-like and tulip-like SV diblock copolymer particles with a neutral interface have been observed via 3D-SCSA using a single CTAB surfactant.
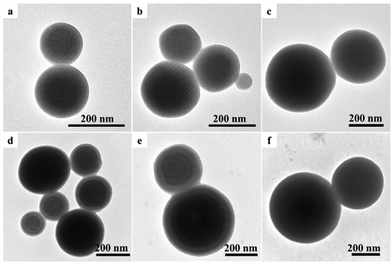 |
| Fig. 8 TEM images of S12.2kV3.7k diblock copolymer particles obtained via 3D-SCSA with various CTAB concentrations of (a) 0.1, (b) 0.5, (c) 1.0 mg mL−1. TEM images of S12.2kV12.0k diblock copolymer particles obtained via 3D-SCSA with various CTAB concentrations of (d) 0.1, (e) 0.5, (f) 1.0 mg mL−1. The samples were stained with iodine vapor. | |
Possible formation mechanism of VSV triblock copolymer particles
For the VSV triblock copolymer, the movement of the middle S block was significantly hindered by the two V free end blocks. However, the S and V blocks of the SV diblock copolymer simultaneously had S and V free end blocks. This thus meant that the middle S block of the VSV triblock copolymer requires more energy to come out of its domain.41 The obvious mobility discrepancy of S and V blocks along with the surface selective interactions during 3D-SCSA would have impacts on the formation of VSV triblock copolymer particles. Moreover, the middle PS block chains might form two possible chain conformations, i.e. loop and bridge conformations, during the phase separation of VSV triblock copolymer particles, as shown in Fig. 9a.42,52–54 The extended length (L) of the middle PS block with a zig-zag conformation is estimated to be about 58.4 nm by using LPS = 2NlC–C
sin(θ/2) (N is the degree of polymerization of PS, lC–C is the bond length of C–C, and θ is the bond angle of C–C), as shown in Fig. 9b. The domain spacings (d) of V3.0kS24.2kV3.0k CL-like particles and V13.4kS24.2kV13.4k tulip-like particles determined by TEM were about 22.4 nm and 29.0 nm, respectively (Fig. 9c and d), which were comparable to those of 19.2 nm and 32.6 nm for their domain spacings in bulk calculated by SAXS. Therefore, the segment chain of the middle PS block could form both loop and bridge conformations during the formation of VSV particles, as depicted in Fig. 9c and d.
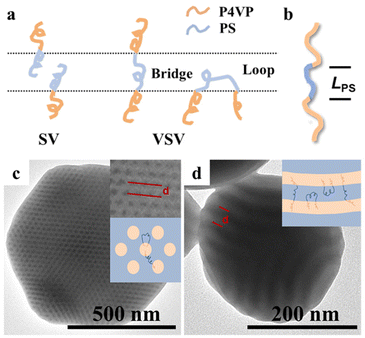 |
| Fig. 9 (a) Possible chain arrangement of the SV diblock copolymer and VSV triblock copolymer at the PS and P4VP domain interface. (b) Extended length (L) of the PS block. (c) CL-like V3.0kS24.2kV3.0k particles obtained via 3D-SCSA with a CTAB concentration of 1.0 mg mL−1. The insets show the domain spacing and possible chain arrangement. (d) Tulip-like V13.4kS24.2kV13.4k particles obtained via 3D-SCSA with a CTAB concentration of 1.0 mg mL−1. The insets show the domain spacing and possible chain arrangement. | |
Based on the experimental results discussed above, the shape and inner phase structures of VmS24.2kVm triblock copolymer particles obtained via 3D-SCSA were strongly dependent on the CTAB concentration and volume fraction of the P4VP block, as summarized in Fig. 10. Possibly, there were three competitive interactions during the formation of VSV triblock copolymer particles via 3D-SCSA with a CTAB surfactant: (a) the polar interaction between P4VP and surrounding water molecules, (b) the selective interaction between PS and CTAB, and (c) the packing frustration of the stretched VSV polymer chain. At low CTAB concentrations, the polar interactions between P4VP and water molecules were dominant, leading to the formation of VSV triblock copolymer particles with the P4VP outermost layer. Upon increasing the CTAB concentration, the PS middle block tended to move towards the oil/water interface due to the enhancement of the selective interaction between PS and CTAB. The balance of (a) and (b) interactions resulted in the formation of CL-like or tulip-like VSV particles with a neutral interface. However, the strong selective interaction between PS and CTAB with a high CTAB concentration would lead to the formation of VSV particles with a PS outmost layer. Furthermore, the shape of VSV particles was determined by the extent of the packing frustration of stretched VSV polymer chains. Non-spherical particles, such as CL-like and tulip-like VSV particles with low curvature, were formed in order to release the packing frustration of the polymer chain.
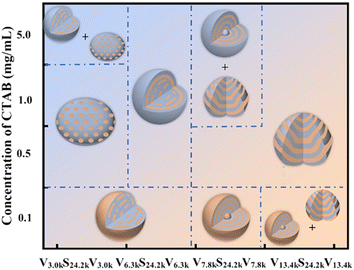 |
| Fig. 10 Summary of the shape and inner phase structures of VmS24.2kVm triblock copolymer particles obtained via 3D-SCSA with various CTAB concentrations of 0.1, 0.5, 1.0 and 5.0 mg mL−1. | |
Conclusion
VmS24.2kVm (m = 3.0k, 6.3k, 7.9k, 13.4k) triblock copolymer particles were successfully fabricated via 3D-SCSA with CTAB as the single surfactant. Onion-like particles with concentric stacked PS and P4VP lamellae and a P4VP outmost layer, spherical particles with an internal curved P4VP cylinder, CL-like particles with a hexagonal P4VP cylinder and neutral interface, tulip-like particles with axially stacked PS and P4VP lamellae and a neutral interface, and reverse onion-like particles with a PS outmost layer were obtained, depending on the composition of VmS24.2kVm triblock copolymers and the concentration of CTAB from 0.1 to 5.0 mg mL−1. This was the first time that CL-like and tulip-like particles with a neutral interface were observed for the VSV triblock copolymer via 3D-SCSA using a single CTAB surfactant. These results indicated that the chain architecture and composition of triblock copolymers as well as the concentration of the surfactant would have significant impacts on the shape and internal phase structures of triblock copolymer particles formed via 3D-SCSA.
Author contributions
Lei Guo: conceptualization, methodology, data curation, formal analysis, investigation, writing – original draft, preparation, and writing – review & editing. Dingli Xia: methodology and formal analysis. Yaping Wang: methodology and formal analysis. Shipeng Ding: writing – review & editing. Junting Xu: resources and writing – review & editing. Yutian Zhu: resources and writing – review & editing. Binyang Du: conceptualization, methodology, resources, funding acquisition, supervision, project administration, and writing – review & editing.
Conflicts of interest
The authors declare that they have no known competing financial interests or personal relationships that could have appeared to influence the work reported in this paper.
Acknowledgements
The authors thank the “Pioneer” and “Leading Goose” R&D Program of Zhejiang (no. 2022C01109) for financial support. The authors also thank the Analysis Center of Agrobiology and Environmental Sciences, Chemistry Department Analysis Test Platform, Zhejiang University for technical assistance with SEM and TEM measurement and the beamline BL16B1 at the SSRF for providing the beam time.
References
- J. D. Forster, J.-G. Park, M. Mittal, H. Noh, C. F. Schreck, C. S. O'Hern, H. Cao, E. M. Furst and E. R. Dufresne, ACS Nano, 2011, 5, 6695–6700 CrossRef CAS PubMed.
- Y. Yang, J. B. Kim, S. K. Nam, M. Zhang, J. Xu, J. Zhu and S. H. Kim, Nat. Commun., 2023, 14, 793 CrossRef CAS.
- M. Iqbal, N. Zafar, H. Fessi and A. Elaissari, Int. J. Pharm., 2015, 496, 173–190 CrossRef CAS PubMed.
- Q. L. Yu, N. Sun, D. W. Hu, Y. P. Wang, X. H. Chang, N. Yan, Y. T. Zhu and Y. J. Li, Polym. Chem., 2021, 12, 4184–4192 RSC.
- N. Yonet-Tanyeri, M. Amer, S. C. Balmert, E. Korkmaz, L. D. Falo and S. R. Little, ACS Biomater. Sci. Eng., 2022, 8, 2864–2877 CrossRef CAS PubMed.
- M. P. van der Helm, C. L. Wang, B. W. Fan, M. Macchione, E. Mendes and R. Eelkema, Angew. Chem., Int. Ed., 2020, 59, 20604–20611 CrossRef CAS.
- Z. H. Lu, G. J. Liu, H. Phillips, J. M. Hill, J. Chang and R. A. Kydd, Nano Lett., 2001, 1, 683–687 CrossRef CAS.
- A. Setaro, S. Lettieri, P. Maddalena and L. De Stefano, Appl. Phys. Lett., 2007, 91, 051921 CrossRef.
- Y. Y. Mai and A. Eisenberg, Chem. Soc. Rev., 2012, 41, 5969–5985 RSC.
- U. Tritschler, S. Pearce, J. Gwyther, G. R. Whittell and I. Manners, Macromolecules, 2017, 50, 3439–3463 CrossRef CAS.
- D. Lee, J. Kim, K. H. Ku, S. Li, J. J. Shin and B. J. Kim, Polym. Chem., 2022, 13, 2570–2588 RSC.
- N. Yan, Y. T. Zhu and W. Jiang, Chem. Commun., 2018, 54, 13183–13195 RSC.
- D. W. Hu, X. H. Chang and Y. T. Zhu, Chin. J. Chem., 2023, 41, 237–245 CrossRef CAS.
- K. H. Ku, J. M. Shin, H. Yun, G. R. Yi, S. G. Jang and B. J. Kim, Adv. Funct. Mater., 2018, 28, 1802961 CrossRef.
- I. Wyman, G. Njikang and G. J. Liu, Prog. Polym. Sci., 2011, 36, 1152–1183 CrossRef CAS.
- J. J. Shin, E. J. Kim, K. H. Ku, Y. J. Lee, C. J. Hawker and B. J. Kim, ACS Macro Lett., 2020, 9, 306–317 CrossRef CAS.
- R. H. Deng, F. X. Liang, W. K. Li, Z. Z. Yang and J. T. Zhu, Macromolecules, 2013, 46, 7012–7017 CrossRef CAS.
- J. Lee, K. H. Ku, M. Kim, J. M. Shin, J. Han, C. H. Park, G. R. Yi, S. G. Jang and B. J. Kim, Adv. Mater., 2017, 29, 1700608 CrossRef PubMed.
- K. H. Ku, J. M. Shin, M. P. Kim, C. H. Lee, M. K. Seo, G. R. Yi, S. G. Jang and B. J. Kim, J. Am. Chem. Soc., 2014, 136, 9982–9989 CrossRef CAS PubMed.
- J. Lee, K. H. Ku, C. H. Park, Y. J. Lee, H. Yun and B. J. Kim, ACS Nano, 2019, 13, 4230–4237 CrossRef CAS.
- K. H. Ku, Y. Kim, G. R. Yi, Y. S. Jung and B. J. Kim, ACS Nano, 2015, 9, 11333–11341 CrossRef CAS PubMed.
- Y. P. Wang, D. W. Hu, X. H. Chang and Y. T. Zhu, Macromolecules, 2022, 55, 6211–6219 CrossRef CAS.
- J. P. Xu, Y. Yang, K. Wang, Y. Q. Wu and J. T. Zhu, Mater. Chem. Front., 2017, 1, 507–511 RSC.
- J. M. Shin, M. P. Kim, H. Yang, K. H. Ku, S. G. Jang, K. H. Youm, G. R. Yi and B. J. Kim, Chem. Mater., 2015, 27, 6314–6321 CrossRef CAS.
- D. Klinger, C. X. Wang, L. A. Connal, D. J. Audus, S. G. Jang, S. Kraemer, K. L. Killops, G. H. Fredrickson, E. J. Kramer and C. J. Hawker, Angew. Chem., Int. Ed., 2014, 53, 7018–7022 CrossRef CAS.
- R. H. Deng, L. F. Zheng, X. Mao, B. H. Li and J. T. Zhu, Small, 2021, 17, 2006132 CrossRef CAS.
- J. M. Shin, Y. Kim, H. Yun, G. R. Yi and B. J. Kim, ACS Nano, 2017, 11, 2133–2142 CrossRef CAS.
- Y. L. Liu, F. F. Ke, Y. C. Li, Y. Shi, Z. Zhang and Y. M. Chen, Nano Res., 2023, 16, 564–582 CrossRef.
- M. L. Peng, D. W. Hu, X. H. Chang and Y. T. Zhu, J. Phys. Chem. B, 2022, 126, 9435–9442 CrossRef CAS PubMed.
- J. P. Xu and J. T. Zhu, Chin. J. Polym. Sci., 2019, 37, 744–759 CrossRef CAS.
- J. Lee, K. H. Ku, J. Kim, Y. J. Lee, S. G. Jang and B. J. Kim, J. Am. Chem. Soc., 2019, 141, 15348–15355 CrossRef CAS.
- D. W. Hu, X. H. Chang, Y. Q. Xu, Q. L. Yu and Y. T. Zhu, ACS Macro Lett., 2021, 10, 914–920 CrossRef CAS.
- J. Kim, Y. J. Lee, K. H. Ku and B. J. Kim, Macromolecules, 2022, 55, 8355–8364 CrossRef CAS.
- F. M. Zhao, Z. W. Xu and W. H. Li, Macromolecules, 2021, 54, 11351–11359 CrossRef CAS.
- S. G. Jang, D. J. Audus, D. Klinger, D. V. Krogstad, B. J. Kim, A. Cameron, S. W. Kim, K. T. Delaney, S. M. Hur, K. L. Killops, G. H. Fredrickson, E. J. Kramer and C. J. Hawker, J. Am. Chem. Soc., 2013, 135, 6649–6657 CrossRef CAS.
- R. H. Deng, H. Li, F. X. Liang, J. T. Zhu, B. H. Li, X. L. Xie and Z. Z. Yang, Macromolecules, 2015, 48, 5855–5860 CrossRef CAS.
- M. Tromer, E. M. Zirdehi, A. Nikoubashman and A. H. Groschel, Macromol. Rapid Commun., 2023, 44, 2300123 CrossRef CAS.
- J. P. Xu, K. Wang, J. Y. Li, H. M. Zhou, X. L. Xie and J. T. Zhu, Macromolecules, 2015, 48, 2628–2636 CrossRef CAS.
- X. L. Qiang, X. Z. Dai, A. Steinhaus and A. H. Groschel, ACS Macro Lett., 2019, 8, 1654–1659 CrossRef CAS.
- A. Steinhaus, T. Pelras, R. Chakroun, A. H. Groschel and M. Mullner, Macromol. Rapid Commun., 2018, 39, 1800177 CrossRef.
- S. M. Mai, W. Mingvanish, S. C. Turner, C. Chaibundit, J. P. A. Fairclough, F. Heatley, M. W. Matsen, A. J. Ryan and C. Booth, Macromolecules, 2000, 33, 5124–5130 CrossRef CAS.
- M. W. Matsen and R. B. Thompson, J. Chem. Phys., 1999, 111, 7139–7146 CrossRef CAS.
- F. S. Bates, M. A. Hillmyer, T. P. Lodge, C. M. Bates, K. T. Delaney and G. H. Fredrickson, Science, 2012, 336, 434–440 CrossRef CAS.
- L. Guo, J. T. Xu and B. Y. Du, Polym. Rev., 2023 DOI:10.1080/15583724.2023.2178008 DOI:10.1080/15583724.15582023.12178008.
- A. Horvat, K. S. Lyakhova, G. J. A. Sevink, A. V. Zvelindovsky and R. Magerle, J. Chem. Phys., 2004, 120, 1117–1126 CrossRef CAS PubMed.
- Y. P. Sheng, J. An and Y. T. Zhu, Chem. Phys., 2015, 452, 46–52 CrossRef CAS.
- B. Yu, P. C. Sun, T. H. Chen, Q. H. Jin, D. T. Ding, B. H. Li and A. C. Shi, Phys. Rev. Lett., 2006, 96, 138306 CrossRef PubMed.
- J. T. Lai, D. Filla and R. Shea, Macromolecules, 2002, 35, 6754–6756 CrossRef CAS.
- J. G. Kennemur, Macromolecules, 2019, 52, 1354–1370 CrossRef CAS.
- Z. W. Xu and W. H. Li, Chin. J. Chem., 2022, 40, 1083–1090 CrossRef CAS.
- M. P. Kim, D. J. Kang, D. W. Jung, A. G. Kannan, K. H. Kim, K. H. Ku, S. G. Jang, W. S. Chae, G. R. Yi and B. J. Kim, ACS Nano, 2012, 6, 2750–2757 CrossRef CAS PubMed.
- Z. J. Huo, S. Arora, V. A. Kong, B. J. Myrga, A. Statt and J. E. Laaser, Macromolecules, 2023, 56, 1845–1854 CrossRef CAS PubMed.
- H. Schmalz, A. Boker, R. Lange, G. Krausch and V. Abetz, Macromolecules, 2001, 34, 8720–8729 CrossRef CAS.
- D. Cai, J. B. Li, Z. Ma, Z. H. Gan, Y. Shao, Q. Xing, R. Tan and X. H. Dong, ACS Macro Lett., 2022, 11, 555–561 CrossRef CAS PubMed.
|
This journal is © The Royal Society of Chemistry 2024 |
Click here to see how this site uses Cookies. View our privacy policy here.