DOI:
10.1039/D4RA00091A
(Paper)
RSC Adv., 2024,
14, 6253-6261
Improved synthesis of antiplasmodial 4-aminoacridines and 4,9-diaminoacridines†
Received
4th January 2024
, Accepted 7th February 2024
First published on 19th February 2024
Abstract
Acridines are one of the most important nitrogen-containing heterocycle systems and have many applications in the therapeutic field. However, the synthesis of acridine-based scaffolds is not always straightforward. Herein, we report the optimization of two multi-step synthetic routes towards 4,9-diaminoacridines and 4-aminoacridines, which have shown promising antiplasmodial properties. The improved synthesis pathways make use of greener, simpler, and more efficient methods, with less reaction steps and increased overall yields, which were doubled in some cases. These are impactful results towards future approaches to the chemical synthesis of acridine-based compounds.
Introduction
Acridine (Fig. 1) was initially extracted by Grabe and Caro from the anthracene fraction of coal tar in 1870.1,2 Due to its unique physical and chemical properties, biological activities, and industrial applications, it represents one of the most important nitrogen-containing heterocycle systems. Acridines were first used as dyes in fabrics, but their fluorescence properties soon after triggered their wide application in several cellular investigations such as cell cycle determination, nucleic acid staining, and flow cytometry. The therapeutic potential of aminoacridines, owing to their antimicrobial properties, was recognized as soon as in 1912 and underpinned the use of acridines as antiseptics during World War I. The therapeutic relevance of acridine-based compounds was further consolidated in World War II, when mepacrine, a 9-aminoacridine, was widely employed as an antimalarial drug to compensate for the ongoing chloroquine shortage. Since then, both mepacrine and other acridine derivatives have been reported with antitumoral, antibacterial, anthelmintic, antiviral, antifungal, antitubercular, and antileishmanial activities, among other potential therapeutic uses, including for neurodegenerative diseases.1–6
 |
| Fig. 1 Acridine structure and its numbering as defined by the International Union of Pure and Applied Chemistry (IUPAC). | |
There is no general method for the synthesis of acridine derivatives. Direct functionalization of the acridine ring is a most interesting method, since it reduces the number of synthetic steps required, facilitating the synthesis of complex polycyclic structures. However, these approaches have a limited range of applications, as classic electrophilic substitutions on the acridine ring are usually not regioselective and produce many polyfunctionalized products. In this sense, the most widely applied syntheses of acridines involve, as a first step, ring closure through the condensation of adequately functionalized anilines and o-halobenzoic acids. The Bernthsen reaction was one of the earliest methods to obtain the acridine core and consists in mixing an aromatic or aliphatic carboxylic acid with a diphenylamine in the presence of zinc chloride and heating at high temperature (200–270 °C) for several hours.7 Due to these harsh conditions and to the limited variety of substrates available, this method was quickly superseded by copper- or palladium-catalyzed methods, namely, Ulmann and Buchwald–Hartwig cross-coupling reactions, respectively, followed by dehydrogenation.1,6,8,9 The acridine core obtained via the aforementioned methods is a versatile scaffold that can undergo many further modifications, mainly in the C-9 and N-10 positions, which are the most reactive sites due to the presence of the pyridinic nitrogen in the heterocycle. The reactivity of these positions is obviously modulated by the electron-donating or withdrawing properties of the substituents on the phenyl rings of the acridine scaffold.6,10,11 The N-10 position is the main nucleophilic site of the acridine core, in which N-alkylations can easily occur, with the formation of acridinium salts under strongly acidic conditions. On the other hand, due to the strong electron deficiency caused by the pyridinic nitrogen on the C-9 position, this is the most electrophilic site of the acridine system, allowing for nucleophilic reactions to take place there.10 For these reasons, most of the acridine-based compounds developed so far display chemical modifications in those two positions,12,13 while modifications elsewhere in the acridine ring remain limited.13
Based on the well-known therapeutic potential of aminoacridines,14–17 our research group recently developed two unprecedented synthesis routes towards 4,9-diaminoacridines and 4-aminoacridines (Scheme 1).15,16 These routes comprise two main parts: (a) production of the 6,9-dichloro-2-methoxy-4-nitroacridine precursor common to both 4,9-diaminoacridine and 4-aminoacridine target families (Scheme 1, part A), and (b) further modifications in C-9 and/or C-4 positions of the acridine ring (Scheme 1, part B).15,16 Both are extensive and laborious synthetic routes that involve several steps featuring harsh reactional conditions and low yields. Based on this, and further motivated by the fact that these families of aminoacridines have shown interesting antiplasmodial properties,16 we have now investigated whether simpler, more selective, and less time-consuming methods could be used to synthesize the same target aminoacridines in fewer reactional steps and with improved yields. Successful improvement of the synthesis routes was achieved, as herein reported.
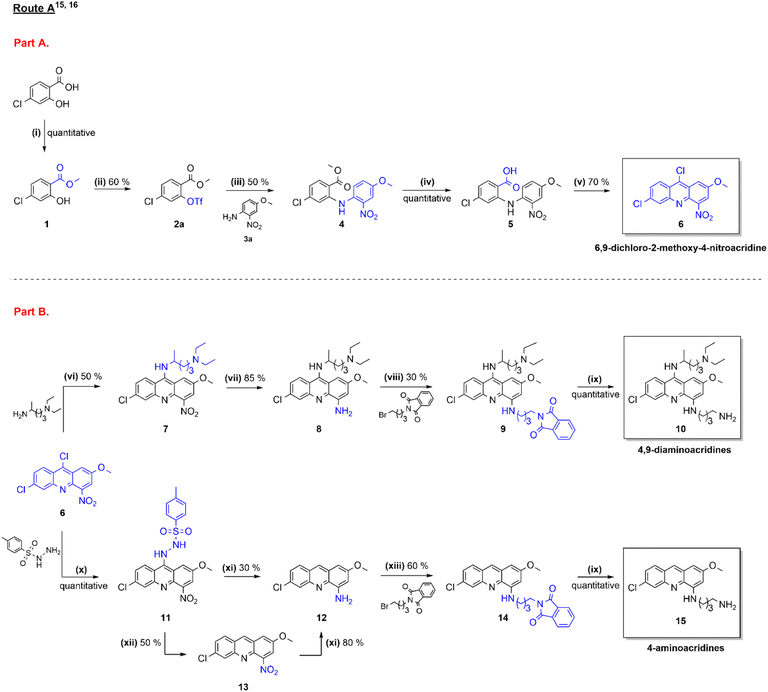 |
| Scheme 1 Synthetic route A towards 6,9-dichloro-2-methoxy-4-nitroacridine (6), 4,9-diaminoacridines (10) and 4-aminoacridines (15), as previously reported by Fonte M. et al.15,16 Reagents and conditions: (i) CH3I (5 molar equivalents, equiv.), Cs2CO3 (0.5 equiv.), dimethylformamide (DMF), room temperature (rt), 1.5 h; (ii) Tf2O (1.5 equiv.), Et3N (2 equiv.), CH2Cl2, N2 atmosphere, −25 °C, 30 min; (iii) 4-methoxy-2-nitroaniline (3a, 1.2 equiv.), Cs2CO3 (1.4 equiv.), Pd(OAc)2 (0.05 equiv.), rac-BINAP (0.08 equiv.), toluene, N2 atmosphere, 120 °C, 5 h; (iv) (1) Ba(OH)2·8H2O (1.5 equiv.), CH3OH, 90 °C, 2 h; (2) 1 M aq. HCl; (v) POCl3 (34 equiv.), 120 °C, 2.5 h; (vi) (1) phenol (15 equiv.), Cs2CO3 (1 equiv.), anhydrous dimethyl sulfoxide (DMSO), 4 Å molecular sieves, 100 °C, 2 h; (2) N′,N′-diethylpentan-1,4-diamine (4 equiv.), 100 °C, 4 h; (vii) SnCl2 (5 equiv.), 37% aq. HCl, 0 → 40 °C, 30 min; (viii) N-(4-bromobutyl)phthalimide (3 equiv.), CH3COONa (3 equiv.), CH3CH2OH, microwave (MW) heating (100 W, 120 °C) in a pressurized vial (100 psi), 2.5 h; (ix) hydrazine monohydrate (40 equiv.), tetrahydrofuran (THF), 80 °C, 24–48 h; (x) p-toluenesulfonyl hydrazide (p-TSH, 1 equiv.), CHCl3, rt, 24–48 h; (xi) NH2NH2 (10 equiv.), Pd/C (10% wt), CH3OH, 80 °C, 1–3 h; (xii) HOCH2CH2OH/H2O (2 : 1), Na2CO3 (0.0625 M), 95 °C, 1.5 h; (xiii) N-(4-bromobutyl)phthalimide (3 equiv.), Et3N (3 equiv.), CH3CH2OH, MW heating (100 W, 120 °C) in a pressurized vial (100 psi), 3 h. | |
Results and discussion
Optimization of the synthesis route to 6,9-dichloro-2-methoxy-4-nitroacridine (6)
6,9-Dichloro-2-methoxy-4-nitroacridine (6) is the main synthetic precursor of acridines modified in the C-4 and C-9 positions. We have previously achieved the synthesis of 6, starting from commercial 4-chlorosalicylic acid that was first protected by conversion into its methyl ester (1, Scheme 1, step i), then activated by conversion of the alcohol into a triflate (2a, Scheme 1, step ii), which was next reacted with 4-methoxy-2-nitroaniline (3a) via the Buchwald–Hartwig coupling reaction (Scheme 1, step iii). The product obtained (4) was next submitted to basic hydrolysis (Scheme 1, step iv) to afford the carboxylic acid 5, and this was then treated POCl3 (Scheme 1, step v) to promote the desired cyclization into 6,9-dichloro-2-methoxy-4-nitroacridine 6 (Scheme 1, part A).15 This synthetic route was adapted from the literature,18 and was the only one that, in our hands, successfully led to the target precursor 6, which we recurrently failed to produce by means of Ulmann-type reactions.15 Still, this synthetic route to 6 posed a few challenges, mainly due to the strong electron-withdrawing effect of the nitro group that significantly decreased the reactivity of the aniline 3a in the Buchwald–Hartwig coupling. While this was compensated by use of an excellent leaving group such as triflate, triflate 2a is not commercially available, which added three more steps to the synthesis route (Scheme 1, steps i, ii and iv).
In face of the above, we decided to work on the optimization of the synthesis route to 6. Therefore, we opted to take advantage of the properties of the nitro group, and replaced 2a and 3a (Table 1, entry 1) by reagents 2c and 3b (Table 1, entry 2). In this case, the nucleophilicity of 2c is not affected by the nitro group that, in turn, makes the bromine in ortho position of 3b very electrophilic, which favors the cross-coupling reaction. This led to successful synthesis of 5 in moderate yield (Table 1, entry 2; η = 45%) and without the need for steps i, ii and iv shown in Scheme 1. Additionally, the increase of the reaction time from 5 h to 24 h considerably improved the yield (Table 1, entry 3a; η = 70%). Despite this clear improvement, the low solubility of 5 makes scale-up challenging, especially concerning its isolation by column chromatography on silica, which reduces the overall yield to below 50% (Table 1, entry 3b). An attempt to overcome this obstacle, by carrying out both the cross-coupling and cyclization steps in a one-pot procedure, avoiding the purification step, was not successful. In view of this, we next tested replacement of the carboxylic acid 2c by the corresponding methyl ester 2b; this allowed production and isolation of 4 in very good overall yield (75%), without any purification issues (Scheme 2, step iii and Table 1, entry 4). Relevantly, the yield was significantly improved by not using any excess of 2b (Table 1, entry 5).
Table 1 Optimization of step iii (Scheme 1, part A) in the synthesis route to 6,9-dichloro-2-methoxy-4-nitroacridine 6
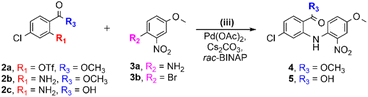
|
Entry |
Amine (equiv.) |
Halogen (equiv.) |
Product |
Time/h, T/°C |
Yield/% |
Synthesis at the 100 mg scale.
Synthesis at the 1.5 g scale.
|
1 |
3a (1.2) |
2a (1.0) |
4
|
5, 120 |
50b |
2 |
2c (1.2) |
3b (1.0) |
5
|
5, 120 |
45a |
3 |
2c (1.2) |
3b (1.0) |
5
|
24, 120 |
70a (<50)b |
4 |
2b (1.2) |
3b (1.0) |
4
|
1, 120 |
75b |
5 |
2b (1.0) |
3b (1.0) |
4
|
2, 120 |
90b |
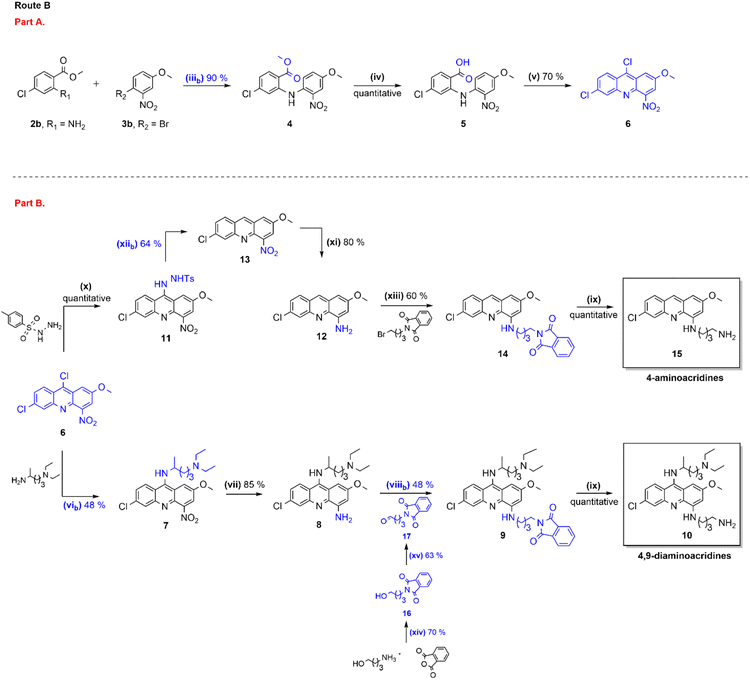 |
| Scheme 2 Synthetic route B towards 6,9-dichloro-2-methoxy-4-nitroacridine (6), 4,9-diaminoacridines (10) and 4-aminoacridines (15). Reagents and conditions: (iiib) 2b (1 equiv.), 3b (1 equiv.), Cs2CO3 (1.4 equiv.), Pd(OAc)2 (0.05 equiv.), rac-BINAP (1.8 equiv.), toluene, 120 °C, 2 h; (iv) (1) Ba(OH)2·8H2O (1.5 equiv.), CH3OH, 90 °C, 2 h; (2) 1 M aq. HCl; (v) POCl3 (34 equiv.), 120 °C, 2.5 h. (vib) Phenol (15 equiv.), Cs2CO3 (1 equiv.), N′,N′-diethylpentan-1,4-diamine (4 equiv.), anhydrous DMSO, MW heating (100 W, 100 °C) in a pressurized vial (100 psi), 40 min; (vii) SnCl2 (5 equiv.), 37% aq. HCl, 0 °C → 40 °C, 0.5 h; (viiib) 17 (1.1 equiv.), NaBH(OAc)3 (2 equiv.), 1,2-dichloroethane (DCE), rt, 4 h or 72 h; (ix) hydrazine monohydrate (40 equiv.), THF, 80 °C, 24–48 h; (x) p-TSH (1 equiv.), CHCl3, rt, 24 h; (xi) NH2NH2 (10 equiv.), Pd/C (10% wt), CH3OH, 80 °C, 1–3 h; (xiib) NaBH4 (10 equiv.), CH3OH, 80 °C, 24 h; (xiii) N-(4-bromobutyl)phthalimide (3 equiv.), Et3N (3 equiv.), CH3CH2OH, MW heating (100 W, 120 °C) in a pressurized vial (100 psi), 3 h; (xiv) phthalic anhydride (1 equiv.), 4-aminobutan-1-ol (1 equiv.), dioxane, 100 °C, overnight; (xv) 16 (1 equiv.), Dess–Martin periodinane (DMP, 1.1 equiv.), CH2Cl2, rt, 24 h. | |
The optimization efforts above targeted production of 6, which bears a chlorine substituent in C-9. Yet, production of the acridine derivative devoid of chlorine in this position is also of great interest for the synthesis of the 4-aminoacridines 15, as it will turn the two reduction steps in Scheme 1 (step x and xii) unnecessary. As such, we addressed the partial reduction of ester 4 into the corresponding aldehyde, which after cyclization using a strong acid like trifluoracetic acid could directly afford compound 13. Therefore, we adopted the methodology reported by Na and co-workers,19,20 which makes use of a DIBALH/morpholine system to produce a morpholine amide intermediate that, in the presence of an hydride source, leads to aldehyde formation in mild conditions, short reaction times (<60 min), and almost quantitative yields. Unfortunately, this approach was not successful in our case. Likewise, the reduction of ester 4 to the corresponding alcohol using a sodium borohydride–methanol system, followed by oxidation to the aldehyde,21–23 equally failed.
One last optimization effort for the synthesis of 6 regarded the cyclization by dehydrogenation (Scheme 1, step v). Replacement of POCl3 by POBr3 to produce the Br-substituted acridine in C-9 was attempted, given the fact that bromine is a better leaving group than chlorine which could improve the ensuing aromatic substitutions (Scheme 1, steps vi and x). Unfortunately, these conditions led to a complex mixture of products, amongst which the desired one could not be detected by mass spectrometry (MS) or thin layer chromatography (TLC).
Overall, and regardless of obstacles found, the synthesis route to 6 depicted in Scheme 2 allowed to produce the target compound, 6,9-dichloro-2-methoxy-4-nitroacridine, in only three steps and with an overall yield of 63% (Scheme 2, part B). This is clearly a significant improvement over the previously reported route, where 6 was produced in 5 steps (Scheme 1, part A) with an overall 21% yield.15,16
Optimization of the synthesis route to 4,9-diaminoacridines (10)
The chemical synthesis of 4,9-diaminoacridines 10 had been previously achieved via four reaction steps (Scheme 1, steps vi–ix),15 starting with a nucleophilic aromatic substitution (SNAr) on 6 using N′,N′-diethylpentan-1,4-diamine; this was a time-consuming (6 h) procedure comprising activation of 6 by formation of an aryl ether intermediate at C-9, and the subsequent nucleophilic attack by the amine to this intermediate (Scheme 1, step vi). The rate and regioselectivity of this reaction is strongly dependent on acridine substituents, and the presence of the nitro group in the C-4 position of 6 significantly increases its reactivity, which results in the formation of multiple by-products.10,11 For this reason, our first optimization attempt consisted in first reducing 6 into 6,9-dichloro-2-methoxy-4-aminoacridine, by use of SnCl2, and then perform the SNAr reaction. However, 6,9-dichloro-2-methoxy-4-aminoacridine failed to undergo the desired SNAr, probably due to the electron-donating effect of the amine group in C-4. Therefore, we alternatively focused our optimization efforts on the steps carried out under microwave (MW) heating, as this has very well-known advantages, such as reducing the reaction times, improving yields and purity of the target compounds, and overall simplifying work-up procedures.24 As such, we adapted the procedure reported by Staderini and co-workers,25 to produce compound 7 using MW microwave heating at 100 °C (Scheme 2, step vib); this reaction showed to be quite time-dependent, since an increase of reaction time from 40 minutes to 1 h about halved the yield from 46% to 24%. Relevantly, conventional heating led to similar yields, but use of MW heating notably reduced the reaction time from 6 h to 40 minutes, and simplified the overall procedure and work-up, by obviating the need for an initial phenol-activation step. We next addressed optimization of the introduction of the aminoalkyl side chain in the C-4 position of the acridine ring of aniline 8; we had previously started by reducing the nitro group in 7 with SnCl2/HCl, to produce 8 in 85% yield (Scheme 1, step vii), and next alkylated 8 with N-(4-bromobutyl)phthalimide using CH3COONa as base (Scheme 1, step viii and Table 2, entry 1). However, this approach required high temperature (120 °C) and excess of the alkylating agent (3 equiv.), which allowed the formation of the di-alkylated product. As such, we started by testing whether reducing the amount of alkylating agent from 3 to 1 molar equivalents could reduce the amount of di-alkylated product and consequently increase the yield of the desired mono-alkylated product, 9. However, although this change did decrease the extension of the side-reaction, it also lowered the yield of formation of 9 from 30% to 10% (Table 2, entry 2). Alternatively, compound 9 was synthesized via reductive amination of aldehyde 17, which was first produced by reaction of phthalic anhydride with 4-aminobutan-1-ol (Scheme 2, step xiv; η = 70%) followed by selective oxidation with Dess–Martin periodinane (DMP) (Scheme 2, step xv; η = 63%).26 This method, which uses milder conditions and avoids di-alkylated by-products, successfully afforded 9 in 48% yield, thus offering a significant improvement over our initial approach (Table 2, entry 3 and Scheme 2, step viiib). The increase of reaction time and of the amount of aldehyde to 24 h and 3 molar equivalents, respectively, favored formation of by-products with a consequent decrease in the reaction yield to 10% (Table 2, entry 4).
Table 2 Optimization of step viii (Scheme 1, part B) in the synthesis route to 4,9-diaminoacridines 10
Optimization of the synthesis route to 4-aminoacridines (15)
Our initial synthesis route to 4-aminoacridines 15 comprised five reactional steps starting from 6, in which reduction of the chlorine and nitro groups in C-9 and C-4, respectively, led to the 4-aminoacridine 12 that was next N-alkylated to afford the N-phthalimide 14, which was finally converted into 15 by removal of the phthaloyl N-protecting group (Scheme 1, steps x–ix).16 Our efforts to improve the synthesis of the target 4-aminoacridines 15, which are summarized in Table 3, first focused on the reduction steps. Initial attempts involved the selective remotion of the chlorine in C-9 by reduction with H2/Pd–C or with Et3SiH/Pd(PPh3)2Cl2; these attempts led to the loss the two chlorines in the acridine systems (Table 3, entry 1 and 2). In view of this, we redirected our focus to the reduction of intermediate 11 that is produced by reacting 6 with p-toluenesulfonyl hydrazide (p-TSH). The cleavage of the p-TSH group and the reduction of the nitro group can be simultaneously achieved by treatment with hydrazine (Table 3, entry 3) or SnCl2 (Table 3, entry 4). Alternatively, a two-step procedure can be employed using Na2CO3 in aqueous ethylene glycol (Table 3, entry 5) followed by reduction with hydrazine (Table 3, entry 7).16 As a way of enhancing the yield, we next tested the stepwise reduction of 11 into 13, by use of NaBH4 in refluxing CH3OH (Scheme 2, step xiib and Table 3, entry 6), which afforded the desired compound in 65% yield, higher than that previously achieved (50%). Encouraged by this result, we next tried once more to improve the conditions for simultaneous reduction of the two groups of interest, i.e., chlorine in C-9 and nitro in C-4. To this end, different combinations of NaBH4 with transition-metal salts, such as NiCl2·6H2O, CuBr2, Fe(OTf)3, Cu(NO3)2·3H2O, CuSO4/CoCl2, Ni(OAc)2·4H2O, Cu(acac)2, SnCl2·2H2O, have been reported in the literature as being capable of reducing nitro groups as well.27–31 Therefore, we tested the use of NaBH4/Cu(NO3)2·3H2O, NaBH4/CuBr2 and NaBH4/SnCl2·2H2O combinations, but these failed to promote reduction of the nitro group, thus affording 13 (Table 3, entries 8, 9 and 10). Another three one-pot reactions were attempted whereby other combinations of reducing agents were used, namely, Na2CO3 in aqueous ethylene glycol followed by hydrazine hydrate (Table 3, entry 11; η = 6%); Na2CO3 in aqueous ethylene glycol followed by SnCl2/HCl (Table 3, entry 12; η = 13%); and NaBH4 followed by hydrazine hydrate (Table 3, entry 13; η = 15%). Again, none of these attempts gave better yields than the previous methods for concomitant reduction of both groups, i.e., direct conversion of 11 into 12. As one last attempt to improve production of aminoacridine 12, SnCl2 was used to reduce the nitro group (Scheme 2, step xib and Table 3, entry 14), similarly to what had been carried out in the synthesis route to 4,9-diaminoacridines 10. However, results were not so good in this case, since compound 12 was obtained in only 27% yield; mass spectrometry analysis (data not shown) revealed that reduction of the nitro group into the corresponding aniline was accompanied by the entry of a chlorine atom in C-9. Altogether, this optimization effort enabled us to conclude that the best option to produce 12 is by a two-step procedure where the p-TSH group in 11 is first removed by reduction with NaBH4 followed by reduction of the nitro group with hydrazine, which allows an improvement of the overall yield from 40% to 52% (Scheme 2, steps xi and xiib).
Table 3 Optimization of steps x–xii (Scheme 1, part B) in the synthesis route to 4-aminoacridines 15
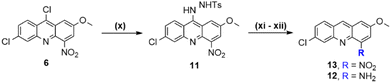
|
Entry |
Starting reagent |
Product |
Reducing agent (equiv.) |
Catalyst |
T/°C, time/h |
Solvent |
Yield/% |
Reaction conditions: H2 (50 psi).
NaBH4, Cu(NO3)2·3H2O, CH3OH, 80 °C, 24 h.
NaBH4, CuBr2, CH3OH, 80 °C, 28 h.
NaBH4, CH3OH, 80 °C, 24 h, followed by addition of SnCl2·2H2O, 4 h.
Na2CO3 (0.0625 M), HOCH2CH2OH/H2O (2 : 1), 95 °C, 1.5 h, followed by addition of NH2NH2 and Pd–C, 80 °C, 1 h.
Na2CO3 (0.0625 M), HOCH2CH2OH/H2O (2 : 1), 95 °C, 1.5 h, filtration of the black precipitate followed by its dissolution in aqueous HCl 37% → anhydrous SnCl2, 40 °C, 0.5 h.
NaBH4, CH3OH, 80 °C, 24 h, followed by addition of NH2NH2 and Pd–C, 80 °C, 1 h.
One-pot reaction; without isolation of intermediate 13.
|
1a |
6
|
13
|
H2 |
Pd–C (10 wt%) |
rt, 5 |
CH3OH |
— |
2 |
6
|
13
|
Et3Si (1.4) |
Pd(PPh3)2Cl2 (1 mol%) |
70, 3 |
CH3CN |
— |
3 |
11
|
12
|
NH2NH2 (10) |
Pd–C (10 wt%) |
80, 5 |
CH3OH |
30 |
4 |
11
|
12
|
SnCl2 (3.0) |
— |
40, 0.5 |
Aq. HCl 37% |
18 |
5 |
11
|
13
|
Na2CO3 |
— |
95, 1.5 |
HOCH2CH2OH/H2O (2 : 1) |
50 |
6 |
11
|
13
|
NaBH4 (20) |
— |
80, 24 |
CH3OH |
65 |
7 |
13
|
12
|
NH2NH2 (10) |
Pd–C (10 wt%) |
80, 1 |
CH3OH |
80 |
8b |
11
|
12
|
NaBH4 (3.0) |
Cu(NO3)2·3H2O (15 mol%) |
80, 24 |
CH3OH |
— |
9c |
11
|
12
|
NaBH4 (10) |
CuBr2 (10 mol%) |
80, 28 |
CH3OH |
— |
10d |
11
|
12
|
(1) NaBH4 (10) |
— |
80, 28 |
CH3OH |
— |
(2) SnCl2·2H2O (10) |
11e,h |
11
|
12
|
(1) Na2CO3 |
Pd–C (10 wt%) |
(1) 95, 1.5 |
HOCH2CH2OH/H2O (2 : 1) |
6 |
(2) NH2NH2 (10) |
(2) 80, 1 |
12f,h |
11
|
12
|
(1) Na2CO3 |
— |
(1) 95, 1.5 |
(1) HOCH2CH2OH/H2O (2 : 1) |
13 |
(2) SnCl2 (3.0) |
(2) 40, 0.5 |
(2) HCl |
13g,h |
11
|
12
|
(1) NaBH4 (20) |
Pd–C (10 wt%) |
80, 25 |
CH3OH |
15 |
(2) NH2NH2 (10) |
14 |
13
|
12
|
SnCl2 (3) |
— |
40, 0.5 |
Aq. HCl 37% |
27 |
Finally, we addressed the improvement of the alkylation of 12 with N-(4-bromobutyl)phthalimide to produce 14 (Scheme 1, step xiii). Based on the good results obtained in the optimized synthesis route to 4,9-diaminoacridines 10, we applied reductive amination to obtain 14. However, a significant amount of 12 remained unreacted even after 72 h, probably due to the lowered reactivity of its aniline group, as compared to that in compound 8, its counterpart in the synthesis of 4,9-diaminoacridines 10. This translated into a 30% yield, lower than the 60% achieved via bimolecular nucleophilic substitution in the presence of triethylamine (Scheme 1, step xiii). As a very last effort, we still tested the use of acetic acid as catalyst under MW heating,32 but these conditions failed to deliver the desired product.
The highest scale attempt for the synthesis of 4-aminoacridine (15) was about 2 g, i.e., starting from approximately 1.8 g of 6,9-dichloroaminoacridine (6). Under these conditions, we were able to obtain 0.5 g of 4-aminoacridine (15). No further scale-up attempts have been performed, but we trust that at least a doubled scale (starting from 4 g) is feasible without significantly affecting the yield.
Experimental section
Synthetic procedures§
All chemicals were purchased from Sigma-Aldrich, Abcr, Fluorochem, Fluka, Biochem, Chemopharma, Alfa Aesar, Merck or PanReac AppliChem and used without further purification. The solvents were all of p.a. quality and purchased from VWR International, Carlo Erba, LabChem or Honeywell Riedel-de-Haën. When needed, anhydrous solvents were purchased as such, i.e., not requiring any additional treatment (apart from the use of inert reaction conditions that are identified whenever applicable). Information about the specific brand of each reagent and solvent used is given in detail in the ESI.† Similarly, detailed information about the type of equipments used (such as NMR and mass spectrometers, rotary evaporator, microwave, etc.) is included in the ESI.†
Route A¶.
Compounds 1–15 were prepared following the procedures previously described, and their structural analyses agreed with formerly reported data.15,16
Route B.
Synthesis of compound 4 (step iiib).
In a 250 mL glass round-bottom flask charged with a magnetic stir bar, 4-bromo-3-nitroanisole (1 equiv., 4.36 mmol, 1.0117 g) was dissolved in toluene (50 mL). Then, Cs2CO3 (1.4 equiv., 6.10 mmol, 1.9875 g), Pd(OAc)2 (0.05 equiv., 0.22 mmol, 0.0494 g) and rac-BINAP (0.08 equiv., 0.35 mmol, 0.2179 g) were added. After about 5 min, methyl 2-amino-4-chlorobenzoate (1 equiv., 4.36 mmol, 0.8093 g) was added, and the mixture was heated to 120 °C in an oil bath for 2 h until the starting material disappeared (monitored by TLC). After cooling the mixture to rt, CH2Cl2 was added (50 mL) and a grey precipitate was collected by gravity filtration and discarded. The filtrate collected in a 250 mL glass round-bottom flask was then evaporated to dryness under reduced pressure and purified by liquid chromatography on a silica gel column using CH2Cl2/hexane (3
:
1 v/v) as eluent. Orange solid, η = 90% (1.3213 g, 3.92 mmol); 1H-NMR (400 MHz, DMSO-d6) δH 3.85 (3H, s); 3.88 (3H, s); 6.98 (1H, dd, J = 8.6 Hz, J = 2.0 Hz); 7.20 (1H, d, J = 2.0 Hz); 7.36 (1H, dd, J = 9.1 Hz, J = 3.0 Hz); 7.61 (1H, d, J = 3.0 Hz); 7.64 (1H, d, J = 9.1 Hz); 7.92 (1H, d, J = 8.6 Hz); 10.48 (1H, bs s); 13C-NMR (100 MHz, DMSO-d6) δC 52.35; 55.97; 109.09; 113.18; 115.17; 119.57; 122.67; 124.32; 128.90; 133.15; 139.01; 140.79; 145.75; 154.56; 166.84; m/z (ESI-IT MS, +) 337.07 (M + H+), M+ (C15H13ClN2O5) requires 336.05; retention factor (Rf), 0.46 in CH2Cl2/hexane 3
:
1 (v/v); HPLC-DAD: retention time (RT), 25.620 min, purity degree (peak relative area) 100%.
Synthesis of compound 7 (step vib).
A suspension of phenol (15 equiv., 3.81 mmol, 0.3586 g), Cs2CO3 (1 equiv., 0.25 mmol, 0.0828 g), N,N-diethylpentane-1,4-diamine (4 equiv., 1.02 mmol, 0.20 mL) and compound 6 (1 equiv., 0.25 mmol, 0.0820 g) in anhydrous DMSO (15 mL) was prepared in a 50 mL glass round-bottom flask. Then, the mixture was transferred to a 35 mL reaction glass vessel, sealed, and submitted to MW heating (100 W) for 40 minutes, at 120 °C. Finished this time, the mixture was cooled to rt, diluted with CH2Cl2 (25 mL), and, washed with a 2 M aqueous NaOH solution (3 × 50 mL) in a 100 mL glass separatory funnel. The organic layer was collected into a 250 mL Erlenmeyer flask, dried over anhydrous Na2SO4, filtered by gravity, and evaporated to dryness under reduced pressure to yield the crude product. This was next purified by liquid chromatography on a silica gel column, using CH2Cl2/CH3OH (4
:
1 v/v) as eluent. Red oil, η = 48% (51.5 mg, 0.12 mmol); 1H-NMR (400 MHz, DMSO-d6) δH 0.74 (6H, t, J = 7,1 Hz), 1.15–1.36 (2H, m), 1.44 (3H, d, J = 6.4 Hz), 1.55–1.64 (1H, m), 1.73–1.82 (1H, m), 2.12–2.17 (2H, m), 2.20 (4H, q, J = 7.1 Hz), 3.98 (3H, s), 4.03–4.14 (1H, m), 6.87 (1H, d, J = 9.76 Hz), 7.43 (1H, dd, J = 9.3 Hz; J = 2.2 Hz), 7.85 (1H, d, J = 2.2 Hz), 7.86 (1H, d, J = 2.6 Hz), 8.05 (1H, d, J = 2.6 Hz), 8.32 (1H, d, J = 9.32 Hz); 13C-NMR (100 MHz, DMSO-d6) δC 11.30, 21.85, 23.36, 36.10, 46.07, 51.69, 56.14, 56.41, 104.78, 115.51, 117.34, 118.22, 124.04, 126.42, 127.30, 135.10, 137.10, 148.54, 149.15, 151.62, 152.82; m/z (ESI-IT MS, +) 445.47 (M + H+), M+ (C23H30ClN4O3) requires 444.19; Rf, 0.45 in CH2Cl2/CH3OH 4
:
1 (v/v); HPLC-DAD: RT, 11.913 min, purity degree (peak relative area) 96%.
Synthesis of compound 9 (step viiib).
In a 25 mL glass round-bottom flask charged with a magnetic stir bar and put under inert conditions (argon atmosphere), compound 8 (1 equiv., 0.10 mmol, 0.0424 g) was dissolved in DCE (10 mL) and then, compound 17 (1.1 equiv., 0.11 mmol, 0.0239 g) and NaBH(OAc)3 (2 equiv., 0.20 mmol, 0.0424 g) were added. After stirring at rt for 4 h, CH2Cl2 (25 mL) was added and the mixture was washed with a saturated aqueous NaHCO3 solution (3 × 25 mL) in a 100 mL glass separatory funnel, dried with anhydrous Na2SO4, filtered by gravity, and concentrated by evaporation under reduced pressure. Purification by liquid chromatography on a silica gel column using CH2Cl2/CH3OH (4
:
1 v/v) as eluent delivered the target compound; Orange oil, η = 48% (30.5 mg, 0.05 mmol); 1H-NMR (400 MHz, CDCl3) δH 1.19 (6H, t, J = 7.6 Hz), 1.32 (3H, d, J = 6.3 Hz), 1.60–1.77 (2H, m), 1.80–1.91 (6H, m), 2.85 (2H, t, J = 7.5 Hz), 2.93 (4H, q, J = 7.1 Hz), 3.31 (2H, t, J = 6.1 Hz), 3.76 (2H, t, J = 6.6 Hz), 3.93 (3H, s), 3.99–4.08 (1H, m), 6.24 (1H, d, J = 2.2 Hz), 6.59 (1H, m), 7.26 (1H, dd), 7.67–7.69 (2H, m), 7.79–7.81 (2H, m), 8.00 (1H, d, J = 9.2 Hz), 8.07 (1H, d, J = 1.8 Hz); 13C-NMR (100 MHz, CDCl3) δC 168.53, 158.54, 134.03, 132.17, 125.01, 124.72, 123.29, 55.69, 55.34, 51.77, 46.67, 43.11, 37.80, 35.37, 29.78, 26.55, 26.29, 22.29, 20.94, 8.48, 0.08; m/z (ESI-IT MS, +) 616.73 (M + H+), M+ (C35H42ClN5O3) requires 615.30; Rf, 0.46 in CH2Cl2/CH3OH 8
:
1 (v/v); HPLC-DAD: RT, 15.347 min, purity degree (peak relative area) 89%.
Synthesis of compound 13 (step xiib).
A suspension of 11 (1 equiv., 3.75 mmol, 1.7749 g) in CH3OH (50 mL) was prepared in a 250 mL glass round-bottom flask charged with a magnetic stir bar, and after that, NaBH4 (10 equiv., 37.5 mmol, 1.4186 g) was carefully and slowly added using a spatula during 30 min (paying attention to the exothermic reaction; as during the addition the mixture heats up to boiling). Finished the addition, the mixture was left under stirring until all the starting reagent was consumed (monitored by TLC, approximately 24 h) at 80 °C in an oil bath. After that, the mixture was cooled to rt and CH3OH was eliminated by evaporation under reduced pressure. Next, the resulting residue was dissolved in CH2Cl2 (50 mL) and transferred to a 250 mL glass separatory funnel where it was washed with a saturated aqueous NaHCO3 solution (3 × 50 mL). The organic layer was collected in a 250 mL Erlenmeyer flask, dried over anhydrous Na2SO4, filtered by gravity, and concentrated under reduced pressure. The residue was incorporated in the silica (1 g) and then purified by liquid chromatography on a silica gel column using CH2Cl2 as eluent. Light-yellow solid, η = 64% (0.6914 g, 2.39 mmol); 1H-NMR (400 MHz, DMSO-d6) δH 4.01 (3H, s), 7.70 (1H, dd, J = 9.0 Hz, J = 2.1 Hz), 7,80 (1H, d, J = 2.7 Hz), 8.16 (1H, d, J = 2.0 Hz), 8.24–8.27 (2H, m), 9.18 (1H, s); 13C-NMR (100 MHz, DMSO-d6) δC 56.49, 108.10, 119.68, 125.46, 127.21, 127.27, 128.07, 130.39, 135.59, 135.82, 136.54, 148.29, 154.76; m/z (ESI-IT MS, +) 289.53 (M + H+), M+ (C14H9ClN2O3) requires 288.03; Rf, 0.77 in CH2Cl2; HPLC-DAD: RT, 23.817 min, purity degree (peak relative area) 96.4%.
Synthesis of compound 16 (step xiv).
In a 250 mL glass round-bottom flask charged with a magnetic stir bar, 4-aminobutan-1-ol (1 equiv., 6.93 mmol, 0.64 mL) and phthalic anhydride (1 equiv., 6.93 mmol, 1.0270 g) were dissolved in dioxane (100 mL) and left under stirring overnight at 100 °C in an oil bath. Then, dioxane was removed under reduced pressure and 50 mL of CH2Cl2 was added to the flask. The mixture was transferred to a 250 mL glass separating funnel and washed with a saturated aqueous NaCl solution (3 × 50 mL). The resulting organic phase was collected in a 250 mL Erlenmeyer flask, dried over anhydrous Na2SO4, filtered by gravity, and evaporated to dryness under reduced pressure. The residue was then purified by liquid chromatography on a silica gel column using CH2Cl2/CH3OH (4
:
1 v/v) as eluent. Yellowish solid, η = 70% (1.0633 g, 4.85 mmol); 1H-NMR (400 MHz, DMSO-d6) δH 1.44–1.37 (2H, m), 1.66–1.58 (2H, m), 3.39 (2H, q, J = 2.0 Hz), 3.57 (2H, t, J = 7.1 Hz), 4.38 (1H, t, J = 5.2 Hz), 7.88–7.81 (4H, m); 13C-NMR (100 MHz, DMSO-d6) δC 24.71, 29.77, 37.37, 60.15, 122.96, 131.58, 134.34, 167.93; Rf, 0.86 in CH2Cl2/CH3OH 4
:
1 (v/v); HPLC-DAD: RT, 12.280 min, purity degree (peak relative area) 99.8%.
Synthesis of compound 17 (step xv).
In a 250 mL glass round-bottom flask charged with a magnetic stir bar and placed under inert conditions (argon atmosphere), compound 16 (1 equiv., 4.51 mmol, 0.9892 g) was dissolved in anhydrous CH2Cl2 (100 mL) followed by the addition of DMP (1.1 equiv., 4.96 mmol, 2.1037 g). The mixture reacted at rt for 1 h and a white precipitate was formed, which was collected by gravity filtration and discarded. The filtrate was evaporated to dryness under reduced pressure and purified by liquid chromatography on a silica gel column using hexane/AcOEt (1
:
1 v/v) as eluent. Yellowish oil, η = 63% (0.6169 g, 2.84 mmol); 1H-NMR (400 MHz, DMSO-d6) δH 1.84 (2H, p, J = 7.0 Hz), 2.54–2.51 (2H, m), 3.59 (2H, t, J = 6.8 Hz), 7.88–7.81 (4H, m), 9.64 (1H, t, J = 1.1 Hz); 13C-NMR (100 MHz, DMSO-d6) δC 20.61, 36.77, 122.96, 131.65, 134.30, 167.97, 200.46; Rf, 0.61 in hexane/AcOEt 1
:
1 (v/v).
Concluding remarks
Acridine-based compounds have for long attracted the interest of researchers across disciplines, and such interest endures due to the unique chemical, physical, and biological properties of those heterocyclic molecules. Our research group makes no exception to this rule, very much driven by our long-term interest in anti-infective agents, including antimalarials inspired in mepacrine.14–17,33–38 This led us to advance, in recent years, new 4-aminoacridines (15) and 4,9-diaminoacridines (10) as multi-stage antiplasmodial hits.15,16 Although we were successful in addressing their quite challenging synthesis, the overall modest yields obtained as well as harsh and/or time-consuming procedures involved prompted us to work on the optimization of the synthetic routes. The efforts undertaken are herein described and allowed us to make significant progress on several parts of the overall synthesis scheme. As such, the synthesis of compound 6, a common precursor to both families of the target aminoacridines, can now be achieved in three (Scheme 2, part A) instead of five (Scheme 1, part A) steps, using two commercially available reagents and offering an overall yield of 63%, which more than doubles that of the previous approach (24%). Also, the introduction of side chains in the C-9 and C-4 positions of the acridine ring in the route to 4,9-diaminoacridines 10 (Scheme 1, steps vi and viii) could be significantly improved by, respectively, use of MW heating in step vi, and change from a bimolecular nucleophilic substitution into a reductive amination in step viii (Scheme 2, part B, steps vib and viiib); overall, this simplified experimental procedures and considerably reduced reaction time and the need to use high temperatures, while improving yield. Finally, the synthesis route to 4-aminoacridines 15 could also be improved, mainly by a fine tuning of experimental conditions of the reduction steps leading to the 4-aminoacridine intermediate 12 (Scheme 2, part B, steps xib and xiib).
To sum up, after these efforts, 4,9-diaminoacridines 15 can be produced from 6 by four reactional steps (SNAr in C-9, reduction in C-4, reductive amination in C-4, and hydrazinolysis) in 21% overall yield that is about 2-fold higher than that previously reported (13%).15 Likewise, 4-aminoacridines 10 can be now delivered from 6 by five reactional steps (SNAr in C-9, reduction in C-9 and C-4, SN2 in C-4 and hydrazinolysis) with an overall yield of 34%, also superior to that afforded by the previous synthetic route (21%).15,16 Overall, this translates into a more convenient and sustainable production of functionalized acridines, paving the way towards future synthetic approaches targeting acridine-based compounds.
Conflicts of interest
There are no conflicts to declare.
Acknowledgements
This work received financial support from PT national funds (FCT/MCTES, Fundação para a Ciência e Tecnologia and Ministério da Ciência, Tecnologia e Ensino Superior) through project CIRCNA/BRB/0281/2019. The authors further thank FCT/MCTES for supporting Research Unit LAQV-REQUIMTE (10.54499/LA/P/0008/2020; 10.54499/UIDP/50006/2020; 10.54499/UIDB/50006/2020) and for the Doctoral Grant to MF (SRFH/BD/147354/2019). MP further acknowledges the “la Caixa” Foundation for Grant HR21-848.
Notes and references
-
N. R. Raulins, Acridines, in Chemistry of Heterocyclic Compounds, ed. R. M. Acheson, John Wiley & Sons, Inc., 2nd edn, 1973, ch. 1, pp. 9–108 Search PubMed.
-
R. M. Acheson, Nomenclature and Numbering System, in Chemistry of Heterocyclic Compounds, ed. R. M. Acheson, John Wiley & Sons, Inc., 2nd edn, 1973, vol. 9, pp. 1–8 Search PubMed.
- P. Prasher and M. Sharma, MedChemComm, 2018, 9, 1589–1618 RSC.
- M. Gensicka-Kowalewska, G. Cholewiński and K. Dzierzbicka, RSC Adv., 2017, 7, 15776–15804 RSC.
-
R. Kumar, S. Sharma and D. Prasad, Acridones: A Relatively Lesser Explored Heterocycle for Multifactorial Diseases, in Key Heterocycle Cores for Designing Multitargeting Molecules, ed. O. Silakari, Elsevier, 1st edn, 2018, ch. 3, pp. 53–132 Search PubMed.
- R. Kumar, M. Kaur and M. Kumari, Acta Pol. Pharm., 2012, 69, 3–9 CAS.
- A. Bernthsen, Justus Liebigs Ann. Chem., 1884, 224, 1–56 CrossRef.
-
J. Ježek, J. Hlaváček and J. Šebestík, Syntheses, in Biomedical Applications of Acridines: Derivatives, Syntheses, Properties and Biological Activities with a Focus on Neurodegenerative Diseases, ed. J. Ježek, J. Hlaváček and J. Šebestík, Springer International Publishing, Cham, 2017, vol. 72, pp. 9–45 Search PubMed.
-
A. Schmidt and M. Liu, Recent Advances in the Chemistry of Acridines, in Advances in Heterocyclic Chemistry, ed. E. F. V. Scriven and C. A. Ramsden, Academic Press, 2015, ch. 4, vol. 115, pp. 287–353 Search PubMed.
- J. Chiron and J.-P. Galy, Synthesis, 2004, 313–325 CrossRef CAS.
- Z. Zawada, J. Šebestík, M. Šafařík and P. Bouř, Eur. J. Org Chem., 2011, 6989–6997 CrossRef CAS.
- M. Kozurkova, D. Sabolova and P. Kristian, J. Appl. Toxicol., 2021, 41, 175–189 CrossRef CAS.
- M. Fonte, N. Tassi, P. Gomes and C. Teixeira, Molecules, 2021, 26, 600 CrossRef CAS PubMed.
- A. Gomes, B. Pérez, I. Albuquerque, M. Machado, M. Prudêncio, F. Nogueira, C. Teixeira and P. Gomes, ChemMedChem, 2014, 9, 305–310 CrossRef CAS.
- M. Fonte, N. Fagundes, A. Gomes, R. Ferraz, C. Prudêncio, M. J. Araújo, P. Gomes and C. Teixeira, Tetrahedron Lett., 2019, 60, 1166–1169 CrossRef CAS.
- M. Fonte, N. Tassi, D. Fontinha, I. Bouzón-Arnáiz, R. Ferraz, M. J. Araújo, X. Fernàndez-Busquets, M. Prudêncio, P. Gomes and C. Teixeira, ChemMedChem, 2021, 16, 788–792 CrossRef CAS.
- B. Pérez, C. Teixeira, A. S. Gomes, I. S. Albuquerque, J. Gut, P. J. Rosenthal, M. Prudêncio and P. Gomes, Bioorg. Med. Chem. Lett., 2013, 23, 610–613 CrossRef.
- M. O. Anderson, J. Sherrill, P. B. Madrid, A. P. Liou, J. L. Weisman, J. L. DeRisi and R. K. Guy, Bioorg. Med. Chem., 2006, 14, 334–343 CrossRef CAS PubMed.
- S. Young Kim, Y. Ri Kim, H. Tae Kim, A. Kumar Jaladi and D. Keun An, ChemistrySelect, 2022, 7, e202202351 CrossRef CAS.
- A. R. Jeon, M. E. Kim, J. K. Park, W. K. Shin and D. K. An, Tetrahedron, 2014, 70, 4420–4424 CrossRef CAS.
- N. Boechat, J. C. S. da Costa, J. de Souza Mendonça, P. S. M. de Oliveira and M. V. N. D. Souza, Tetrahedron Lett., 2004, 45, 6021–6022 CrossRef CAS.
- C. P. Prasanth, E. Joseph, A. Abhijith, D. S. Nair, I. Ibnusaud, J. Raskatov and B. Singaram, J. Org. Chem., 2018, 83, 1431–1440 CrossRef CAS PubMed.
- A. Saeed and Z. Ashraf, J. Chem. Sci., 2006, 118, 419–423 CrossRef CAS.
-
A. de la Hoz, A. Díaz-Ortiz and P. Prieto, Microwave-Assisted Green Organic Synthesis, in Alternative Energy Sources for Green Chemistry, ed. G. Stefanidis and A. Stankiewicz, The Royal Society of Chemistry, 23rd edn, 2016, ch. 1, pp. 1–33 Search PubMed.
- M. Staderini, N. Cabezas, M. L. Bolognesi and J. C. Menéndez, Tetrahedron, 2013, 69, 1024–1030 CrossRef CAS.
- S. D. Meyer and S. L. Schreiber, J. Org. Chem., 1994, 59, 7549–7552 CrossRef CAS.
- D. Setamdideh and B. Khezri, Asian J. Chem., 2010, 22, 5575–5580 CAS.
- H. K. Kadam and S. G. Tilve, RSC Adv., 2012, 2, 6057–6060 RSC.
- A. J. MacNair, M.-M. Tran, J. E. Nelson, G. U. Sloan, A. Ironmonger and S. P. Thomas, Org. Biomol. Chem., 2014, 12, 5082–5088 RSC.
- M. Ficker, J. F. Petersen, J. S. Hansen and J. B. Christensen, Org. Prep. Proced. Int., 2014, 46, 176–182 CrossRef CAS.
- K. Hanaya, T. Muramatsu, H. Kudo and Y. L. Chow, J. Chem. Soc., Perkin Trans. 1, 1979, 2409–2410 RSC.
- H. Bailey, W. Heaton, N. Vicker and B. Potter, Synlett, 2006, 2006, 2444–2448 CrossRef.
- B. Pérez, C. Teixeira, J. Gut, P. J. Rosenthal, J. R. B. Gomes and P. Gomes, ChemMedChem, 2012, 7, 1537–1540 CrossRef.
- B. C. Pérez, C. Teixeira, I. S. Albuquerque, J. Gut, P. J. Rosenthal, J. R. B. Gomes, M. Prudêncio and P. Gomes, J. Med. Chem., 2013, 56, 556–567 CrossRef.
- B. C. Pérez, C. Teixeira, M. Figueiras, J. Gut, P. J. Rosenthal, J. R. B. Gomes and P. Gomes, Eur. J. Med. Chem., 2012, 54, 887–899 CrossRef.
- B. Pérez, C. Teixeira, I. S. Albuquerque, J. Gut, P. J. Rosenthal, M. Prudêncio and P. Gomes, MedChemComm, 2012, 3, 1170–1172 RSC.
- A. Gomes, I. Fernandes, C. Teixeira, N. Mateus, M. J. Sottomayor and P. Gomes, ChemMedChem, 2016, 11, 2703–2712 CrossRef CAS PubMed.
- A. Gomes, M. Machado, L. Lobo, F. Nogueira, M. Prudêncio, C. Teixeira and P. Gomes, ChemMedChem, 2015, 10, 1344–1349 CrossRef CAS PubMed.
Footnotes |
† Electronic supplementary information (ESI) available: 1H-NMR and 13C-NMR spectra, ESI-MS and HPLC chromatograms shown in the ESI only refer to compounds that were synthesized using an improved protocol. See DOI: https://doi.org/10.1039/d4ra00091a |
‡ Current affiliation: Gyros Protein Technologies Inc., Tucson, Arizona, USA. |
§ The procedures described in the Experimental section only refer to the new procedures that led to an improvement on the overall synthesis routes, as depicted in the synthetic route B (Scheme 2). |
¶ The procedures employed in the synthetic route A (Scheme 1) have been already described in detail in ref. 15 and 16. |
|
This journal is © The Royal Society of Chemistry 2024 |
Click here to see how this site uses Cookies. View our privacy policy here.