DOI:
10.1039/D4RA01438C
(Paper)
RSC Adv., 2024,
14, 18126-18135
Deep eutectic solvent extraction of myricetin and antioxidant properties†
Received
24th February 2024
, Accepted 28th May 2024
First published on 6th June 2024
Abstract
In this study, a response surface method (RSM) was used to optimise the ultrasonic-assisted deep eutectic solvent (DES) extraction of myricetin from myricetin leaves. The results demonstrated that the DES-5 (choline chloride–oxalic acid) system exhibited better extraction results than the other seven DESs prepared. The optimum extraction conditions for myricetin were a DES-5 system with 19% water content of DES, a liquid-to-solid ratio of 37
:
1 mL g−1, an extraction time of 45 min, and an extraction temperature of 72 °C. Under these conditions, the extraction amount of myricetin was 22.47 mg g−1. To optimise the extraction process, the crude myricetin extract was purified, and the optimal conditions were as follows: an AB-8 macroporous adsorption resin was used with an anhydrous ethanol desorption agent. The adsorption rate was 1 BV per h (bed volume per hour), the desorption rate was 1 BV per h, and the desorption capacity was 2 BV (bed volume). The antioxidant properties of the myricetin were also investigated. The results demonstrated that, with an increase in concentration, the scavenging rates of DPPH and ˙OH free radicals increased. Compared to Vc, myricetin had a better scavenging ability for DPPH free radicals, whereas purified myricetin had a better antioxidant effect. At the same concentration, the radical-scavenging rate of the ˙OH radical was slightly higher in myricetin purified by the macroporous adsorption resin than in Vc, and that of the unpurified myricetin was the smallest. Myricetin was purified using a macroporous adsorption resin to improve its antioxidant properties.
1 Introduction
Myrica is an evergreen tree of the genus Myrica that has high medicinal value.1 Myricetin, a polyhydroxyl flavonoid, is the main active ingredient in the leaves of Myrica rubra.2 The phenolic hydroxyl group in its structure can react with free radicals to form a more stable semiquinone free radical, thereby effectively scavenging free radicals.3 Myricetin has good biological activity and is widely used in medicine, healthcare, and food.4
The ultrasonic-assisted extraction technique uses the cavitation effect of ultrasound to increase the frequency of movement of macromolecules, thus increasing the penetration of the deep eutectic solvent (DES), and mechanical and thermal effects of ultrasound to increase the dissolution rate of myricetin, thus increasing the amount of dissolution.5,6 Ultrasound-assisted extraction technology has been widely used for the extraction of natural products because of its short extraction time, high extraction efficiency, and environmental friendliness.7–10
DES is prepared by mixing hydrogen bond acceptors (quaternary ammonium salts, amphoteric ions, etc.) with hydrogen bond donors (amides, carboxylic acids, etc.) in a certain molar ratio and has the advantages of low toxicity, green, and is environmentally friendly.11–14 Using DES as an extraction solvent combined with ultrasonic-assisted extraction technology can further improve the extraction efficiency of natural plants and has promising applications in the extraction and separation of natural plants. Oliveira et al.15 developed seven DES and used pectin lyase and DES to extract high concentrations of flavonoids from citrus pomace. The extraction effect and antioxidant activity of flavonoids and total phenolic compounds from citrus pomace using DES were higher than those of conventional extraction, and it had excellent extraction potential and was related to pectin lyase. Du et al.,16 confirmed that DES (betaine hydrochloride
:
sucrose
:
water = 1
:
1
:
94.50) was a more efficient and green extraction solvent when extracting jasmine flavonoids with DES compared with water and ethanol, which are two traditional solvents. Wu et al.9 adopted an ultrasonic-assisted natural deep eutectic solvent method (UAE-NADES) to extract five polyphenols greenly and efficiently (chlorogenic acid, (+)-catechin, protocatechuic acid, caffeic acid, and gallic acid) from Chaenomeles speciosa (CSP), and choline chloride–urea–malonic acid (ChCl–Ur–MA) was identified as the optimal composition for NADES. The total yield of five components was 3.92 ± 0.03 mg g−1, which was higher than traditional organic solvents. Wang et al.17 adopted a low eutectic solvent oscillation-assisted extraction (DES-OS) combined with a macroporous resin adsorption–desorption technique to achieve rapid green extraction and separation of dihydromyricetin (DMY), a characteristic component of vine tea. Multivariate data analysis demonstrated that the DES system composed of tetrabutylammonium bromide (N444Br) and pyruvate (molar ratio: 1
:
2) enhanced the destruction of the DMY cell wall structure, resulting in the release of more DMY and good extraction performance. Liu et al.,18 used natural eutectic solvents to extract five important coumarins from Angelica dahurica: bergapten, oxypeucedanin, imperatorin, cnidilin, and isoimperatorin. Compared to conventional extraction methods, the extraction efficiency of this method increased by 10.74%. Wang et al.,19 used ultrasonic-assisted extraction (DES-UAE) with deep eutectic solvent to extract the Selaginella chaetoloma total biflavonoids (SCTB). Tetrapropylamonium bromide-1,4-butanediol (Tpr-But) was used as the extractant for optimisation. DES-UAE saves time and increases the extraction rate of SCTB by 1.5–3 times. SCTB has excellent antioxidant activity and can effectively inhibit cancer cell growth. Rashid et al.,20 used a natural eutectic solvent (NADES) in combination with ultrasound-assisted extraction (UAE) to extract bioactive compounds from apple pomace. The solvent that outperformed conventional solvents was DES1 (choline chloride
:
glycerin (1
:
2)). High-performance liquid chromatography (HPLC) of the optimised extract was used to quantify the polyphenol compounds. Quercetin was the main compound present, followed by chlorogenic acid, gallic acid, phloretin, phloretin, and rutin. Peng et al.,21 selected low eutectic solvent (choline chloride (ChCl)–urea) to extract flavonoids from Moringa oleifera leaves (FMOL) with the highest extraction efficiency of 63.2 ± 0.3 mg R per g DW, and identified 9 types of flavonoids. FMOL is superior to positive control drugs in terms of antioxidant activity. This study confirmed that FMOL extracted with (ChCl)–urea is rich in bioactive components and that microencapsulated FMOL has potential applications in the food industry. Ultrasonic-assisted DES extraction technology has become a research hotspot in the field of natural product research in recent years, with significant potential for development.22,23 At present, research on this aspect mainly focuses on process conditions, such as the water content of the DES, liquid–solid ratio, extraction temperature, extraction time, and other factors.24,25
Macroporous resin adsorption is based on the van der Waals attraction between the resin and myricetin through the specific surface physical adsorption of the resin, followed by eluent purification and separation using myricetin technology.26,27 Myricetin extracted using ultrasound-assisted DES and further purified by macroporous resin can have better biological activity and significantly expand its applications.28–30
In this study, we investigated the process conditions for the DES extraction of myricetin and its separation and purification by macroporous resin. Finally, the antioxidant activity of the myricetin was evaluated. This study aimed to provide a reference for the extraction, purification, and application of myricetin from Myrica rubra leaves.
2 Materials and methods
2.1 Instruments and chemicals
2.1.1 Main instruments. FW117 herbal pulveriser (Tianjin Teste Instruments Co., Ltd), BSA224S-CW Electronic Balance (Sartorius Scientific Instruments (Beijing) Co., Ltd), GW1030 ultrasonic cleaning machine (Shenzhen Guanbo Technology Co., Ltd), LD5-10 low-speed centrifuge (Beijing Jingli Centrifuge Co., Ltd), Cary50 ultraviolet-visible spectrophotometer (Varian), and WGLL-230BE electric blast drying oven.
2.1.2 Chemicals. Myricetin standard (≥97%, Shanghai Dibo Biotechnology Co., Ltd). Myrica rubra leaves collected in Yuyao, Zhejiang Province, China. Vitamin C tablets (Xi'an Lijun Pharmaceutical Co. Ltd). DPPH (≥98%, Shanghai Ika Biotechnology Co., Ltd). Choline chloride (ChCl, AR, 98%), 1,2-propanediol (C3H8O2, AR, 99%), glycerol (C3H8O3, 98%), D-fructose (C6H12O6, 99%), vanillin (C8H8O3) were obtained from Aladdin Chemistry Co. Ltd. Ethanedioic acid (H2C2O4, ≥99.5%), propanedioic acid (C3H4O4, 98%), lactic acid (C3H6O3, 85%), urea (CH4N2O, ≥99.5%), triethanolamine (C6H15NO3, AR) were purchased from Shanghai Macklin Biochemical Co., Ltd. Ethanol (CH3CH2OH, AR, 95.0%), methanol (CH3OH, AR, 99.5%), ferrous sulfate (FeSO4·7H2O, AR, ≥99.0%), hydrogen peroxide (H2O2, AR, 30 wt% in H2O), and salicylic acid (C7H6O3, AR, 99.5%) were purchased from Chengdu Alfa Biotechnology Co., Ltd (Chengdu, China).
2.2 Preparation of DES
Combined with the research reports on the extraction of flavonoids in plants, hydrophilic DESs were selected to extract Myricetin.21,22 According to the molar ratio of hydrogen bond acceptor to hydrogen bond donor in Table 1, a certain mass of hydrogen bond acceptor and hydrogen bond donor was weighed into a 250 mL beaker, and the glass rod was stirred and mixed evenly. The DES was prepared by heating at 80 °C with magnetic stirring.
Table 1 DES components and molar ratios
Number |
Components |
Mole ratio |
DES-1 |
Choline chloride–glycerol |
1 : 2 |
DES-2 |
Choline chloride–ethylene glycol |
1 : 1 |
DES-3 |
Choline chloride–ethylene glycol |
1 : 2 |
DES-4 |
Choline chloride–D-fructose |
1 : 2 |
DES-5 |
Choline chloride–ethanedioic acid |
1 : 1 |
DES-6 |
Choline chloride–propanedioic acid |
1 : 1 |
DES-7 |
Choline chloride–lactic acid |
1 : 2 |
DES-8 |
Choline chloride–urea |
1 : 2 |
DES-9 |
Choline chloride–triethanolamine |
1 : 1 |
DES-10 |
Choline chloride–ethylene glycol–urea |
1 : 1 : 1 |
DES-11 |
Choline chloride–vanillin–lactic acid |
1 : 1 : 1 |
2.3 DES extraction of myricetin in Myrica rubra leaves
2.3.1 Determination of the maximum absorption wavelength. Twenty milligrams of the myricetin standard in a 50 mL beaker was dissolved in a 50 mL beaker with a volume fraction of 80% ethanol aqueous solution by volume, transferred to a 100 mL volumetric bottle, and shaken well to fix the volume. The maximum absorption wavelength of myricetin was determined by measuring the wavelength of the myricetin solution after a certain proportion of dilution using UV spectrophotometry.
2.3.2 Preparation of standard curve of myricetin. The myricetin standard (20 mg) was accurately weighed and dissolved in a 100 mL beaker with methanol, transferred to a 100 mL volumetric bottle, and shaken well to determine the volume. Subsequently, 1.0, 2.0, 3.0, 4.0, 5.0, 6.0, and 7.0 mL myricetin standard solution were put into five-10 mL volumetric flasks, respectively, diluted with methanol, and shaken well at a constant volume. A 1–1.5 mL of diluted standard solution was taken to a 0.22 μm filter membrane and the peak area of the myricetin standard was determined; the standard curve was plotted on a horizontal coordinate as the concentration of the solution (μg mL−1) and vertical coordinate as the peak area. Chromatographic conditions: methanol
:
water = 40
:
60 of mobile phase, detection wavelength 370 nm, column: C18 (4.6 mm × 150 mm, 5 μm), flow rate 1.0 mL min−1.
2.3.3 Pre-treatment of Myrica rubra leaves. The leaves were washed with distilled water, dried naturally, and put in an oven at 60 °C until they were absolutely dry. The bayberry leaves were pulverised using a Chinese herbal crusher, sifted through a 40-mesh sieve, and the powder was sealed for later use.
2.3.4 Extraction of myricetin. A 100 mg of arbutus leaf powder was accurately weighed in a 10 mL centrifuge tube, 2.5 mL of DES with 2.5 mL of 80% ethanol aqueous solution by volume was added, and a centrifuge tube was put into an ultrasonic cleaning machine for ultrasonic-assisted extraction. After ultrasonication, the samples were cooled to room temperature and centrifuged, and the supernatant was collected to record the volume (V) and the crude extract of myricetin was obtained. The sample was diluted severally, the absorbance of the diluted sample was measured, and the concentration of the crude extract of myricetin (C) was calculated based on the standard curve equation; each experiment was repeated three times. Finally, the amount of extracted myricetin was calculated using eqn (1-1).Extraction amount of myricetin:
|
 | (1-1) |
C—the concentration calculated from the standard curve equation, mg mL
−1,
V—the volume of myricetin extract, mL,
N—dilution ratio,
M—powder quality of bayberry leaves, g.
2.4 Separation and purification of myricetin
2.4.1 Separation and purification of myricetin. To separate and purify the myricetin extract, the resin was added to a glass chromatography column, and a 10 cm (5% mass concentration) hydrochloric acid solution was added above the resin layer and soaked for 2–4 hours. A 5% (mass concentration) hydrochloric acid solution of 4 times the volume of the column, was then passed through the column. Distilled water was added to wash the solution to neutral pH. The resin was then soaked in 5% (mass concentration) NaOH solution for 4 hours. The column was washed four times with a 5% (mass concentration) NaOH solution. Finally, distilled water was used for washing until the pH was neutralised.The crude extract of myricetin obtained from experiment 2.3.4, was passed through the column at a rate of 1 BV per h, and the resin was washed with distilled water. Ethanol was then used for elution at rates of 2 BV and 1 BV per h. The effluent was collected to obtain the myricetin extract, which was stored for further use. The eluate was collected, concentrated by vacuum using a rotary evaporator and finally dried under vacuum to obtain crystal powder.
2.4.2 Adsorption rate test of macroporous resin. The crude extract of myricetin obtained in experiments 2.3.4, was passed through the column at rates of 1, 2, and 3 BV per h. The effluent was collected, and the absorbance was measured.
2.4.3 Adsorption analysis rate test of macroporous resin. The crude extract of myricetin obtained in experiment 2.3.4, was passed through the column at a rate of 1 BV per h, respectively, and the resin was washed with distilled water and then eluted with ethanol at rates of 1, 2, and 3 BV per h. The effluent was collected, and the absorbance was measured.
2.4.4 Desorption capacity test of macroporous resin. The crude extract of myricetin obtained in experiments 2.3.4, was passed through the column at a rate of 1 BV per h, and the resin was washed with distilled water. Subsequently, ethanol was administered at 1 BV per h at dosages of 1, 2, and 3 BV. The effluent was collected, and the absorbance was measured.
2.4.5 Calculation of adsorption capacity and desorption rate of macroporous resin. The adsorption rate of the macroporous resin was calculated using eqn (1-2), and the desorption rate of the resin was calculated using eqn (1-3). |
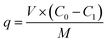 | (1-2) |
where q is the resin adsorption capacity (mg g−1); V is the volume of crude extract (mL); C0 is the concentration of myricetin in the sample solution of Myrica rubra leaves (mg mL−1); C1 is the concentration of myricetin adsorbed by the crude extract (mg mL−1); and M is the resin dry weight (g). |
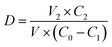 | (1-3) |
where D is the desorption rate of the adsorbent resin; C2 is the concentration of myricetin in the resolving solution (mg mL−1); V2 is the volume of the resolving solution, mL; V is the volume of the crude extract solution (mL); C0 is the concentration of myricetin in the sample solution of Myrica rubra leaves (mg mL−1); and C1 is the concentration of myricetin in the complete crude extract solution (mg mL−1).
2.4.6 Determination of sample purity. Take the crystal powder obtained in 2.4.1 and use the HPLC conditions in 2.3.2 to determine the peak area and calculate the purity of myricetin in the powder (=mass of myricetin in the powder/mass of the powder).31,32
2.5 Antioxidant test
2.5.1 DPPH method. DPPH-scavenging capacity was determined with reference to Wang et al.,33 eight-10 mL test tubes with plugs were used to extract 2 mL crude myricetin of 2, 4, 6, 8, 10, 12, 14, and 16 μg mL−1 of myricetin (1.3.4 extraction of myricetin). Next, 2 mL of DPPH solution (6.34 × 10−4 mmol L−1) was added to each stoppered test tube, shaken well, and allowed to stand for 30 min away from light. The absorbance value was measured as A with a visible spectrophotometer, the wavelength was adjusted to 517 nm, anhydrous ethanol plus DPPH solution was used as a blank control and recorded as A0, and clearance was recorded as (1-4).The above experiments were repeated, and the crude myricetin extract was replaced with the myricetin extract after separation and purification of 2.4.1.
The above experiment was repeated, and the crude myricetin extract was replaced with the same concentration of Vc solution.
2.5.2 ˙OH radical method. Determination based on salicylic acid method,34 eight-10 mL test tubes with plug were used to measure 2 mL of 2 L, 4, 6, 8, 10, 12, 14, and 16 μg mL−1 of myricetin solution. Subsequently, a 1 mL of 9 mmol per L FeSO4 solution was added, then 1 mL of 8.8 mmol per L H2O2 solution was added. After 5 min reaction, a 1 mL of 5.4 mmol per L salicylic acid–ethanol solution was added and then shaken well and was measured at 510 nm after reaction in a 35 °C water bath for 30 min. Distilled water was used as a blank control instead of the sample to be tested and was recorded as A0. The radical scavenging rate was calculated according to eqn (1-4).The aforementioned experiments were repeated and the crude extract of myricetin was replaced with 2.4.1 myricetin extract after the isolation and purification of myricetin.
The aforementioned experiments were repeated with the addition of extracts replaced with the same concentration of Vc solution. Finally, the clearance was calculated using eqn (1-4).
|
 | (1-4) |
3 Result analysis and discussion
3.1 Selection of maximum absorption wavelength
The test was conducted as described in Section 2.3.1. As shown in Fig. S1,† the maximum absorption wavelength of the myricetin standard is 370 nm.
3.2 Standard curve of myricetin
As described in Section 2.3.2, the HPLC chromatogram of the standard sample is illustrated in Fig. S2.† The linear equation is A = 0.05088C + 0.03578 in the concentration range of 3.7–18.5 μg mL−1, where R2 = 0.99963, indicating that there is a good linear relationship between absorbance and myricetin standard solution concentration.
3.3 Analysis and discussion of single factor results
3.3.1 Selection of DES. The DES was prepared according to the procedure described in Section 2.2, and the myricetin extraction experiment was conducted according to Section 2.3. DES-1, DES-2, DES-3, DES-4, DES-5, DES-6, DES-7, DES-8, DES-9, DES-10, and DES-11 with 30% water content and 80% aqueous ethanol solution by volume fraction were used as the extraction solvents. The amounts of myricetin extracted from Myrica leaves were compared. The extracted amounts are illustrated in Fig. 1. The other conditions were an extraction time of 50 min, extraction temperature of 60 °C, ultrasonic power of 600 W, and a liquid-to-material ratio of 25
:
1 mL g−1. The extracted amounts are illustrated in Fig. 2.
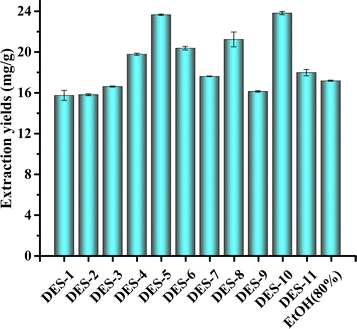 |
| Fig. 1 Effect of DES type on extraction yields. | |
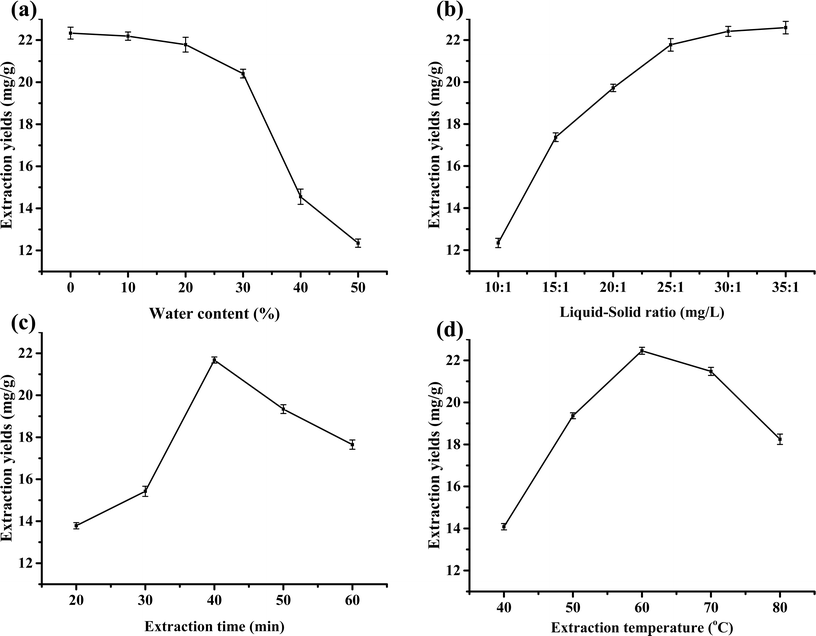 |
| Fig. 2 Effects of DES different parameters on the extraction yields of myricetin. (The extraction experiment of myricetin was performed as outlined in Section 2.3.) (a) Effect of water content on the extraction yields of myricetin. (Extraction conditions: extraction time of 50 min, extraction temperature of 60 °C, ultrasonic power of 600 W, and liquid-material ratio of 25 : 1 mL g−1.) (b) Effect of liquid–solid ratio on extraction yields of myricetin. (Extraction conditions: extraction time of 50 min, extraction temperature of 60 °C, ultrasonic power of 600 W.) (c) Effect of extraction time on the extraction amount of myricetin. (Extraction conditions: extraction time of 50 min, extraction temperature of 60 °C, ultrasonic power of 600 W, liquid–material ratio of 32 : 1 mL g−1.) (d) Effect of extraction temperature on the extraction amount of myricetin (Extraction conditions: extraction time of 50 min, the ultrasonic power of 600 W, liquid–material ratio of 32 : 1 mL g−1.). | |
As illustrated in Fig. 1, the extraction yields of DES-4, DES-5, DES-6, DES-8, and DES-10 were higher than those of 80% ethanol extraction, with DES-5 having the highest extraction yield. This was caused by the different polarities of the different DES species, with DES-5 being the closest to myricetin. The viscosities and densities of the DESs differed at different temperatures, and the extraction of DES-5 was better than that of the other 10 under the same extraction conditions. Therefore, DES-5 (choline chloride–oxalate molar ratio of 1
:
1) is a suitable extraction solvent.
3.3.2 Effect of DES water content on the extraction amount of myricetin. As illustrated in Fig. 2a, with an increase in the water content of the DES, the amount of myricetin extracted from bayberry leaves decreased. When the water content of the DES was 0–20%, the extraction amount of myricetin from Myrica rubra leaves was larger, and then with an increase in the water content of the DES, the extraction amount decreased.A possible reason for this is the change in system polarity owing to the increase in water content.35 Although the surface tension decreased with the increase in water content, the viscosity of the DES decreased accordingly, which facilitated the extraction; this is not sufficient to compensate for the change in the polarity of the system, while a small amount of bound water exists in oxalic acid itself.36 Moreover, an increase in water content can reduce the amount of DES and extraction costs.
3.3.3 Effect of liquid–solid ratio on extraction amount of myricetin. As illustrated in Fig. 2b, with an increase in the liquid–solid ratio, the extraction amount of myricetin from Myrica rubra leaves gradually increased and tended to be flat when the liquid–solid ratio reached 30
:
1. It is because with the increase of the liquid–solid ratio, that is, the amount of DES increases, myricetin is continuously extracted. When the liquid–solid ratio was increased to 30
:
1 mL g−1, myricetin was extracted; thereafter, the extraction amount remained unchanged by increasing the DES. The dosage of DES and extraction costs increased simultaneously. In summary, the most suitable liquid–solid ratio was 30
:
1 mL g−1.
3.3.4 Effect of extraction time on the extraction amount of myricetin. As illustrated in Fig. 2c, the extraction amount of myricetin from Myrica rubra leaves first increased and then decreased with an increase in ultrasonic time. At 20–40 min, with an increase in time, ultrasonic mechanical cavitation was enhanced, and the flow and penetration of the DES increased;37 thus, the amount of extraction increased. Myricetin was extracted after 40 min. The extraction time exceeded 40 min, the structure of myricetin was damaged by mechanical action, cavitation effect and thermal effect for a long time, resulting in the decomposition and loss of part of myricetin in Myrica rubra leaves, and the extraction amount decreased instead. The optimal extraction time was determined to be 40 min.
3.3.5 Effect of extraction temperature on the extraction amount of myricetin. As illustrated in Fig. 2d, with an increase in temperature, the extraction amount of myricetin first increased and then decreased, and the maximum extraction amount was reached at 60 °C. This is because of the maximum solubility of DES5 at 60 °C for myricetin. Simultaneously, the viscosity decreased with increasing temperature, which was more conducive to the dissolution of myricetin.38 When the temperature was higher than 60 °C, the extraction amount decreased. A possible reason for this is that the structure of myricetin is easily damaged by high temperatures. In summary, the extraction efficiency was higher at 60 °C.
3.4 Response surface results analysis
3.4.1 Response surface method experimental design. Based on the single-factor test, response surface methodology (RSM) was adopted to investigate the water content of the DES, liquid–solid ratio, extraction temperature, and extraction time as the four main factors influencing the extraction amount of myricetin (Y), denoted by A, B, C, and D. The different test levels for each factor were coded as −1, 0, and 1, and the Box–Behnken experimental design (BBD) was selected. To further optimise the extraction process and increase the precision of the experiment, five central points were selected, and 29 groups of experiments were conducted.39,40 The response surface design factor levels are listed in Table 2.
Table 2 Response surface design factor level table
Factors |
Level |
−1 |
0 |
1 |
Water content (%) |
10 |
20 |
30 |
Liquid–solid ratio (mL g−1) |
24 : 1 |
32 : 1 |
40 : 1 |
Extraction temperature (°C) |
60 |
70 |
80 |
Extraction time (min) |
30 |
40 |
50 |
3.4.2 Response surface test results. The test was conducted according to the response surface design described in Section 3.4.1, and the results are listed in Table 3.
Table 3 Response surface design results for extraction of myricetin from myrica rubra leaves
Run |
Water content/% |
Liquid–solid ratio/mL g−1 |
Extraction time/min |
Extraction temperature/°C |
Myricetin content mg g−1 |
1 |
−1 |
−1 |
0 |
0 |
13.49 |
2 |
−1 |
1 |
0 |
0 |
19.86 |
3 |
1 |
−1 |
0 |
0 |
13.05 |
4 |
1 |
1 |
0 |
0 |
17.26 |
5 |
0 |
0 |
−1 |
−1 |
15.83 |
6 |
0 |
0 |
−1 |
1 |
12.08 |
7 |
0 |
0 |
1 |
−1 |
11.60 |
8 |
0 |
0 |
1 |
1 |
18.53 |
9 |
−1 |
0 |
0 |
−1 |
11.30 |
10 |
−1 |
0 |
0 |
1 |
13.73 |
11 |
1 |
0 |
0 |
−1 |
12.58 |
12 |
1 |
0 |
0 |
1 |
13.82 |
13 |
0 |
−1 |
−1 |
0 |
12.19 |
14 |
0 |
−1 |
1 |
0 |
13.58 |
15 |
0 |
1 |
−1 |
0 |
16.32 |
16 |
0 |
1 |
1 |
0 |
15.63 |
17 |
−1 |
0 |
−1 |
0 |
14.55 |
18 |
−1 |
0 |
1 |
0 |
16.30 |
19 |
1 |
0 |
−1 |
0 |
14.20 |
20 |
1 |
0 |
1 |
0 |
17.15 |
21 |
0 |
−1 |
0 |
−1 |
17.74 |
22 |
0 |
−1 |
0 |
1 |
10.43 |
23 |
0 |
1 |
0 |
−1 |
12.64 |
24 |
0 |
1 |
0 |
1 |
20.10 |
25 |
0 |
0 |
0 |
0 |
21.11 |
26 |
0 |
0 |
0 |
0 |
20.03 |
27 |
0 |
0 |
0 |
0 |
20.91 |
28 |
0 |
0 |
0 |
0 |
21.26 |
29 |
0 |
0 |
0 |
0 |
22.67 |
3.4.3 Analysis of variance for response surface test results. The results in Table 3 were statistically analysed using SAS Release 8.01 TS Level 01MO, and the results are listed in Tables 4 and 5.
Table 4 Variance analysis of the modela
Source of variance |
Degree of freedom |
Sum of squares |
Mean square |
F-Value |
p-Value |
*the difference was significant, p < 0.05. |
A |
1 |
0.1141 |
0.1141 |
0.0536 |
0.8203 |
B |
1 |
37.9141 |
37.9141 |
17.8034 |
0.0009* |
C |
1 |
4.8387 |
4.8387 |
2.2721 |
0.1540 |
D |
1 |
4.0833 |
4.0833 |
1.9174 |
0.1878 |
A*A |
1 |
65.8038 |
65.8038 |
30.8996 |
0.0001* |
A*B |
1 |
1.1664 |
1.1664 |
0.5477 |
0.4715 |
A*C |
1 |
0.3600 |
0.3600 |
0.1690 |
0.6872 |
A*D |
1 |
0.3540 |
0.3540 |
0.1662 |
0.6896 |
B*B |
1 |
42.5126 |
42.5126 |
19.9627 |
0.0005* |
B*C |
1 |
1.0816 |
1.0816 |
0.5079 |
0.4878 |
B*D |
1 |
54.5382 |
54.5382 |
25.6096 |
0.0002* |
C*C |
1 |
62.3888 |
62.3888 |
29.2960 |
0.0001* |
C*D |
1 |
28.5156 |
28.5156 |
13.3901 |
0.0026* |
D*D |
1 |
106.3333 |
106.3333 |
49.9310 |
0.0001* |
Model |
14 |
314.0914 |
22.4351 |
10.5349 |
0.0001* |
Error |
14 |
29.8144 |
2.1296 |
|
|
Total |
28 |
343.9058 |
|
|
|
Table 5 Lack of fit test analysis
Source of variance |
Degree of freedom |
Sum of squares |
Mean square |
F-Value |
p-Value |
Lack of fit |
10 |
26.1889 |
2.6189 |
2.89 |
0.1591 |
Pure error |
4 |
3.6255 |
0.9064 |
|
|
Total error |
14 |
29.8144 |
2.1296 |
|
|
As listed in Table 4, model P < 0.0001, indicating that the regression model reached a significant statistical level of 0.01; equation determination factor R2 = 0.9133, that is, the variables explain 91.33% of the variation in the model; there are many factors influencing the process of extracting myricetin, factors not accounted for, and interactions between factors not accounted for, and those in the model can affect the regression equation.24,25 The model explained only 8.67% of the total variation, indicating that the equation had a high degree of fitness. This model can be used to analyse and predict the extraction process of myricetin from myricetin leaves. The t-test results for the model are listed in Table S1.†
The interaction terms B*D and C*D in Table 4 reached a significance level of 0.01, that is, the liquid–solid ratio, extraction time, and extraction temperature. The contours in (e) and (f) in Fig. 3 show a distinct ellipse; the long axis of the ellipse is near the diagonal, and the response surface shows a steep mountain range shape. The remaining contours are basically circular, response surface is peak-shaped, and interaction effect is not significant. If the liquid-to-material ratio or extraction temperature was changed during the experimental operation, the extraction time was adjusted accordingly. If the extraction time was changed, the liquid–solid ratio and extraction temperature were adjusted accordingly to obtain the best extraction effect. The contour map is depicted in Fig. S3.†
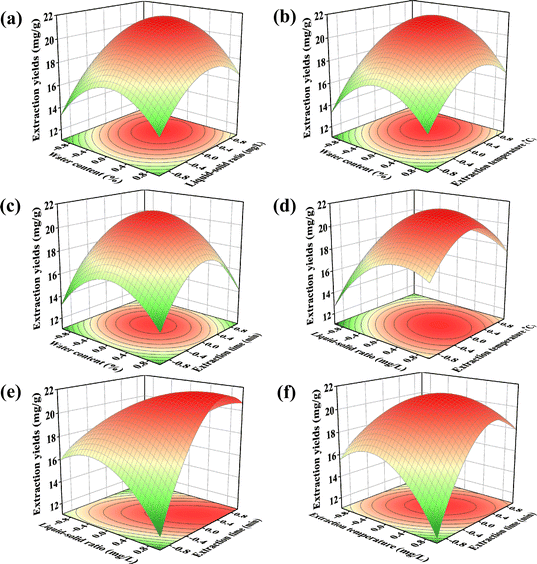 |
| Fig. 3 Response surface plots. | |
As summarized in Table 5, the lack-of-fit item P-value is 0.1591 > 0.05, indicating that the fitted regression model is consistent with the trend of data change, which is favourable to the model. Basically, there is a lack of fit items, and the regression equation can be used to analyse the experimental results instead of the real point of the experiment. Therefore, the model was better for the theoretical prediction of the amount of myricetin extracted from myricetin leaves in DES using the following quadratic regression equation:
Y = 21.196 − 0.0975 × A + 1.7775 × B + 0.635 × C + 0.5833 × D − 3.1851 × A × A − 0.5400 × A × B + 0.3 × A × A − 0.2975 × A × D − 2.5601 × B × B − 0.52 × B × C + 3.6925 × B × D − 3.1013 × C × C + 2.67 × C × D − 4.0488 × D × D |
The model predicted a maximum value of 21.99 and the maximum value is the point of stability. The predicted optimal conditions were: −0.081148, 0.659
076, 0.238
744, and 0.454
274, which are substituted back into Table 2, and the best conditions are obtained as follows: moisture content 19.1885%, liquid–solid ratio of 37.2716
:
1 mL g−1, extraction temperature of 72.3874 °C, and extraction time of 44.5428 min. Considering the operability of the experiment; the water content of 19%, liquid–solid ratio of 37
:
1 mL g−1, extraction temperature of 72 °C, and extraction time of 45 min were taken. Validation experiments were performed under these conditions and repeated thrice to obtain an extraction amount of 22.47 mg g−1.
3.5 Analysis and discussion of myricetin separation by macroporous resin
3.5.1 Adsorption rate of macroporous resin. The test was performed according to Section 2.4.2. As shown in Fig. 4a, the amount of adsorption decreased with an increase in the adsorption rate. This is because adsorption occurs at a certain rate and an extremely fast adsorption rate leads to a short residence time for myricetin in the liquid phase, which cannot be adsorbed onto the macroporous adsorption resin.18 The adsorption rate was low, adsorption time was long, and operating costs increased. In summary, an adsorption rate of 1 BV per h was the most suitable.
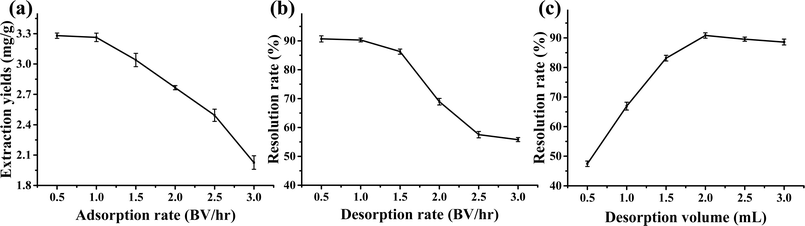 |
| Fig. 4 (a) Effect of adsorption rate on adsorption volume. (b) Effect of desorption rate on resolution rate. (c) Effect of desorption volume on the resolution rate. | |
3.5.2 Adsorption and desorption rate of macroporous resin. The test was conducted according to Section 2.4.3. As shown in Fig. 4b, the resolution rate decreased with an increase in the desorption rate. This is because the desorption speed was extremely high for myricetin in the adsorption column to be eluted in time, and the desorption rate was low. This is because the desorption rate was extremely high, the amount of myricetin in the adsorption column was less than that in the elution column, and the desorption rate was low. The desorption rate was low and myricetin was desorbed. Simultaneously, the desorption rate decreased, desorption time increased, and operating costs increased. On balance, a desorption rate of 1 BV per h is suitable.
3.5.3 Desorption volume of macroporous resin. The test was performed as described in Section 2.4.4, as shown in Fig. 4c. With an increase in the desorption volume, myricetin was continuously desorbed, and the desorption rate increased at a desorption volume of 2 BV. Subsequently, the desorption volume increased, and the desorption rate remained essentially unchanged. As the desorption volume increased, the required solvent and cost. Therefore, it was more appropriate to select a desorption volume of 2 BV.
3.5.4 Determination of sample purity. The experiment was carried out according to 2.4.6 and it was found that the purity of the purified samples of myricetin was 90.72%.
3.6 Antioxidant analysis and discussion
3.6.1 Antioxidant analysis and discussion of DPPH method. The test was conducted according to Section 2.5.1, as illustrated in Fig. 5a. With an increase in the concentration, the radical scavenging rate of myricetin on DPPH free radicals increased. At the same concentration, the radical scavenging rate of DPPH free radicals purified by the macroporous adsorption resin was higher than that of unpurified myricetin, and the radical scavenging rate of Vc was the lowest. An HPLC chromatogram of the extract is shown in Fig. S3.† The radical scavenging rate of DPPH radicals by myricetin tended to be unchanged after the concentration was greater than 8 μg mL−1, which was caused by the reaction between myricetin and DPPH free radicals reached equilibrium. Overall, myricetin had a better DPPH radical scavenging ability than Vc, and purified myricetin had better antioxidant effects.
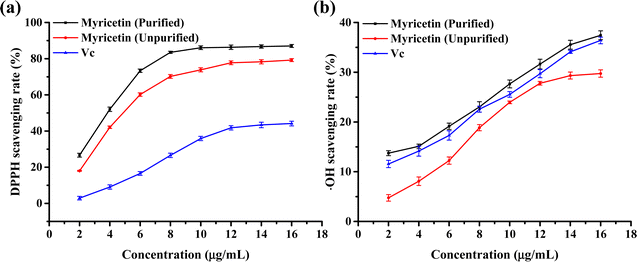 |
| Fig. 5 (a) Effect of concentration on DPPH free radical scavenging rate. (b) Effect of concentration on the scavenging rate of ˙OH radical. | |
3.6.2 ˙OH radical antioxidant analysis and discussion. The test was performed according to Section 2.5.2, as shown in Fig. 5b. With an increase in the concentration, the radical scavenging rate of myricetin for ˙OH free radicals increased as the myricetin concentration increased. The scavenging rate of –OH radicals at the same concentration was slightly greater for myricetin purified by the macroporous adsorption resin than that for Vc, and the lowest for unpurified myricetin. If myricetin is to be removed, it is better to separate and purify the myricetin extract using a macroporous adsorbent resin to obtain better antioxidant properties.
4 Conclusion
(1) A DES-5 (choline chloride–oxalic acid) system with a water content of 19% was used to extract myricetin. With a liquid–solid ratio of 37
:
1 mL g−1, an extraction time of 45 min and extraction temperature of 72 °C was a suitable DES extraction process for myricetin. Under these conditions, the extraction amount of myricetin was 22.47 mg g−1.
(2) Adsorption of myricetin extracted from DES using the AB-8 macroporous adsorbent resin. Desorption using anhydrous ethanol was performed under optimal conditions with an adsorption rate of 1 BV per h, desorption rate of 1 BV per h and desorption volume of 2 BV.
(3) The antioxidant effects of myricetin after purification and crude extraction were studied using an in vitro antioxidant method and the scavenging effects of myricetin on DPPH and ˙OH free radicals were investigated. The results showed that with an increase in myricetin concentration, the scavenging rates of DPPH free radicals and ˙OH free radicals increased. Compared to Vc, myricetin has a better scavenging ability for DPPH free radicals, and purified myricetin has a better antioxidant effect. The scavenging rate of –OH radicals at the same concentration was slightly greater for myricetin purified by the macroporous adsorption resin than that of Vc and the lowest for unpurified myricetin. Myricetin was purified using a macroporous adsorption resin to improve its antioxidant properties.
Author contributions
Junhai Liu: conceptualization, methodology, investigation, visualization, writing – original draft, writing – review and editing, project administration, funding acquisition. Xiaosha Guo: data curation, methodology, investigation, formal analysis. Qiaowei Miao: investigation, data curation, validation. Xiaohui Ji: data curation, supervision, validation. Yinku Liang: data curation, validation. Tianjiao Tong: investigation, data curation, supervision.
Conflicts of interest
The authors declare that they have no known competing financial interests or personal relationships that could have appeared to influence the work reported in this paper.
Acknowledgements
This research was funded by the Key R & D Projects of Shaanxi Province (2023-YBSF-017) Qinba Biological Resources and Ecological Environment Provincial and Ministerial Co-Construction National Key Laboratory (Cultivation) “City School Co-Construction” Scientific Research Special Project (SXC-2110), and Science and Technology Fund Plan Project of Hanzhong City, Shaanxi Province in 2022 (HZKJGG-13).
Notes and references
- M. Xing, Y. Cao, C. Ren, Y. Liu, J. Li, D. Grierson, C. Martin, C. Sun, K. Chen, C. Xu and X. Li, Plant J., 2021, 108, 411–425 Search PubMed.
- D. K. Semwal, R. B. Semwal, S. Combrinck and A. Viljoen, Nutrients, 2016, 8, 90 Search PubMed.
- A. Giacosa, R. Barale, L. Bavaresco, M. A. Faliva, V. Gerbi, C. L. Vecchia, E. Negri, A. Opizzi, S. Perna, M. Pezzotti and M. Rondanelli, Crit. Rev. Food Sci. Nutr., 2016, 56, 635–640 Search PubMed.
- R. Bridi, E. Atala, P. N. Pizarro and G. Montenegro, J. Nat. Prod., 2019, 82, 559–565 Search PubMed.
- J. O. Airouyuwa, H. Mostafa, A. Riaz and S. Maqsood, Ultrason. Sonochem., 2022, 91, 106233 CrossRef PubMed.
- K. J. Lanjekar, S. Gokhale and V. K. Rathod, Bioresour. Technol., 2022, 18, 101074 CrossRef CAS.
- M. Zhong, S. Huang, H. W. J. Xu and L. Zhang, RSC Adv., 2019, 9, 1576–1585 RSC.
- C. Xing, W. Q. Cui, Y. Zhang, X. S. Zou, J. Y. Hao, S. D. Zheng, T. T. Wang, X. Z. Wang, T. Wu, Y. Y. Liu, X. Y. Chen, S. G. Yuan, Z. Y. Zhang and Y. H. Li, Ultrason. Sonochem., 2022, 83, 105946 CrossRef CAS PubMed.
- W. Wang, M. An, G. Zhao, Y. Wang, D. Zhang, L. Zhao, J. Han, G. Wu and Y. Bo, Microchem. J., 2023, 193, 108952 CrossRef CAS.
- M. C. Ali, J. Chen, H. Zhang, Z. Li, L. Zhao and H. Qiu, Talanta, 2019, 203, 16–22 CrossRef CAS PubMed.
- M. de los Ángeles Fernández, J. Boiteux, M. Espino, F. Gomez and M. Fernanda Silva, Anal. Chim. Acta, 2018, 1038, 1–10 CrossRef PubMed.
- S. C. Cunha and J. O. Fernandes, Trends Anal. Chem., 2018, 105, 225–239 CrossRef CAS.
- F. Li, L. Xiao, X. Lin, J. Dai, J. Hou and L. Wang, Foods, 2023, 12, 1872 Search PubMed.
- L. Wu, Z. Chen, S. Li, L. Wang and J. Zhang, Sep. Purif. Technol., 2021, 262, 118339 CrossRef CAS.
- J. A. R. de Oliveira, P. de Paula Menezes Barbosa and G. A. Macêdo, Foods, 2022, 11, 3205 Search PubMed.
- H. Du, Y. Liu, Z. Yan, Z. Hu, G. Liu and C. Wu, Xiandai Shipin Keji, 2021, 37, 1–8 Search PubMed.
- L. Wang, Y. Wang, M. Chen, Y. Zhu, Y. Qin and Y. Zhou, RSC Adv., 2022, 12, 28659–28676 RSC.
- X. Liu and F. Li, Biomed. Chromatogr., 2023, e5639 CrossRef CAS PubMed.
- C. Liu, L. Qiao, Q. Gao, F. Zhang, X. Zhang, J. Lei, M. Ren, S. Xiao, J. Kuang, S. Deng, X. Yuan, Y. Jiang and G. Wang, Ultrason. Sonochem., 2023, 98, 106491 CrossRef CAS PubMed.
- R. Rashid, S. M. Wani, S. Manzoor, F. A. Masoodi and M. Masarat Dar, Food Chem., 2023, 398, 133871 CrossRef CAS PubMed.
- P. Wei, Y. Zhang, Y. Y. Wang, J. F. Dong, B. N. Liao, Z. C. Su, W. L, J. C. Xu, W. Y. Lou, H. H. Su and C. Peng, Biomass Convers. Biorefin., 2023, 13, 15095–15109 CAS.
- B. Roman, A. Muzykiewicz-Szymańska, P. Ossowicz-Rupniewska, A. Klimowicz and E. Janus, RSC Adv., 2021, 11, 25983–25994 Search PubMed.
- Y. J. Tan, G. S. Zhou, S. Guo, H. Yan, J. Zhang, Z. H. Zhu, X. Q. Shi, S. J. Yu, Y. P. Tang, S. L. Huang, G. P. Peng and J. A. Duan, RSC Adv., 2018, 8, 40748–40759 RSC.
- K. A. Avilés-Betanzos, J. V. Cauich-Rodríguez, M. González-Ávila, K. Morozova, M. O. Ramírez-Sucre and I. M. Rodríguez-Buenfil, Processes, 2023, 11, 1729 Search PubMed.
- T. P. Vo, N. D. Pham, T. V. Pham, H. Y. Nguyen, L. T. V. Vo, T. N. H. Tran, T. Tran and D. Q. Nguyen, Heliyon, 2023, 9, e14884 CrossRef CAS PubMed.
- Y. Xie, Q. S. Guo and G. S. Wang, Molecules, 2016, 21, 768 CrossRef PubMed.
- D. Yang, M. M. Li, W. Wang, G. D. Zheng, Z. P. Yin, J. G. Chen and Q. F. Zhang, LWT, 2022, 161, 113371 Search PubMed.
- J. Ren, L. Liao, S. Shang, Y. Zheng, W. Sha and E. Yuan, J. Food Sci., 2019, 84, 667–677 Search PubMed.
- Y. Zhang, L. Kong, C. Yin, D. Jiang, J. Jiang, J. He and W. Xiao, LWT, 2013, 51, 343–347 Search PubMed.
- Y. Li, J. Liu, R. Cao, S. Deng and X. Lu, J. Chem. Eng. Data, 2013, 58, 2527–2537 CrossRef CAS.
- Q. Fan, W. Luo, L. Liu, L. Zhao, H. Liu, W. Zi and X. Chen, Food Res. Dev., 2022, 43, 117–122 Search PubMed.
- W. Li, H. Wu, B. Liu, X. Hou, D. Wan, W. Lou and J. Zhao, J. Biotechnol., 2015, 199, 31–37 Search PubMed.
- G. Li, J. Lei, S. Li, Y. Jiang, F. Zhang, C. Song, S. Xiao, S. Fu, J. Zhou, F. Wu and G. Wang, RSC Adv., 2022, 12, 26975–26988 Search PubMed.
- J.-Y. Yeh, L.-H. Hsieh, K.-T. Wu and C.-F. Tsai, Molecules, 2011, 16, 3197–3211 CrossRef CAS PubMed.
- L. Wang, X. Fang, Y. Hu, Y. Zhang, Z. Qi, J. Li and L. Zhao, RSC Adv., 2021, 11, 17924–17935 RSC.
- K. Xu, Y. Wang, Y. Huang, N. Li and Q. Wen, Anal. Chim. Acta, 2015, 864, 9–20 CrossRef CAS PubMed.
- X. Shang, J.-N. Tan, Y. Du, X. Liu and Z. Zhang, Molecules, 2018, 23, 2110 CrossRef PubMed.
- T. Ahmed, M. R. Rana, M. A. Hossain, S. Ullah and M. Suzauddula, Biomass Convers. Biorefin., 2023, 1–15 Search PubMed.
- L. Wu, L. Li, S. Chen, L. Wang and X. Lin, Sep. Purif. Technol., 2020, 247, 117014 CrossRef CAS.
- N. Tsvetov, O. Paukshta, N. Fokina, N. Volodina and A. Samarov, Molecules, 2023, 28, 912 CrossRef CAS PubMed.
|
This journal is © The Royal Society of Chemistry 2024 |
Click here to see how this site uses Cookies. View our privacy policy here.