DOI:
10.1039/D4RA01785D
(Paper)
RSC Adv., 2024,
14, 15413-15418
Ultrasmall copper nanoclusters as an efficient antibacterial agent for primary peritonitis therapy†
Received
8th March 2024
, Accepted 27th April 2024
First published on 13th May 2024
Abstract
The urgent need to develop biocompatible, non-resistant antibacterial agents to effectively combat Gram-negative bacterial infections, particularly for the treatment of peritonitis, presents a significant challenge. In this study, we introduce our water-soluble Cu30 nanoclusters (NCs) as a potent and versatile antibacterial agent tailored for addressing peritonitis. The as-synthesized atomically precise Cu30 NCs demonstrate exceptional broad-spectrum antibacterial performance, and especially outstanding bactericidal activity of 100% against Gram-negative Escherichia coli (E. coli). Our in vivo experimental findings indicate that the Cu30 NCs exhibit remarkable therapeutic efficacy against primary peritonitis caused by E. coli infection. Specifically, the treatment leads to a profound reduction of drug-resistant bacteria in the peritoneal cavity of mice with peritonitis by more than 5 orders of magnitude, along with the resolution of pathological features in the peritoneum and spleen. Additionally, comprehensive in vivo biosafety assessment underscores the remarkable biocompatibility, low biotoxicity, as well as efficient hepatic and renal clearance of Cu30 NCs, emphasizing their potential for in vivo application. This investigation is poised to advance the development of novel Cu NC-based antibacterial agents for in vivo antibacterial treatment and the elimination of abdominal inflammation.
1. Introduction
Bacterial infections pose a significant threat to global public health and human well-being, with approximately 700
000 people succumbing to drug-resistant bacterial infections annually, according to the World Health Organization.1 Although antibiotics have historically been effective in combating bacterial infections, the widespread overuse and abuse of these drugs have escalated the issue of bacterial resistance.2–5 Notably, different from the situation that Gram-positive bacteria could be easily treated by novel drugs targeting Gram-positive bacteria in clinical trials, the unique structure of Gram-negative bacteria, and their proclivity for transmitting drug resistance have significantly impeded the development of new antibiotics targeting these bacteria over the last two decades.6,7 This has rendered the treatment of Gram-negative infections particularly challenging.4,8 Gram-negative bacterial infections can be classified into local and systemic infections according to the different infection sites. Local infections are typically confined to the skin or organs, while systemic infections are commonly associated with abdominal cavity or bloodstream infections. Among these infections, primary peritonitis, a serious systemic infection caused by Gram-negative bacteria in the abdominal cavity, merits particular attention. Without timely treatment, peritonitis can precipitate systemic multiple organ failure, septic shock, and death.9–11 Therefore, the pressing need to develop new, non-resistant antibacterial agents to combat Gram-negative bacterial infections, notably to effectively manage peritonitis, cannot be overstated.12
In recent years, there has been a surge in the development of ultrasmall coinage metal nanoclusters (NCs; e.g., Au and Ag) with a core size of <3 nm,13–15 which have gained widespread acceptance in various biomedical applications such as bioimaging,16,17 drug delivery,18 disease theranostic,19 and broad-spectrum antibacterial.1 Metal NCs exhibit atomically precise size,20,21 molecule-like luminescence,22–26 good biocompatibility,27 size/structure-dependent physicochemical properties,28–30 strong antibacterial activity,31,32 and no drug resistance,1 rendering them effective in diverse antibacterial applications.1,19,31,33 Notably, a series of Au and Ag NCs-based antibacterials have demonstrated either long-lasting photodynamic antibacterial performance or intrinsic antibacterial activity,31,32 thereby promoting the healing of bacteria-infected wounds.34,35 However, previous studies on metal NCs-based antibacterials have predominantly focused on the treatment of local infections, neglecting systemic ones. Moreover, the limited availability of Au and Ag on Earth hinders their widespread utilization in cost-effective antibacterial applications. Conversely, copper (Cu), belonging to the same IB group as Au and Ag and being abundant, presents a promising alternative for developing affordable yet efficient metal NCs-based antibacterial agents.33,36 Nevertheless, the easy oxidation of Cu and the infeasibility of previously used synthetic strategies for Au and Ag NCs pose challenges in the synthesis of atomically precise water-soluble Cu NCs. Consequently, the development of low-cost water-soluble Cu NCs-based antibacterial agents and their application to systemic infections, such as peritonitis caused by Gram-negative bacteria, hold considerable potential for research and clinical value, thereby motivating this project.
In this study, we present our design of water-soluble Cu30 NCs as a potent and versatile antibacterial agent for the effective treatment of peritonitis (Scheme 1). The synthesized Cu30 NCs exhibit a monodisperse size at the atomic level, and have demonstrated outstanding broad-spectrum antibacterial activity. They have been shown to achieve bactericidal rates of more than 99% against both Gram-negative Escherichia coli (E. coli), Gram-positive Staphylococcus aureus (S. aureus), and the fungus Candida albicans, and >97% against obstinate methicillin-resistant S. aureus (MRSA). Moreover, the in vivo evaluation of Cu30 NCs, administered through intraperitoneal injection, against primary peritonitis caused by Gram-negative E. coli has shown their efficacy for peritonitis treatment and reducing inflammation in bacterial infections. Furthermore, both in vitro and in vivo studies have revealed the good biocompatibility of the Cu30 NCs, emphasizing their suitability for combatting systemic infections. To the best of our knowledge, this study represents the first successful application of ultrasmall Cu NCs-based antibacterial agents in the treatment of primary peritonitis.
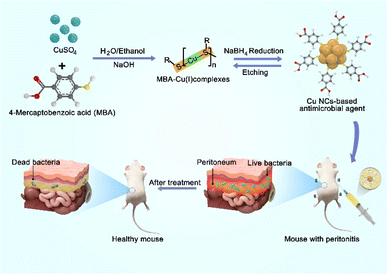 |
| Scheme 1 Schematic illustration of the synthesis of Cu30 NCs and their antibacterial application in treating primary peritonitis. | |
2. Result and discussion
2.1 Synthesis and characterization of Cu30 NCs
The synthesis of Cu30 NCs in this study was accomplished using the previously reported “NaOH-mediated NaBH4 reduction” method. It should be mentioned that optimal pH and solvent polarity are the key parameters to obtain monodisperse Cu30 NCs. Specifically, NaOH was found to modulate the reduction ability of NaBH4 by impeding its self-hydrolysis, while concurrently heightening the etching ability of thiolate ligands by deprotonating free ligands in an alkaline environment. Additionally, the mixed water/ethanol solvent was instrumental in fine-tuning the size and structure of MBA–Cu(I) complexes. Therefore, the absence of NaOH and ethanol led to the formation of polydisperse products.37 As shown in Scheme 1, the Cu precursor CuSO4, the protecting ligand 4-mercaptobenzoic acid (MBA), and NaOH were mixed in a water/ethanol solution (containing 35 vol% ethanol) under stirring condition to form MBA–Cu(I) complexes. Subsequently, a reducing agent (NaBH4) was slowly introduced to reduce the MBA–Cu(I) complexes and promote a rapid equilibrium between the forward reduction reaction and the reverse etching reaction,38 resulting in the formation of yellow atomically-precise Cu30(MBA)16 NCs within 4 h, as illustrated in the inset of Fig. 1a. The synthesized MBA-protected Cu NCs were characterized, and the results revealed an optical absorption peak at 380 nm in the UV-vis absorption spectrum (Fig. 1a). This observation effectively ruled out the production of large-sized Cu nanoparticles (∼640 nm) and indicated the formation of monodisperse Cu NCs.39 Furthermore, the transmission electron microscope (TEM) image revealed a core size of ∼1.12 nm for the synthesized Cu NCs, suggesting the formation of ultrasmall Cu NCs (Fig. 1b). The natural polyacrylamide gel electrophoresis (PAGE) analysis of Cu NCs (Fig. 1c) disclosed a single band, demonstrating the excellent monodispersity of the Cu NCs. Notably, size analysis of this type of Cu NCs was conducted with atomic precision in our previous study. Based on the results from electrospray ionization mass spectrometry,37 their formula was assigned to be Cu30(MBA)16 NCs.
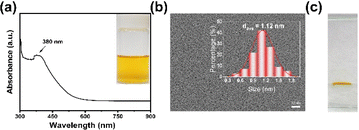 |
| Fig. 1 Characterization of Cu30 NCs. (a) UV-vis absorption spectrum of Cu30 NCs in 35 vol% of ethanol. The inset shows digital photograph of Cu30 NCs solution. (b) TEM image and size distribution histogram (inset) of Cu30 NCs. (c) PAGE result of Cu30 NCs. | |
2.2 In vitro antimicrobial activity and cytotoxicity of Cu30 NCs
The Cu30 NCs showed excellent antibacterial activity against both Gram-negative and Gram-positive bacteria. As indicated in Fig. 2a, Cu30 NCs significantly inhibited the growth of E. coli and S. aureus in the culture medium compared to the PBS control group. Particularly, Cu NCs with a total concentration of 10 μM (based on Cu ions) exhibited a high antibacterial performance against E. coli and S. aureus, with antibacterial rates of 100% and 99.17 ± 1.21%, respectively, as shown in Fig. 2b. In addition, we evaluated the antimicrobial effectiveness of Cu30 NCs against MRSA and Candida albicans (Fig. S1 and S2†). The findings demonstrated that the Cu30 NCs with 20 μM concentration exhibited a 97.89 ± 2.94% antibacterial rate against MRSA and a 99.81 ± 1.19% antifungal rate against Candida albicans under the same testing conditions. The remarkable antimicrobial efficacy of Cu30 NCs can be attributed to their ultrasmall size and high surface-to-volume ratio, as well as the inherent antibacterial properties of Cu species. These characteristics significantly enhance atomic utilization and antimicrobial performance. Additionally, the as-designed Cu30 NCs simultaneously possess ultrasmall size, excellent antibacterial performance, and cost-effectiveness compared to other antibacterial agents (i.e., silver–gallium nano-amalgamated particles and polycationic silver NCs),12,15 increasing the acceptance in peritonitis treatment.
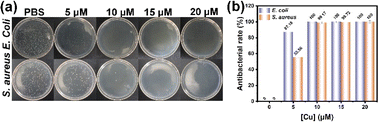 |
| Fig. 2 (a) In vitro antibacterial results of Cu30 NCs with a concentration range of 5–20 μM against E. coli and S. aureus with the usage of PBS as control. (b) Antibacterial rates of Cu30 NCs against E. coli and S. aureus. | |
Upon treatment with Cu30 NCs, the cell morphology of E. coli and S. aureus undergoes a noticeable transformation from a plump and smooth appearance (Fig. S3a and S4a†) to an atrophic and wrinkled state (Fig. S3b and S4b†), indicating the disruption of the cell membrane. To gain a deeper insight into the antibacterial mechanism, two experiments were conducted. Initially, the cell uptake behavior of E. coli was investigated by measuring the optical absorbance of Cu30 NCs before and after incubation with E. coli. The results in Fig. S5† revealed a significant decrease in the optical absorbance of Cu30 NCs at 380 nm from 2.94 to 1.67 after the separation of E. coli from the Cu30 NCs solution via centrifugation, suggesting that 46.3% of the Cu30 NCs were absorbed on the membrane of E. coli or endocytosed by the bacterium. Subsequently, transmission electron microscope (TEM) analysis was employed to examine the cell uptake behavior of E. coli for Cu30 NCs, with E. coli cells incubated with Cu30 NCs being fixed prior to TEM analysis. Fig. S6† illustrated clear cell morphology with a fractured membrane of E. coli, along with numerous ultra-tiny black dots (Cu30 NCs) observed both on the cell membrane and within the cell. Based on this data, it can be inferred that the absorption and internalization of Cu30 NCs by E. coli may trigger the generation of ROS, leading to oxidative stress, subsequent ROS production, and eventual destruction of the cell membrane, resulting in the inactivation or dysfunction of the bacteria.31,33 It is worth noting that the peptidoglycan membrane of Gram-negative E. coli is thinner compared to Gram-positive S. aureus,40 rendering the former more susceptible to inactivation. Therefore, this disparity may contribute to the superior antibacterial effectiveness of Cu30 NCs against Gram-negative bacteria in comparison to Gram-positive counterparts.
To assess the biological applications, the cytotoxicity of Cu30 NCs against 4T1 cells was examined using an MTT assay (Fig. S7†). The results revealed that the cytotoxicity of Cu30 NCs (12.5 μM) was low, with the 4T1 cells retaining a viability of 96.12% after 24 h, compared to the positive control (ultrapure water) and significantly higher than the negative control (Tween 20, 6.92% cell viability). These results indicate the good biocompatibility of the Cu30 NCs.
2.3 In vivo treatment of primary peritonitis with Cu30 NCs
The Cu30 NCs have exhibited a promising in vitro antimicrobial effect, low cytotoxicity, and good biocompatibility, providing strong justification for further investigation of their potential efficacy for treating in vivo peritonitis with antibacterial and anti-inflammatory effects. Initial experiments depicted in Fig. 3a involved the establishment of a mouse model of primary peritonitis through intraperitoneal injection of E. coli suspended in saline. Notably, the concentration of E. coli required to induce 100% mortality in mice after 2 days was found to be 5 × 107 CFU mL−1, as illustrated in Fig. 3b. Consequently, this concentration was utilized in subsequent peritonitis induction experiments. Within 3 h post E. coli injection, mice exhibited symptoms characteristic of primary peritonitis such as depression, moodiness, curling up into a ball, and exclusion of mucus feces. Subsequently, mice afflicted with peritonitis were injected with Cu30 NCs in 100 μL saline with varied concentrations, while a saline group served as a control. Fig. 3c illustrates the outcomes where infected mice faced mortality within 120 h when treated with Cu30 NCs below 0.5 mM. Conversely, administering Cu30 NCs at concentrations of ≥0.6 mM resulted in mice surviving normally at 120 h, indicating that the minimum effective concentration for successful primary peritonitis therapy is 0.6 mM. Interestingly, even with a threefold increase in concentration (1.8 mM), all mice maintained a 100% survival rate, suggesting a wide therapeutic window. This result can be ascribed to the outstanding biocompatibility, low biotoxicity, and effective therapeutic action of Cu30 NCs. Thereafter, the efficacy of 0.6 mM Cu30 NCs in treating primary peritonitis was evaluated.
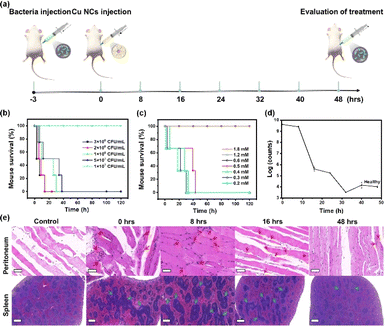 |
| Fig. 3 In vivo evaluation of the therapeutic effect of Cu30 NCs on peritonitis in mice. (a) Schematic illustration for the animal experimentation timeline. (b) Survival rate of mice after injection with different concentrations of E. coli. (c) Survival rate of peritonitis mice after injection with different concentrations of Cu30 NCs. (d) Bacterial quantity within the peritoneal cavity of mice after treatment with Cu30 NCs for different time. (e) H&E staining of histological sections including spleen and peritoneum of mice with acute peritonitis after treatment with Cu30 NCs for different time. The increasing inflammatory cells on peritoneum and the enlarged lymphoid nodules on spleen are marked with red and blue arrows, respectively. The normal mice without any infection were used as a control group. Scale bar, 50 μm (peritoneum group), 500 μm (spleen group). | |
The Cu30 NCs demonstrated notable effectiveness in treating in vivo primary peritonitis infected with E. coli. Fig. 3d and S8† illustrated the rapid reduction of E. coli in the peritoneal cavity of mice following Cu30 NCs administration, with a subsequent gradual decrease towards normal levels over time. Significantly, the bacterial counts decrease by more than 5 orders of magnitude at 48 h compared to their initial population, indicating the strong bactericidal properties of Cu30 NCs within organisms. Further validation of the in vivo therapeutic effect of Cu30 NCs was observed through hematoxylin–eosin (H&E) staining (Fig. 3e and S9†). Compared with the control mice with the injection of saline rather than E. coli, the peritonitis model exhibited increased inflammatory cells (marked with red arrows, see upper panel in Fig. 3e and S9†) in the peritoneum post-bacterial infection, as well as enlarged splenic lymph nodes (labelled with blue arrows, see lower panel in Fig. 3e and S9†). Fig. S10† displayed the detailed size information of the inflammatory cells and splenic lymph nodes.11 Notably, the number of inflammatory cells in the peritoneum of the peritonitis mice group increased, peaked at 8 h from the intraperitoneal injection of Cu30 NCs, and subsequently decreased to normal levels by 48 h. Similarly, splenic lymph nodes reached maximum extension at 8 h from the intraperitoneal injection of Cu30 NCs, declining significantly by 24 h and returning to normal levels at 40 h. The conclusion that Cu30 NCs have anti-inflammatory effects is strengthened by the expression levels of anti-inflammatory factors in mice,41,42 as depicted in Fig. S11.† Specifically, the expression levels of tumor necrosis factor-α (TNF-α, Fig. S11a†), interleukin-6 (IL-6, Fig. S11b†), and interleukin-1β (IL-1β, Fig. S11c†) in the Cu30 NCs-treated group gradually decreased to levels within the normal range observed in uninfected mice. These observations collectively substantiate the remarkable therapeutic effects of Cu NCs against drug-resistant bacterial infections and primary peritonitis. Furthermore, the as-designed Cu30 NCs showed better therapeutic performance than ampicillin, a commercial antibacterial agent, in peritonitis treatment, which is evidenced by their dose–response curves (Fig. S12†) and in vivo antibacterial activities (Fig. S13†), emphasizing the huge potential of Cu30 NCs in clinical peritonitis treatment.
2.4 In vivo biosafety assessment of Cu30 NCs
The in vivo biosafety of Cu30 NCs is a significant concern, despite the strong confirmation of their superior therapeutic efficacy for primary peritonitis as demonstrated above. To address this concern, H&E staining was carried out on major organs, including the heart, liver, spleen, lungs, and kidneys, in Cu30 NCs-treated mice five days after the injection of Cu30 NCs. This was compared with the normal mice as a control, as depicted in Fig. 4. The results revealed that there was no observable histopathological damage or abnormality in the major organs of the mice. Furthermore, blood routine analysis and blood biochemistry tests indicated that all the blood indexes, as well as the liver and kidney functions, were within the normal range (Table 1). These findings clearly showed that Cu30 NCs are safe and compatible for use in peritonitis therapy, demonstrating their promising potential for in vivo application.
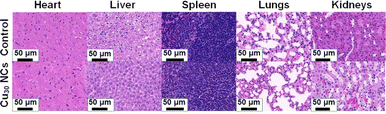 |
| Fig. 4 H&E staining of histological sections including heart, liver, spleen, lungs and kidneys of peritonitis mice 5 days post injection with Cu30 NCs for in vivo biosafety evaluation. The normal mice without any infection were used as a control group. | |
Table 1 Blood routine and liver function parameters for peritonitis mice 5 days post injection with Cu30 NCs
Name |
White blood cell (109 L−1) |
Monocytes (109 L−1) |
Neutrophil (109 L−1) |
Alkaline phosphatase (U L−1) |
Total protein (g L−1) |
Alanine aminotransferase (U L−1) |
Numerical value |
2.9 |
0.2 |
0.9 |
157.50 |
54 |
34.2 |
Normal range |
0.8–6.8 |
0.0–0.3 |
0.1–1.8 |
62–209 |
36–66 |
28–132 |
The in vivo distribution and metabolism of Cu30 NCs also played a crucial role in biosafety. Fig. 5 revealed that Cu30 NCs were present in all organs of the mice, with varying quantities. Specifically, initial increments followed by subsequent decreases in Cu30 NCs levels were observed in the heart, lungs, spleen, and peritoneum within 48 h, indicating non-accumulation in these organs. Conversely, the levels of Cu30 NCs in the kidneys and liver exhibited an “increase–decrease–increase” pattern within 48 h, signifying a tendency towards accumulation. Furthermore, the gradual decrease in Cu30 NCs content in the heart, lungs, spleen, and peritoneum within 30 h, in conjunction with the increase in the kidneys and liver, provided clear evidence that the clearance of Cu30 NCs is dependent on the metabolic functions of the liver and kidneys. This observation is consistent with the role of the liver and kidneys as crucial organs for metabolic and detoxification processes in living organisms.
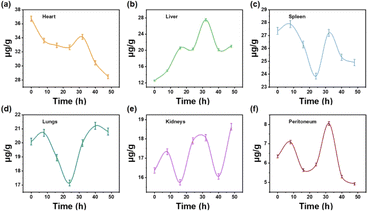 |
| Fig. 5 Time-course biodistribution of Cu30 NCs in different organs of mice: (a) heart, (b) liver, (c) spleen, (d) lungs, (e) kidneys, and (f) peritoneum. | |
3. Conclusions
In summary, water-soluble Cu30 NCs have been developed as an effective antibacterial agent for treating primary peritonitis. The ultrasmall size of Cu30 NCs (<1.2 nm) and their excellent antibacterial properties against Gram-negative E. coli (100%) have contributed to their remarkable therapeutic effects. Moreover, the treatment with Cu30 NCs resulted in a reduction of more than 5 orders of magnitude in the number of drug-resistant E. coli in the peritoneal cavity of mice with peritonitis and led to the disappearance of pathological features in the peritoneum and spleen. Importantly, no significant toxicities were observed in the group of mice treated with Cu30 NCs during the tested period, demonstrating their good biocompatibility, low biotoxicity, as well as hepatic and renal clearance. This study is significant in that it sheds light on the design of novel Cu NCs-based antibacterial agents for in vivo antibacterial treatment and the elimination of abdominal inflammation.
Conflicts of interest
The authors declare no conflict of interests.
Acknowledgements
This work was supported by the National Natural Science Foundation of China (22071127, 22371153, 52301002), the China Postdoctoral Science Foundation (2023M731854), the Taishan Scholar Foundation of Shandong Province (tsqn201812074), the Qingdao Postdoctoral Science Foundation (QDBSH20230101003), and the Youth Innovation Team Development Program of Shandong Higher Education Institutions (2022KJ155).
Notes and references
- K. Zheng, M. I. Setyawati, D. T. Leong and J. Xie, Coord. Chem. Rev., 2018, 357, 1–17 CrossRef CAS.
- F. Jiao, W. Zhao, W. Zhao, Y. Wang, Y. Deng, S. Chang, J. Sun, Q. Lou, L. Wang, C.-X. Shan, Y. Xiao and L. Dong, BMEMat, 2023, 1, e12055 CrossRef.
- B. McCall, L. Shallcross, M. Wilson and A. Hayward, npj Antimicrob. Resist., 2023, 1, 10 CrossRef.
- E. R. Rojas, G. Billings, P. D. Odermatt, G. K. Auer, L. Zhu, A. Miguel, F. Chang, D. B. Weibel, J. A. Theriot and K. C. Huang, Nature, 2018, 559, 617–621 CrossRef CAS PubMed.
- R. Laxminarayan, A. Duse, C. Wattal, A. K. M. Zaidi, H. F. L. Wertheim, N. Sumpradit, E. Vlieghe, G. L. Hara, I. M. Gould, H. Goossens, C. Greko, A. D. So, M. Bigdeli, G. Tomson, W. Woodhouse, E. Ombaka, A. Q. Peralta, F. N. Qamar, F. Mir, S. Kariuki, Z. A. Bhutta, A. Coates, R. Bergstrom, G. D. Wright, E. D. Brown and O. Cars, Lancet Infect. Dis., 2013, 13, 1057–1098 CrossRef.
- R. Ruhal and R. Kataria, Microbiol. Res., 2021, 251, 126829 CrossRef CAS PubMed.
- D. Saxena, R. Maitra, R. Bormon, M. Czekanska, J. Meiers, A. Titz, S. Verma and S. Chopra, npj Antimicrob. Resist., 2023, 1, 17 CrossRef.
- H. C. Neu, Science, 1992, 257, 1064–1073 CrossRef CAS PubMed.
- D. Pörner, S. Von Vietinghoff, J. Nattermann, C. P. Strassburg and P. Lutz, Expert Opin. Pharmacother., 2021, 22, 1567–1578 CrossRef PubMed.
- S. M. Manani, G. M. Virzì, A. Giuliani, M. Baretta, V. Corradi, M. De Cal, C. Biasi, C. Crepaldi and C. Ronco, Blood Purif., 2020, 49, 434–439 CrossRef PubMed.
- Y. Wang, X. Ding, Y. Chen, M. Guo, Y. Zhang, X. Guo and H. Gu, Biomaterials, 2016, 101, 207–216 CrossRef CAS PubMed.
- T. T. Nguyen, P. Zhang, J. Bi, N. H. Nguyen, Y. Dang, Z. Xu, H. Wang, N. Ninan, R. Bright, T. Pham, C. K. Nguyen, Y. Sabri, M. T. Nguyen, J. Vongsvivut, Y. Zhao, K. Vasilev and V. K. Truong, Adv. Funct. Mater., 2023, 23, 10539 Search PubMed.
- X. Wang, B. Yin, L. Jiang, C. Yang, Y. Liu, G. Zou, S. Chen and M. Zhu, Science, 2023, 381, 784–790 CrossRef CAS PubMed.
- Q. Yao, X. Yuan, T. Chen, D. T. Leong and J. Xie, Adv. Mater., 2018, 30, 1802751 CrossRef.
- E. O. Uroro, R. Bright, P. R. L. Dabare, J. Y. Quek, N. Goswami and K. Vasilev, Mater. Today Chem., 2023, 28, 101376 CrossRef CAS.
- S. Li, J. Wei, Q. Yao, X. Song, J. Xie and H. Yang, Chem. Soc. Rev., 2023, 52, 1672–1696 RSC.
- W. Zhong, K. Liang, W. Liu and L. Shang, Chem. Sci., 2023, 14, 8823–8830 RSC.
- G. Yang, X. Pan, W. Feng, Q. Yao, F. Jiang, F. Du, X. Zhou, J. Xie and X. Yuan, ACS Nano, 2023, 17, 15605–15614 CrossRef CAS PubMed.
- G. Yang, Z. Wang, F. Du, F. Jiang, X. Yuan and J. Y. Ying, J. Am. Chem. Soc., 2023, 145, 11879–11898 CrossRef CAS.
- X. Zhu, L. Chen, Y. Liu and Z. Tang, Polyoxometalates, 2023, 2, 9140031 CrossRef.
- Q. Yao, L. Liu, S. Malola, M. Ge, H. Xu, Z. Wu, T. Chen, Y. Cao, M. F. Matus, A. Pihlajamäki, Y. Han, H. Häkkinen and J. Xie, Nat. Chem., 2023, 15, 230–239 CrossRef CAS PubMed.
- S.-S. Zhang, S. Havenridge, C. Zhang, Z. Wang, L. Feng, Z.-Y. Gao, C. M. Aikens, C.-H. Tung and D. Sun, J. Am. Chem. Soc., 2022, 144, 18305–18314 CrossRef CAS PubMed.
- Y. Zhong, J. Zhang, T. Li, W. Xu, Q. Yao, M. Lu, X. Bai, Z. Wu, J. Xie and Y. Zhang, Nat. Commun., 2023, 14, 658 CrossRef CAS PubMed.
- X. Kang and M. Z. Zhu, Chem. Soc. Rev., 2019, 48, 2422–2457 RSC.
- M.-M. Zhang, X.-Y. Dong, Z.-Y. Wang, X.-M. Luo, J.-H. Huang, S.-Q. Zang and T. C. W. Mak, J. Am. Chem. Soc., 2021, 143, 6048–6053 CrossRef CAS PubMed.
- Y. Zhou, L. Liao, S. Zhuang, Y. Zhao, Z. Gan, W. Gu, J. Li, H. Deng, N. Xia and Z. Wu, Angew. Chem., Int. Ed., 2021, 60, 8668–8672 CrossRef CAS PubMed.
- X. Jiang, B. Du, Y. Huang and J. Zheng, Nano Today, 2018, 21, 106–125 CrossRef CAS PubMed.
- X. Cai, G. Saranya, K. Shen, M. Chen, R. Si, W. Ding and Y. Zhu, Angew. Chem., Int. Ed., 2019, 58, 9964–9968 CrossRef CAS PubMed.
- H. Liu, Y. Li, S. Sun, Q. Xin, S. Liu, X. Mu, X. Yuan, K. Chen, H. Wang, K. Varga, W. Mi, J. Yang and X.-D. Zhang, Nat. Commun., 2021, 12, 114 CrossRef CAS PubMed.
- X.-K. Wan, J.-Q. Wang and Q.-M. Wang, Angew. Chem., Int. Ed., 2021, 60, 20748–20753 CrossRef CAS PubMed.
- H. Zhu, S. Wang, Y. Wang, C. Song, Q. Yao, X. Yuan and J. Xie, Biomaterials, 2022, 288, 121695 CrossRef CAS.
- Z. Wang, Y. Fang, X. Zhou, Z. Li, H. Zhu, F. Du, X. Yuan, Q. Yao and J. Xie, Nano Res., 2020, 13, 203–208 CrossRef CAS.
- Z. Zuo, X. Pan, G. Yang, Y. Zhang, X. Liu, J. Zha and X. Yuan, Dalton Trans., 2023, 52, 2942–2947 RSC.
- X. Wang, Z. Wang, S. Fang, Y. Hou, X. Du, Y. Xie, Q. Xue, X. Zhou and X. Yuan, Chem. Eng. J., 2021, 420, 127589 CrossRef CAS.
- L. Wang, Q. Hou, W. Zheng and X. Jiang, ACS Nano, 2021, 15, 17885–17894 CrossRef CAS PubMed.
- T. Jia, Z.-J. Guan, C. Zhang, X.-Z. Zhu, Y.-X. Chen, Q. Zhang, Y. Yang and D. Sun, J. Am. Chem. Soc., 2023, 145, 10355–10363 CrossRef CAS PubMed.
- Y. Ge, X. Yali, W. Yaru, T. Ying, C. L. Leng, J. Fuyi, D. Fanglin, Z. Xianfeng, Y. J. Ying and Y. Xun, Nano Res., 2023, 16, 1748–1754 CrossRef.
- X. Yuan, B. Zhang, Z. Luo, Q. Yao, D. T. Leong, N. Yan and J. Xie, Angew. Chem., Int. Ed., 2014, 53, 4623–4627 CrossRef CAS.
- Y.-e. Shi, J. Ma, A. Feng, Z. Wang and A. L. Rogach, Aggregate, 2021, 2, e112 CrossRef CAS.
- X. Yuan, M. I. Setyawati, D. T. Leong and J. Xie, Nano Res., 2014, 7, 301–307 CrossRef CAS.
- R. Yan, S. Sun, J. Yang, W. Long, J. Wang, X. Mu, Q. Li, W. Hao, S. Zhang, H. Liu, Y. Gao, L. Ouyang, J. Chen, S. Liu, X.-D. Zhang and D. Ming, ACS Nano, 2019, 13, 11552–11560 CrossRef CAS PubMed.
- J.-M. Cavaillon, Toxicon, 2018, 149, 45–53 CrossRef CAS PubMed.
|
This journal is © The Royal Society of Chemistry 2024 |
Click here to see how this site uses Cookies. View our privacy policy here.