DOI:
10.1039/D4RA02523G
(Paper)
RSC Adv., 2024,
14, 18501-18507
Harnessing the 1,3-azadiene-anhydride reaction for the regioselective and stereocontrolled synthesis of lactam-fused bromotetrahydropyrans by bromoetherification of lactam-tethered trisubstituted tertiary alkenols†
Received
3rd April 2024
, Accepted 5th June 2024
First published on 10th June 2024
Abstract
Halo-cycloetherification of lactam-tethered alkenols enables the construction of oxygen-heterocycles that are fused to nitrogen heterocycles via intramolecular halonium-induced nucleophilic addition. Specifically, tetrahydropyrans (THPs) that are fused to a nitrogen heterocycle constitute the core of several bioactive molecules, including tachykinin receptor antagonists and alpha-1 adrenergic antagonists. Although the literature is replete with successful examples of the halo-cycloetherification of simple mono- or disubstituted primary alkenols, methods for the modular, efficient, regioselective, and stereocontrolled intramolecular haloetherification of sterically encumbered trisubstituted tertiary alkenols are rare. Here, we describe a simple intramolecular bromoetherification strategy that meets these benchmarks and proceeds with exclusive 6-endo regioselectivity. The transformation employs mild and water-tolerant conditions, which bodes well for late-stage diversification. The hindered ethers contain four contiguous stereocenters as well as one halogen-bearing tetrasubstituted stereocenter.
Introduction
Functionalized δ- and γ-lactams as well as their deoxygenated variants (i.e., piperidines and pyrrolidines) are prevalent in alkaloid natural products, corrosion inhibitors, fruit preservation agents, ligands, and pharmaceuticals (including antibacterial, antioxidants, appetite suppressants, antitumor and antibiotics).1–7 Similarly, tetrahydropyrans (THPs) are commonplace topologies in natural products and pharmaceuticals, including those that possess antibiotic, antiproliferative, antifungal, antimicrobial, anti-HIV activity.8 For example, C2-aryl and polysubstituted THPs can be found in antibacterial agent centrolobine as well as in antifungal agents morinols A and B (Fig. 1). Importantly, fused bicyclic THPs are present in several bioactive molecules, including tachykinin receptor antagonist A, alpha-1 adrenergic antagonist B, and Lubiprostone C.8 We therefore surmised that a modular strategy that merges a functionalized lactam and a tetrahydropyran motif would undeniably expand the 3D-structural space for the discovery of new small molecules with medicinal value.
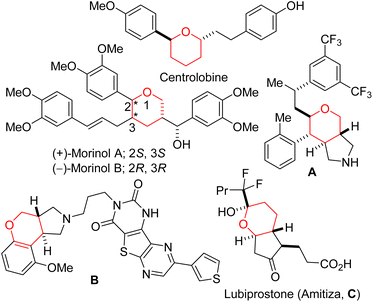 |
| Fig. 1 Examples of bioactive tetrahydropyrans. | |
Diversity-Oriented Synthesis (DOS) is gradually transitioning from structural diversity to biological as well as functional relevance.9 A complementary strategy for generating biologically-relevant chemical libraries is to employ complexity-generating transformations that produce compounds with conformationally rigid cyclic frameworks with a high ratio of sp3 carbon atoms.10 Increasing the three-dimensional character of a molecule has indeed been associated with a more successful outcome in drug discovery.11
In the last decade, our group has sought to harness the 1,3-azadiene-anhydride reaction for the stereocontrolled synthesis of lactam-bearing 5-aryl-4(E)-pentenoic acids and subsequent post-diversification.12 For example, we previously disclosed that the direct oxidative alkoxylation of appropriately tethered alkenols of type 1 proceeds with 6-endo selectivity under Pd- or Cu-catalysis (Fig. 2A, see 2).13 Additionally, we showed that Fe- or Ru-catalyzed dehydrative coupling of 1 furnishes the C–C cross-coupling products (see 3).14
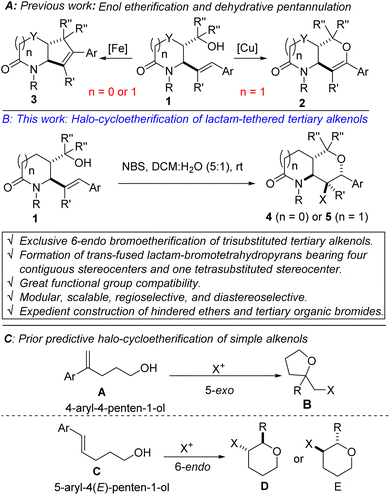 |
| Fig. 2 (A) Prior cyclizations utilizing lactam-tethered alkenol 1, (B) proposed plan for accessing lactam-fused bromotetrahydropyrans from 1, (C) reported predictive approaches for halo-cycloetherification of simple alkenols. | |
In the current studies, we seek to explore the amenability of these lactam-tethered trisubstituted tertiary alkenols to stereocontrolled and regioselective bromoetherification, in view of constructing lactam-fused bromotetrahydropyrans of type 4/5 (Fig. 2B). Halonium-promoted addition of nucleophiles such as alcohols to alkenes is one of the most fundamental reactions in organic chemistry. The intramolecular variant enables the creation of a new ring and the construction of new tetrahedral stereogenic centers. The reaction also introduces a requisite halogen group for subsequent functionalizations. Although the literature is inundated with successful examples of halo-cycloetherification of simple mono- or disubstituted primary alkenols,15 such as those depicted in Fig. 2C, methods for efficient, regioselective, and stereocontrolled haloetherification of sterically encumbered trisubstituted tertiary alkenols are relatively rare. This is hardly surprising given that tertiary alcohols are significantly less nucleophilic than primary alcohols. Additionally, the regioselectivity of attack of the tethered alcohol group on the trisubstituted alkene can hardly be predicted a priori, especially when the more substituted carbon of the alkene does not harbor an aryl substituent (as is the case in alkenol 1).15 Herein, we describe an efficient intramolecular bromoetherification process that meets the aforementioned benchmarks. The reaction proceeds under water-tolerant conditions.
Results and discussion
Studies on the bromoetherification of lactam-tethered alkenols of type 1 commenced with model γ-lactam-tethered alkenol 1a (Table 1) and N-bromosuccinimide (NBS).
Table 1 Optimization of the bromoetherification of lactam-tethered alkenol 1a
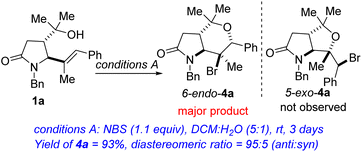
|
Entry |
Deviation from conditions A |
o/o yield of 4a (isolated) |
1 |
Water omitted |
85 |
2 |
1,2-Dichloroethane in place of DCM |
80 |
3 |
Aceton itrile in place of DCM |
79 |
4 |
1,4-Dioxane in place of DCM |
83 |
5 |
HFIP in place of DCM |
69 |
6 |
Diethylether in place of DCM |
83 |
7 |
THF in place of DCM |
88 |
8 |
2-MeTHF in place of DCM |
9 |
9 |
Toluene in place of DCM |
0 |
10 |
Br2 in place of NBS |
86 |
11 |
DBDMH inplace of NBS |
73 |
12 |
NBA in place of NBS |
77 |
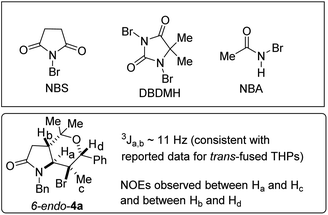 |
After screening several reaction media (i.e., dichloromethane (DCM), 1,2-dichloroethane, acetonitrile, 1,4-dioxane, hexafluoroisopropanol (HFIP), diethylether, tetrahydrofuran (THF), 2-methyltetrahydrofuran (2-MeTHF), and toluene), a cosolvent of DCM and H2O (5
:
1) emerged as the optimum reaction medium with respect to the yield, regioselectivity and stereoselectivity. Thus, after stirring a mixture of 1a (1 mmol) and NBS (1.1 equiv.) in dichloromethane (5 mL) and water (1 mL) for 3 days at room temperature, 1H and 13C NMR analyses of the crude mixture prior to extractive workup revealed the presence of 4a. Of note, the bromocycloetherification performed reasonably in anhydrous DCM (entry 1). Presumably, the role of water is to enhance the solubility of NBS. These studies have revealed that THF out-performs sterically encumbered 2-MeTHF (entry 7 vs. entry 8). Other electrophilic bromine sources did not perform as well as NBS (entries 9–12).
With the optimized conditions in hand, the scope of the bromocycloetherification was next explored (Scheme 1). It is well established that the nitrogen substituent present on a nitrogen heterocycle can have a profound effect on its reactivity and biological activity.16 Thus, the effect of the N-substituent on the cyclization reaction was first explored. Encouragingly, lactam-tethered alkenols harboring electronically diverse N-benzyl substituents undergo satisfactory cyclization (see 4a–e). N-alkyl lactam-alkenols are also competent substrates for the bromoetherification (see 4f/g). The successful construction of lactam-bromotetrahydropyrans harboring the N-phenethyl group (see 4h–j) is noteworthy given that the latter often serves as a precursor to the indolizidine/quinolizidine scaffold.17 Ketone-bearing alkenols are competent substrates for the bromocycloetherification (see 4p). Halogenated arenes are well tolerated (see 4b, 4d, 4e, 4l, 4n, and 4x), which bodes well for late-stage diversification as the halogen group may be utilized as a functional handle for cross-coupling purposes. The incorporation of a fluorinated moiety into organic molecules generally increases the solubility, lipophilicity and metabolic stability of the parent molecules.18 Specifically, the CF3 group enjoys a privileged role because its incorporation often enhances efficacy by promoting electrostatic interactions with targets, improving cellular membrane permeability, and increasing robustness toward oxidative metabolism of the drug.18a It is therefore noteworthy that fluorinated products 4q and 4u are obtainable in satisfactory yields. As a testament to the generality of the transformation, when the phenyl group on the styrenyl unit is replaced by electron-deficient aryl groups (see 4q, 4r, 4t, and 4u) or electron-rich aryl substituents (see 4s and 4w), the efficacy of the transformation is not compromised. In addition to the trisubstituted γ-lactam-tethered alkenols that have been interrogated, we have found that intramolecular bromoalkoxylation of γ-lactam-tethered disubstituted alkenols unsurprisingly proceeds regioselectively in favor of the 6-endo cyclization pathway (see 4v/w).
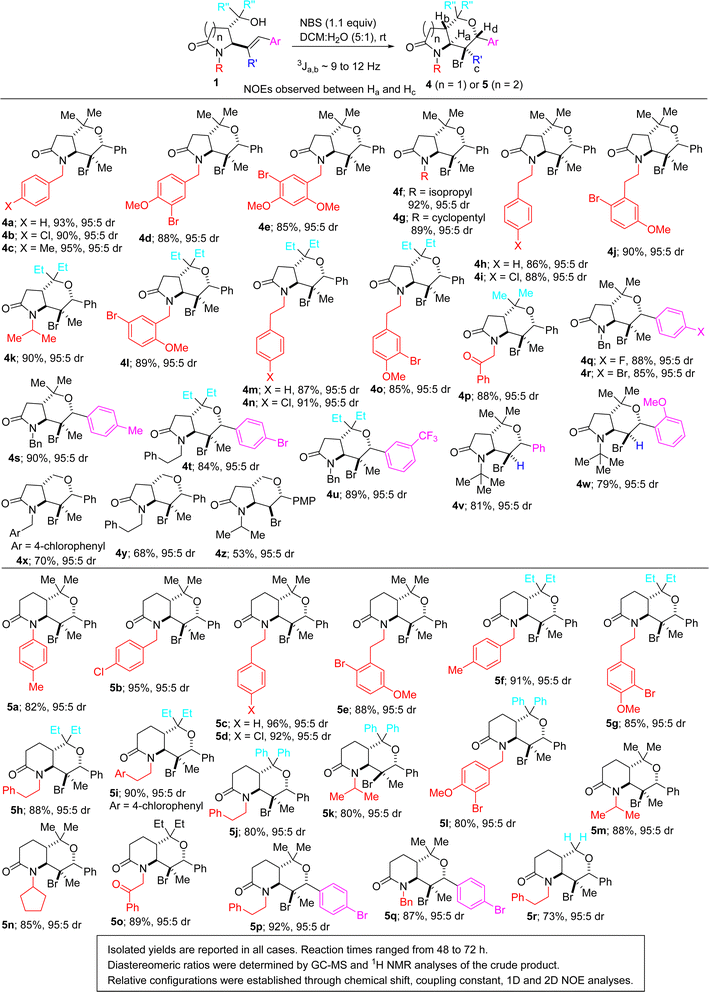 |
| Scheme 1 Scope of bromoetherification of lactam-tethered alkenols. | |
Medicinal chemists have widely explored the gem-dimethyl group in developing bioactive molecules because of the possibility to (1) mitigate toxicity, (2) obtain superior DMPK profile, (3) modulate the pKa of nearby functionality, (4) induce symmetry into a monomethyl substituted chiral center, (5) increase target engagement, potency, and selectivity through van der Waals interactions and entropically favorable restriction to a bioactive conformation, and (6) apply the Thorpe–Ingold conformational effect, to achieve difficult ring-forming transformations.19 As of 2017, 3.7% FDA-approved drugs in the US contained the gem-dimethyl group.20 The successful regioselective and stereocontrolled synthesis of the gem-dimethylated lactam-fused bromotetrahydropyrans depicted in Scheme 1 (see 4a–j, 4q–s, 4v, and 4w) is therefore noteworthy.‡ In a succinct manifestation of the Thorpe–Ingold effect, inherently more reactive primary alkenols are less competent than their tertiary alkenol counterparts in this bromocycloetherification protocol (see 4b vs. 4x and 4h/m vs. 4y). The remaining mass balance in these primary alkenol cases was accounted for by the 5-exo cyclization products, which are separable by chromatography.
Primarily due to conformational differences, extending reactivity trends from one class of heterocycle to another class can be quite daunting and even foolhardy at times. It was therefore gratifying to find that δ-valerolactam-tethered alkenols also react regioselectively and diastereoselectively with NBS to afford the 2-piperidinone-fused bromotetrahydropyrans depicted in Scheme 1 (see 5a–r). There are high incentives for the construction of these bicyclic piperidine derivatives since the piperidine motif is arguably the most prevalent in pharmaceuticals and the strategic placement of substituents about this three-dimensional scaffold is ideally suited for structure–activity relationship studies.11c The successful deployment of a lactam-tethered tertiary alkenol harboring an N-aryl substituent (see 5a) is noteworthy given that N-aryl piperidines and 2-oxopiperidines are embedded in several pharmacologically pertinent targets, including Factor Xa inhibitors and phosphodiesterase-10 inhibitors.21 Sterically encumbered δ-valerolactam-tethered alkenols bearing two phenyl substituents on the alcohol-containing carbon have been interrogated in this bromoetherification protocol. They reacted sluggishly but still furnished the lactam-fused bromotetrahydropyrans in synthetically attractive yields and diastereoselectivities (see 5j–l).
In the synthesis of potential drug candidates, scalability is often a significant factor as it serves to provide sufficient amounts for clinical tests. A potentially beneficial aspect of this methodology is therefore the scalable nature of the reactions given that products such as 3c13 have been prepared in gram scale, with little to no compromise in efficiency and diastereoselectivity.
The 1H NMR spectra of 4/5 revealed coupling constants for the ring-fusion positions (3JA,B) ranging from 9.0 to 12.0 Hz for protons Ha and Hb (where a refers to the α-amino proton and b refers to the β-amino proton, see Scheme 1 and Table 1). The observed values of 3Ja,b for the bicyclic THPs depicted in Scheme 1 fall in the range typical for trans-fused THPs. NOESY experiments confirmed that the anti-diastereomer is the predominant stereoisomer.
A widely accepted mechanism for this electrophilic halo-cycloetherification is that an electrophilic halogen is first transferred from a halogen source (NBS in this case) to the alkene to form a halonium ion, followed by an intramolecular attack by the pendant alcohol group. The observed 6-endo regioselective addition in a Markovnikov sense is the expected outcome for such 5-aryl-4(E)-penten-1-ols, characterized by an unsymmetrical build-up of positive charge next to the aryl group in the transition state.22 The stereocontrolled anti-addition (with respect to the newly formed C–O and C–X bonds) is consistent with intramolecular attack of the transient halonium ion by the pendant alcohol group from the opposite side. The possibility of formation of a bromohydrin intermediate prior to attack by the tethered alcohol motif cannot be ruled out at this stage given that such a product was isolated when the tertiary alcohol motif resident in 1 was replaced by a methyl ketone (Scheme 2).
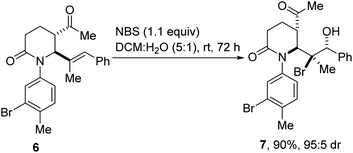 |
| Scheme 2 Evidence of formation of a bromohydrin from homoallylic ketone 6 under similar reaction conditions. | |
Conclusions
In summary, the site-selective, efficient, and stereocontrolled synthesis of lactam-fused bromotetrahydropyrans has been accomplished through the thermodynamic bromoetherification of sterically imposing lactam-tethered tertiary trisubstituted alkenols. The innate tendency of these lactam-tethered-5-aryl-4(E)-penten-1-ols to undergo thermodynamic 6-endo cyclization is enforced by the presence of the 5-aryl substituent. These sp3-rich fused lactam-bromotetrahydropyrans bear four contiguous stereocenters. We anticipate that this efficient strategy would expand the 3D-structural space for the discovery of new lactam-tetrahydropyrans with medicinal value. Efforts to develop an enantioselective variant of this transformation are ongoing and the results will be disclosed in due course.
Experimental
All experiments involving air and moisture-sensitive reagents were carried out under an inert atmosphere of nitrogen and using freshly distilled solvents. Freshly purchased toluene and DMF were stored under 4 Å molecular sieves for several days prior to use. THF and 2-MeTHF were distilled from sodium benzophenone ketyl. All other solvents were used as purchased. All amines, enals, Grignard reagents, and N-bromosuccinimide were newly purchased and used without further purification. Column chromatography was performed on silica gel (230–400 mesh). Thin-layer chromatography (TLC) was performed using Silicycle SiliaplateTM glass backed plates (250 μm thickness, 60 Å porosity, F-254 indicator) and visualized using UV (254 nm). Unless otherwise indicated, NMR spectral data were acquired using CDCl3 as solvent, at room temperature. Chemical shifts are quoted in parts per million (ppm). HRMS-EI+ data were obtained using either electron spray ionization (ESI) or electron impact (EI) techniques. High-resolution ESI was obtained on an LTQ-FT (ion trap; analyzed using Excalibur). High resolution EI was obtained on an Autospec (magnetic sector; analyzed using MassLynx).
General procedure A: bromoetherification of lactam-tethered alkenol 1
An oven-dried vial was equipped with a stir bar and a solution of 1 (1.00 mmol) in dichloromethane (5 mL) was added to the vial followed by deionized water (1 mL) and N-bromosuccinimide (1.10 mmol, 1.1 equiv.) at room temperature. After stirring for 3 days at the same temperature, the reaction mixture diluted by the addition of dichloromethane (20 mL) and quenched by the addition of saturated sodium thiosulfate solution (10 mL). The layers were separated and the aqueous layer was extracted with dichloromethane (20 mL). The combined organic layers were washed with brine (20 mL), dried over anhydrous sodium sulfate, and concentrated in vacuo. The residue was purified by flash column chromatography on silica gel to afford the lactam-fused bromotetrahydropyran.
Typical procedure A
To a solution of alkenol 1a (349.5 mg, 1.00 mmol) in dichloromethane (10 mL) was added N-bromosuccinimide (195.8 mg, 1.10 mmol) at room temperature. After stirring for 3 days at the same temperature, the reaction mixture diluted by the addition of dichloromethane (20 mL) and quenched by the addition of saturated sodium thiosulfate solution (10 mL). The layers were separated and the aqueous layer was extracted with dichloromethane (20 mL). The combined organic layers were washed with brine (20 mL), dried over anhydrous sodium sulfate, and concentrated in vacuo.
Purification
By flash chromatography on silica eluting with hexane/acetone (90
:
10 to 70
:
30). Yellowish oil. Yield = 398.4 mg, 93%, 95
:
5 dr (anti:syn). 1H NMR (400 MHz, CDCl3) δ 7.48–7.39 (m, 2H), 7.39–7.14 (m, 8H), 5.04 (d, J = 15.7 Hz, 1H), 4.89 (s, 1H), 4.73 (d, J = 15.7 Hz, 1H), 4.19 (d, J = 10.8 Hz, 1H), 2.51–2.30 (m, 2H), 2.19–2.08 (m, 1H), 1.47 (s, 3H), 1.33 (s,s, 6H). 13C NMR (101 MHz, CDCl3) δ 175.66, 137.38, 136.01, 129.75, 128.36, 128.33, 127.81, 127.34, 126.98, 80.52, 74.89, 68.08, 67.67, 47.62, 45.20, 33.24, 29.37, 18.19, 17.83. HRMS-EI+ (m/z): calc. for C23H26BrNO2 [M]+ 427.1147, found 427.1153. FTIR (KBr): 2965.4, 1727.5, 1696.3, 1604.9, 1511.0, 1448.5, 1414.7, 1384.9, 1357.4, 1298.7, 1247.5, 1179.3, 1135.9, 1031.8, 905.8, 839.0.
Author contributions
I. S. A. – investigation, data curation, methodology; T. K. B. – conceptualization, project administration, supervision, investigation, data curation, methodology, writing – original draft, internal and external funding acquisition.
Conflicts of interest
There are no conflicts of interest to declare.
Acknowledgements
We are grateful to Central Washington University for financial support through startup funds and continuous maintenance of major research instruments. The school of graduate studies and the office of the provost are thanked for research fellowships to T. K. B. and I. S. A. We thank Organic Syntheses, Inc for partial support of this project.
Notes and references
-
(a) R. M. Trend, Y. K. Ramtohul, E. M. Ferreira and B. Stoltz, Angew. Chem., Int. Ed., 2003, 42, 2892 CrossRef CAS PubMed;
(b) X.-F. Cheng, Y. Li, Y.-M. Su, F. Yin, J.-Y. Wang, J. Sheng, H. U. Vora, X.-S. Wang and J.-Q. Yu, J. Am. Chem. Soc., 2013, 135, 1236 CrossRef CAS PubMed;
(c) W. Yang, S. Wang, Q. Zhang, Q. Liu and X.-X. Xu, Chem. Commun., 2015, 51, 661 RSC;
(d) X.-M. Xie and S. S. Stahl, J. Am. Chem. Soc., 2015, 137, 3767 CrossRef CAS PubMed;
(e) H. Shigehisa, M. Hayashi, H. Ohkawa, T. Suzuki, H. Okayasu, M. Mukai, A. Yamazaki, R. Kawai, H. Kikuchi, Y. Satoh, A. Fukuyama and K. Hiroya, J. Am. Chem. Soc., 2016, 138, 10597 CrossRef CAS PubMed;
(f) Y.-J. Zhang, T. Abe, T. Tanaka, C.-R. Yang and I. Kouno, J. Nat. Prod., 2001, 64, 1527 CrossRef CAS PubMed;
(g) J. J. Beck and S.-C. Chou, J. Nat. Prod., 2007, 70, 891 CrossRef CAS PubMed;
(h) Y. Wache, M. Aguedo, J. M. Nicaud and J. M. Belin, Appl. Microbiol. Biotechnol., 2003, 61, 393 CrossRef CAS PubMed;
(i) A. Parenty, X. Moreau and J. M. Campagne, Chem. Rev., 2006, 106, 911 CrossRef CAS PubMed;
(j) M. I. Konaklieva and B. J. Plotkin, Mini-Rev. Med. Chem., 2005, 5, 73 CrossRef CAS PubMed;
(k) I. Collins, J. Chem. Soc., Perkin Trans. 1, 1998, 1, 1869–1888 RSC.
-
(a) S. Rashid, B. A. Bhat and G. Mehta, Org. Lett., 2015, 17, 3604–3607 CrossRef CAS PubMed;
(b) J. Cao and P. Perlmutter, Org. Lett., 2013, 15, 4327–4329 CrossRef CAS PubMed.
-
(a) L. I. LlarrVull, S. A. Testero, J. F. Fisher and S. Mobashery, Curr. Opin. Microbiol., 2010, 13, 551–557 CrossRef PubMed;
(b) J. Caruano, G. G. Muccioli and R. Robiette, Org. Biomol. Chem., 2016, 14, 10134–10156 RSC;
(c) A. Lepikhina, O. Bakulina, D. Dar’in and M. Krasavin, RSC Adv., 2016, 6, 83808–83813 RSC;
(d) P. Gross and J. A. Zapp, CRC Crit. Rev. Toxicol., 1984, 13, 205–216 CrossRef CAS PubMed;
(e) F. J. R. Rombouts, G. Tresadern, O. Delgado, C. Martinez Lamenca, M. Van Gool, A. Garcia-Molina, S. A. Alonso de Diego, D. Oehlrich, H. Prokopcova, J. M. Alonso, N. Austin, H. Borghys, S. Van Brandt, M. Surkyn, M. De Cleyn, A. Vos, R. Alexander, G. Macdonald, D. Moechars, H. Gijsen and A. A. Trabanco, J. Med. Chem., 2015, 58, 8216 CrossRef CAS PubMed.
-
(a) M. Shahid, F. Sobia, A. Singh, A. Malik, H. M. Khan, D. Jonas and P. M. Hawkey, Crit. Rev. Microbiol., 2009, 35, 81–108 CrossRef CAS PubMed;
(b) K.-F. Kong, L. Schneper and K. Mathee, APMIS, 2010, 118, 1–36 CrossRef CAS PubMed;
(c) G. Kapoor, S. Saigal, A. Elongavan and J. Anaesthesiol, Clin. Pharmacol., 2017, 33, 300–305 CAS.
-
(a) R. D. Marco, A. Bedini, S. Spampinato, L. Comellini, J. Zhao, R. Artali and L. Gentilucci, J. Med. Chem., 2018, 61, 5751–5757 CrossRef PubMed;
(b) S. M. Rawls, W. Robinson, S. Patel and A. Baron, Neuropharmacology, 2008, 55, 865 CrossRef CAS PubMed.
- E. J. Velthuisen, B. A. Johns, D. P. Temelkoff, K. W. Brown and S. C. Danehower, Eur. J. Med. Chem., 2016, 117, 99–112 CrossRef CAS PubMed.
-
(a) M. Baiula, P. Galletti, G. Martelli, R. Soldati, L. Belvisi, M. Civera, S. D. Dattoli, S. M. Spampinato and D. Giacomini, J. Med. Chem., 2016, 59, 9721–9742 CrossRef CAS PubMed;
(b) D. Kuhn, C. Coates, K. Daniel, D. Chen, M. Bhuiyan, A. Kazi, E. Turos and Q. P. Dou, Front. Biosci., 2004, 9, 2605–2617 CrossRef PubMed;
(c) B. Xing, J. Rao and R. Liu, Mini-Rev. Med. Chem., 2008, 8, 455–471 CrossRef CAS PubMed.
-
(a) B.-N. Su, Y. Takaishi and T. Kusumi, Tetrahedron, 1999, 55, 14571–14586 CrossRef CAS;
(b) S. Yamauchi, S. Kawahara, T. Wukirsari, H. Nishiwaki, K. Nishi, T. Sugahara, K. Akiyama and T. Kishida, Bioorg. Med. Chem. Lett., 2013, 23, 4923–4930 CrossRef CAS PubMed;
(c) K. Akiyama, S. Yamauchi, M. Maruyama, T. Sugahara, T. Kishida and Y. Koba, Biosci., Biotechnol., Biochem., 2009, 73, 129–133 CrossRef CAS PubMed;
(d) K. Masuda, H. Nishiwaki, K. Akiyama, S. Yamauchi, M. Maruyama, T. Sugahara and T. Kishida, Biosci., Biotechnol., Biochem., 2010, 74, 2071–2076 CrossRef CAS PubMed;
(e) R. J. Devita, J. Bao, and S. G. Mills, WO 2007075528 A2, 2007;
(f) P. A. Clarke and S. Santos, Eur. J. Org Chem., 2006, 9, 2045–2053 CrossRef;
(g) N. M. Nasir, K. Ermanis and P. A. Clarke, Org. Biomol. Chem., 2014, 12, 3323–3335 RSC;
(h) F. Vetica, P. Chauhan, S. Dochain and D. Enders, Chem. Soc. Rev., 2017, 46, 1661–1674 RSC;
(i) I. M. Pastor and M. Yus, Curr. Org. Chem., 2007, 11, 925–957 CrossRef CAS;
(j) H. Fuwa, T. Noguchi, K. Noto and M. Sasaki, Org. Biomol. Chem., 2012, 10, 8108–8112 RSC;
(k) X. Han, G. Pey and P. E. Floreancig, Eur. J. Org Chem., 2013, 7, 1193–1208 CrossRef;
(l) M. Ikoma, M. Oikawa, M. B. Gill, G. T. Swanson, R. Sakai, K. Shimamoto and M. Sasaki, Eur. J. Org Chem., 2008, 5215–5220 CrossRef CAS PubMed;
(m) M. Ikoma, M. Oikawa and M. Sasaki, Tetrahedron, 2008, 64, 2740–2749 CrossRef CAS;
(n) M. Oikawa, M. Ikoma, M. Sasaki, M. B. Gill, G. T. Swanson, K. Shimamoto and R. Sakai, Eur. J. Org Chem., 2009, 5531–5548 CrossRef CAS PubMed;
(o) P. Escavabaja, J. Viala, Y. Coquerel and J. Rodriguez, Adv. Synth. Catal., 2012, 354, 3200–3204 CrossRef CAS;
(p) R. Sibley, H. Hatoum-Mokdad, R. Schoenleber, L. Musza, W. Stirtan, D. Marrero, W. Carley, H. Xiao and J. Dumas, Bioorg. Med. Chem. Lett., 2003, 13, 1919–1922 CrossRef CAS PubMed;
(q) L. G. Hamann, J. H. Meyer, D. A. Ruppar, K. B. Marschke, F. J. Lopez, E. A. Allegretto and D. S. Karanewsky, Bioorg. Med. Chem. Lett., 2005, 15, 1463–1466 CrossRef CAS PubMed;
(r) R. W. Hsieh, S. S. Rajan, S. K. Sharma, Y. Guo, E. R. DeSombre, M. Mrksich and G. L. Greene, J. Biol. Chem., 2006, 281, 17909–17919 CrossRef CAS PubMed;
(s) M. Nakamura, K. Niiyama and T. Yamakawa, Tetrahedron Lett., 2009, 50, 6462–6465 CrossRef CAS.
-
(a) I. Pavlinov, E. M. Gerlach and L. N. Aldrich, Org. Biomol. Chem., 2019, 17, 1608–1623 RSC;
(b) K.-J. Liu, Z. Wang, L.-H. Lu, J.-Y. Cheng, Y.-W. Lin, Z. Cao, X. Yu and W.-M. He, Green Chem., 2021, 23, 496–500 RSC;
(c) X. Wang, Q. Wang, Y. Xue, K. Sun, L. Wu and B. Zhang, Chem. Commun., 2020, 56, 4436–64439 RSC;
(d) Y. Zhao, X. Niu, H. Yang, J. Yang, Z. Wang and Q. Wang, Chem. Commun., 2022, 58, 8576–8579 RSC;
(e) S. Jin, L. Wang, H. Han, X. Liu, Z. Bu and Q. Wang, Chem. Commun., 2021, 57, 359–362 RSC;
(f) Q. H. Shi, Y.-H. Wang, X.-R. Wang, W.-H. Zhang, F.-L. Tian, L.-J. Peng, Y. Zhou and X.-L. Liu, Chem. Front., 2023, 10, 359–362 Search PubMed.
- C. J. Gerry and S. L. Schreiber, Nat. Rev. Drug Discovery, 2018, 17, 3307–3312 CrossRef PubMed.
-
(a) T. Flagstad, G. Min, K. Bonnet, R. Morgentin, D. Roche, M. H. Clausen and T. E. Nielsen, Org. Biomol. Chem., 2016, 14, 4943–4946 RSC;
(b) D. J. Foley, A. Nelson and S. P. Marsden, Angew. Chem., Int. Ed., 2016, 55, 13650–13657 CrossRef CAS PubMed;
(c) F. Lovering, J. Bikker and C. Humblet, J. Med. Chem., 2009, 52, 6752–6756 CrossRef CAS PubMed.
-
(a) H. Braunstein, S. Langevin, M. Khim, J. Adamson, K. Hovenkotter, L. Kotlarz, B. Mansker and T. K. Beng, Org. Biomol. Chem., 2016, 14, 8864–8872 RSC;
(b) T. K. Beng and A. Moreno, New J. Chem., 2020, 44, 4257–4261 RSC;
(c) T. K. Beng and A. Moreno, RSC Adv., 2020, 10, 8805–8809 RSC;
(d) J. Garcia, J. Eichwald, J. Zesiger and T. K. Beng, RSC Adv., 2022, 12, 309–318 RSC;
(e) T. K. Beng, M. Rodriguez and C. Borg, RSC Adv., 2022, 12, 17617–17620 RSC;
(f) T. K. Beng, C. Borg and M. Rodriguez, RSC Adv., 2022, 12, 28685–28691 RSC;
(g) T. K. Beng, J. Garcia, J. Eichwald and C. Borg, RSC Adv., 2023, 13, 14355–14360 RSC;
(h) A. O. Farah, J. GVarcia, C. Borg and T. K. Beng, RSC Adv., 2023, 13, 3181–3185 RSC;
(i) T. K. Beng, J. Eichwald, J. Fessenden, K. Quigley, S. Sharaf, N. Jeon and M. Do, RSC Adv., 2023, 13, 21250–21258 RSC;
(j) M. Do, S. I. Anosike and T. K. Beng, RSC Adv., 2023, 13, 25691–25698 RSC.
- K. Hovenkotter, H. Braunstein, S. Langevin and T. K. Beng, Org. Biomol. Chem., 2017, 15, 1217–1221 RSC.
- T. K. Beng, M. Bauder, M. J. Rodriguez and A. Moreno, New J. Chem., 2018, 42, 16451–16455 RSC.
-
(a) X. Zeng, C. Miao, S. Wang, C. Xia and W. Sun, Chem. Commun., 2013, 49, 2418–2420 RSC;
(b) Z. Yuan, H. Peng and G. Liu, Chin. J. Chem., 2013, 31, 908–91 CrossRef CAS;
(c) L. F. Lourie, Y. A. Serguchev, M. V. Ponomarenko, E. B. Rusanov, M. V. Vovk and N. V. Ignatev, Tetrahedron, 2013, 69, 833–838 CrossRef CAS;
(d) S. E. Denmark and M. T. Burk, Org. Lett., 2012, 14, 256–259 CrossRef CAS PubMed;
(e) D. Huang, H. Wang, F. Xue, H. Guan, L. Li, X. Peng and Y. Shi, Org. Lett., 2011, 13, 6350–6353 CrossRef CAS PubMed;
(f) A. M. MontaÇa, C. Batalla and J. A. Barcia, Curr. Org. Chem., 2009, 13, 919–938 CrossRef;
(g) M. Sawaguchi, S. Hara, T. Fukuhara and N. Yoneda, Tetrahedron, 2000, 104, 277–280 CAS;
(h) G. Cardillo and M. Orena, Tetrahedron, 1990, 46, 3321–3408 CrossRef CAS;
(i) D. S. Hamilton and D. A. Nicewicz, J. Am. Chem. Soc., 2012, 134, 18577–18580 CrossRef CAS PubMed;
(j) R. Lin, H. Sun, C. Yang, Y. Yang, X. Zhao and W. Xia, Beilstein J. Org. Chem., 2015, 11, 31–36 CrossRef PubMed.
- T. K. Beng and R. E. Gawley, J. Am. Chem. Soc., 2010, 132, 12216 CrossRef CAS PubMed.
- A. Calcaterra, L. Mangiardi, G. D. Monache, D. Quaglio, S. Balducci, S. Berardozzi, A. Iazzetti, R. Franzini, B. Botta and F. Ghirga, Molecules, 2020, 25, 414 CrossRef PubMed.
-
(a) T. Liang, C. N. Neumann and T. Ritter, Angew. Chem., Int. Ed., 2013, 52, 8214–8264 CrossRef CAS PubMed;
(b) D. O'Hagan, J. Fluorine Chem., 2010, 131, 1071–1081 CrossRef;
(c) J. Wang, M. Sánchez-Roselló, J. L. Aceña, C. del. del Pozo, A. E. Sorochinsky, S. Fustero, V. A. Soloshonok and H. L. Liu, Chem. Rev., 2014, 114, 2432–2506 CrossRef CAS PubMed;
(d) T. Fujiwara and D. O'Hagan, J. Fluorine Chem., 2014, 167, 16–29 CrossRef CAS;
(e) R. Berger, G. Resnati, P. Metrangolo, E. Weber and J. Hulliger, Chem. Soc. Rev., 2011, 40, 3496–3508 RSC;
(f) E. A. Ilardi, E. Vitaku and J. T. Njardarson, J. Med. Chem., 2014, 57, 2832–2842 CrossRef CAS PubMed.
- R. M. Beesley, C. K. Ingold and J. F. Thorpe, J. Chem. Soc., Trans., 1915, 107, 1080–1106 RSC.
- D. S. Wishart, C. Knox, A. C. Guo, S. Shrivastava, M. Hassanali, P. Stothard, Z. Chang and J. Woolsey, Nucleic Acids Res., 2006, 34, 668–672 CrossRef PubMed; Nakamura, T. Matsushita, M. Shimura and H. Fujioka, Chem.–Eur. J., 2012, 18, 8448–8453 CrossRef PubMed.
-
(a) X. Qiao, D. L. Cheney, R. S. Alexander, A. M. Smallwood, S. R. King, K. He, A. R. Rendina, J. M. Luettgen, R. M. Knabb, R. R. Wexler and P. Y. S. Lam, Bioorg. Med. Chem. Lett., 2008, 18, 4118–4123 CrossRef PubMed;
(b) M. A. Larsen, E. T. Hennessy, M. C. Deem, Y. Lam, J. Sauri and A. C. Sather, J. Am. Chem. Soc, 2020, 142(2), 726–732 CrossRef CAS PubMed.
-
(a) F. Freeman, Chem. Rev., 1975, 75, 439–490 CrossRef CAS;
(b) K. Murai, A. Nakamura, T. Matsushita, M. Shimura and H. Fujioka, Chem.–Eur. J., 2012, 18, 8448–8453 CrossRef CAS PubMed.
Footnotes |
† Electronic supplementary information (ESI) available: Experimental procedures and spectroscopic data. See DOI: https://doi.org/10.1039/d4ra02523g |
‡ All other lactam-fused bromotetrahydropyrans depicted in Scheme 1 were prepared as described above. Spectroscopic data can be found in the ESI.† |
|
This journal is © The Royal Society of Chemistry 2024 |
Click here to see how this site uses Cookies. View our privacy policy here.