DOI:
10.1039/D4RA02573C
(Paper)
RSC Adv., 2024,
14, 22497-22503
Determination of trace heavy metal elements in litterfall by inductively coupled plasma optical emission spectrometry after extraction using choline chloride-based deep eutectic solvents†
Received
5th April 2024
, Accepted 9th July 2024
First published on 16th July 2024
Abstract
The development of a green, safe, and accurate sample preparation method for the determination of trace metal elements in environmental samples is of great importance. Choline chloride-based deep eutectic solvents (DESs) were used to extract heavy metal elements from litterfall and the target analytes were measured using inductively coupled plasma optical emission spectrometry. Factors such as the type, ratio, dosage, and extraction time and temperature of the DESs were studied. A DES system based on choline chloride and maleic acid had the highest extraction efficiency of 98.5%, 88.4%, 90.2%, and 93.7% for Cd, Cu, Zn, and Fe. Under the optimized conditions, the limits of detection and limits of quantification were in the range of 0.04–0.70 and 0.13–2.30 mg kg−1. The repeatability (n = 3), estimated in terms of the relative standard deviation, ranged from 1.14% to 3.40%. The proposed method was validated for accuracy using GBW10087. Notably, the energy consumption of the newly developed method was only one-fifth that of a traditional acid digestion method. This work not only presents an environmentally friendly method for the determination of trace element concentrations in environmental samples but also deepens our understanding of DES systems.
1. Introduction
In the natural environment, plant litter is the primary source of soil carbon and macronutrients.1 The release of trace elements such as iron (Fe), manganese (Mn), zinc (Zn), copper (Cu) and organic matter is controlled during its decomposition process.2 Previous studies have mainly focused on the loss of mass and dynamics of carbon and macronutrients, such as nitrogen and phosphorus, in plant litter, rather than the content of trace elements.3 It is crucial to determine the presence of heavy metals in litter to assess potential environmental risks.
Like the other plant-based samples, the determination of heavy elements in litterfall requires their pre-treatment in order to transfer the analytes to an aqueous phase compatible with spectroscopic instruments. Several pretreatment methods have been developed for the extraction of metals from complex matrices, such as dry ashing,4 wet digestion,5 microwave digestion,6 and ultrasound-assisted dissolution.7 The most commonly employed sample pretreatment procedures are typically based on the digestion of the sample in a mixture of acid (typically concentrated sulphuric or nitric acid) and an oxidizing agent (such as hydrogen peroxide).8 The emission of acid vapors into the atmosphere is led by the use of concentrated sulfuric or nitric acids in the digestion process.9 While the final solution containing excess acid should be neutralized/diluted for introduction into the spectroscopic instrument.
Ionic liquids are considered to be possible substitutes for conventional organic solvents because of their low melting point, high stability, and superior solubility.10 Since ionic liquids alter the hydration environment of metal ions by providing more hydrophobic regions around the metal through the presence of their own organic ligands,11 heavy metal contaminants can be properly leached using ionic liquids. They have been used to micro-extract trace elements from wastewater,12 pharmaceuticals,13 pesticides,14 and fuel.15 Ionic liquids themselves have certain inherent drawbacks, such as low biodegradability, elevated synthesis costs, a certain level of toxicity and difficult to recover,16 thereby limiting their potential for development.17 Deep eutectic solvents with similar physical properties to ionic liquids have lower toxicity and cost, and are more advantageous for sample pre-treatment methods.
Deep eutectic solvent (DES) consist of a hydrogen bond donor (HBD) and acceptor (HBA), the formation of which results in a lower melting point for the system.18 Many DESs are liquids at room temperature and have properties such as low vapor pressure, wide liquid phase range and biodegradability.19 Due to the attractive properties of DES, they have been used as solvents in different chemical fields. DESs have been reported to separate metals from food,20 soil,21 and water.22 In particular, acid-based DESs can significantly enhance the leaching of metals.23 Kamal Ghanemi used choline chloride–oxalic acid deep eutectic solvents as a medium for microwave digestion as an alternative to nitric acid, which was successfully applied for the detection of Cu, Fe, Ni and Zn in marine samples.24 On the other hand, deep eutectic solvents can also be used as extraction media in the field of microextraction.25 The utilization of DES showcases its potential as a green and efficient alternative in sample preparation for elemental analysis, contributing to advancements in environmentally conscious analytical techniques.
Prior to its determination, an element must be converted into a measurable form through pre-treatment. The majority of sample pre-treatment methods are time-consuming and generate waste. In view of the above, this study proposed a new green method for the extraction of Cd, Cu, Fe and Zn elements from litterfall, which were then analyzed by inductively coupled plasma emission spectrometry. Choline chloride/maleic acid mixture was chosen as the eutectic solvent to extract the target from the litter. Choline chloride/maleic acid DES was chosen because of its high ability to dissolve metal oxides compared to other DES such as ChCl–urea and ChCl–ethylene glycol. The effects of DES type, ratio, dosage, extraction time and extraction temperature on the target extract were investigated. This extraction method can be considered an environmentally friendly alternative to most of the used sample pre-treatment procedures based on wet digestion.
2. Materials and methods
2.1 Materials
All chemicals were of analytical reagent grade. Choline chloride (C5H14NClO, denoted ChCl, 99%), ethylene glycol (99%), glycerol (98%), formic acid (85%), acetic acid (99%), lactic acid (99%), malic acid (99%), malonic acid (99%), and maleic acid (99%) were purchased from Macklin Inc. Working solutions were prepared by diluting 10 g L−1 of standard substances containing Cd, Cu, Fe, and Zn using ultrapure water. The GBW10022 standard substance was used to evaluate the experimental accuracy.
2.2 Instrumentation
The analytes (Cu, Fe, Cd, and Zn) were quantified using ICP-OES (ICAP 7000). Samples were introduced via concentric sprayers and cyclonic spray chambers. The operational parameters of the instrument are listed in Table 1. Graphite eliminator (YKM-36) was used for sample wet digestion. German IKA magnetic heating stirrer (RCT BASIC S025) was used for heating and stirring process. The high-speed centrifuge K82602-F000 was used to separate solutions and precipitates. Fourier transform infrared (FTIR) (Thermo Fisher Scientific Nicolet iS20) was used to characterize the group changes of the HBA, HBD and DES. Water deionizer model Eco-S15 was used to obtain water with a mass of 18.2 MΩ cm−1 meeting quality for the experiments.
Table 1 The parameters for ICP-OES
ICP-OES parameters |
RF generator power (W) |
1150 |
Frequency of RF generator (MHz) |
40 |
Coolant gas flow rate (L min−1) |
10 |
Carrier gas flow rate (L min−1) |
0.7 |
Auxiliary gas (L min−1) |
1.0 |
Max integration times (s) |
15 |
Pump rate (rpm) |
50 |
Replicate |
3 |
Flush time (s) |
30 |
Spectral lines |
324.7Cu, 228.8Cd, 259.9Fe, 213.8Zn |
2.3 Samples
Bamboo litterfall samples were collected from subtropical bamboo forests in Qingshan Township, Hangzhou, China. All the samples were preserved in polythene bags and placed in an oven at 80 °C for drying before used. The samples were sieved through a <120 mesh sieve after being crushed through a shredder and then stored in pre-washed and dried polyethylene containers at ambient temperature. Spiked samples were also prepared as follows: 50 mL of the working analytical solution was mixed with a 5 g sample and shaken well in a shaker for 3 h. The suspension was filtered, and the spiked samples were dried at 100 °C and set aside.
2.4 Preparation of DESs
The DESs were prepared by mixing the carboxylic acid and polyol (HBD) and choline chloride in a molar ratio of 1
:
1. The resulting mixture was then heated at 90 °C on a magnetic stirrer until a homogeneous and transparent liquid was obtained.
2.5 Wet digestion of samples
The 0.10 g portion of litterfall samples was added to a digestion tube with 5.0 mL of concentrated H2SO4 and 2.0 mL of H2O2. The same procedure for wet digestion was used as reported in previous studies.26 First, a graphite digester was heated to 200 °C and maintained for 1 h until the sample was dissolved. The sample was then cooled to room temperature and diluted to 50 mL with ultrapure water. All samples were tested at least three times.
2.6 DES-mediated extraction
The 1.50 g portion of DES was added to 0.10 g of plant sample and the resulting mixture stirred at 70 °C for 45 min. Then, 5.0 mL of ultrapure water was added to dissolve the DES. The resulting mixture was filtered through a microporous membrane with a pore size of 0.45 μm and diluted to a certain concentration to test the trace element content by ICP-OES. All samples were tested at least three times.
2.7 Effect of hydrogen bond donor on extraction efficiency
Eight DESs with different hydrogen bond donors were prepared to investigate the effect of the hydrogen bond donor properties on the extraction process. The standard sample (0.1 g) was mixed with 1.50 g of DES and magnetically stirred at 70 °C for 45 min. Afterward, the DES was dissolved by adding 5 mL of ultrapure water, and the solution was filtered. The solution was diluted tenfold and analyzed using ICP-OES. The extraction recovery (ER) was calculated by the following formula:
where CDES and CWET were the target metal concentration extracted using the DES-mediated extraction and traditional wet digestion methods, respectively.
3. Results and discussion
In this study, the DES extraction method was combined with ICP-OES to determine the content of Cd, Cu, Fe and Zn in litterfall samples. For this purpose, the effect of some variables (DES type, ratio, mass, time and temperature) affecting the extraction efficiency was studied and optimized. A one-variable-at-a-time optimization method was used to obtain the best conditions. All studies were carried out in triplicate in the optimization step.
3.1 Characterization of DES with choline chloride groups
Choline chloride was used as hydrogen bond acceptor to synthesize different types of DES and the effect of hydrogen bonds in the eutectic systems were studied using FT-IR spectroscopy (Fig. S1†). The FT-IR spectra of maleic acid are shown in Fig. 1 as an example. The –OH characteristic peak was shifted from 3440 to 2881 cm−1 in the DES compared with that in maleic acid,27 which can be attributed to the formation of hydrogen bonds between choline chloride and maleic acid. The characteristic peaks of the carbon–oxygen double bond at 1708 cm−1 and the carbon–carbon double bond at 1630 cm−1 were also observed in the DES, indicating that the maleic acid and choline chloride successfully formed the DES.
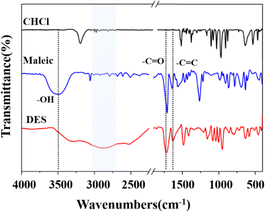 |
| Fig. 1 FT-IR spectra of ChCl, maleic acid, and DES. | |
3.2 Effects of DES composition
The extraction performance of DESs depends on the properties of the constituent HBAs and HBDs. Based on the above, this study further investigated the effect of HBDs on the extraction of metal cations from bamboo litterfall. As shown in Table 2, the extraction recovery of Cd for the glycerin and ethylene based HBDs was 98.6% and 96.2%; the recovery of Fe was only 6% and 7%. This behavior can be attributed to the poor proton activity and complexation ability of alcohol-based DESs. The complexes formed are based on chlorine, which is similar to those of metals in the oxidation state.28,29 It is evident that the carboxylic acid-based HBDs had better extraction performance for heavy metal cations from bamboo litterfall than that of alcohol-based HBDs in the DES system. In particular, maleic acid had the best performance for extraction heavy metal cations from bamboo litterfall. DES based on maleic acid was chosen as the solvent system for the subsequent experiments. The extraction recovery of the maleic acid-based DES for Cd, Cu, Fe, and Zn ions was 98.5%, 88.4%, 90.2%, and 93.7%.
Table 2 Effect of hydrogen bonding types on the DES system on the extraction of Cd, Cu, Zn, and Fe (n = 3)
Hydrogen bonding types |
Extraction recovery (%) |
Cd |
Cu |
Fe |
Zn |
Glycerin |
98.5 ± 2.1 |
36.4 ± 2.4 |
7.3 ± 1.3 |
88.4 ± 1.8 |
Ethylene glycol |
96.0 ± 3.2 |
37.2 ± 3.6 |
6.1 ± 2.1 |
78.7 ± 1.5 |
Formic acid |
83.8 ± 3.4 |
85.3 ± 3.3 |
79.4 ± 3.2 |
82.2 ± 3.5 |
Acetic acid |
89.3 ± 2.6 |
82.1 ± 1.8 |
65.9 ± 2.9 |
85.8 ± 1.6 |
Malic acid |
90.0 ± 3.7 |
82.0 ± 3.6 |
83.4 ± 3.0 |
79.6 ± 3.1 |
Lactic acid |
90.8 ± 2.2 |
80.0 ± 1.2 |
86.3 ± 2.2 |
83.6 ± 2.4 |
Malonic acid |
84.7 ± 2.8 |
79.3 ± 1.7 |
86.9 ± 3.2 |
80.1 ± 5.1 |
Maleic acid |
98.3 ± 2.3 |
89.6 ± 1.4 |
86.1 ± 3.1 |
90.4 ± 2.8 |
The main product formed by the reaction of a metal with a DES is a metal chlorate [MxClO(carboxylic acid)].30 Complexation with organic anions occurs in two stages.31 In the first stage, the carboxylic acid chemically adsorbs to the active site of the metal complex. When the stability of the metal–ligand carboxyl complex is stronger than that of the metal–OH complex, the complex anion will be substituted. In the second stage, the chemical bond energy of the metal complex is weakened during protonation, resulting in dissolution of the heavy metal cations in solution. Based on the above, carboxylic acid-based DESs have a stronger ability to react with the heavy metal cations. In this case, the chelating complex of the multi-charged cations with carboxylic acids is the main driving force for efficient mass transfer.
3.3 Effects of DES synthesis ratio and use for extraction of heavy metal elements
The dissolution of heavy metal cations in the DES-mediated extraction depended on the proton activity and complexation capacity of the DES. The synthesis ratio of HBDs and HBAs greatly affected the extraction performance of the DES system. The choline chloride–maleic acid systems were measured with four different molar ratios of components (2
:
1, 1.5
:
1, 1
:
1, 1
:
2), while maintaining the standard sample weight of 0.10 g. As shown in Table 3, decreasing the choline chloride content in the synthesis process, the extraction recovery of the four heavy metal cations first increased and then decreased. The DES synthesized with choline and maleic acid at a 1
:
1 molar ratio clearly showed the best extraction performance. The extraction recovery reached 100% and 95.4% for Cd and Zn. As previously stated, the protons of carboxylic acids act as good oxygen acceptors in metal complexes, leading to the formation of chlorinated substances with metals. This property was relatively unchanged when the relative composition of maleic acid was increased to 1
:
2 (ChCl
:
maleic). As the molar ratio of maleic acid was increased, the solid components of ChCl–maleic solvents formed liquid eutectics at higher temperatures, resulting in the DES becoming unable to form a stable homogeneous liquid at 80 °C. The molar ratio of 1
:
1 (ChCl
:
maleic) was selected as the optimal DES composition for the extraction of metals from plant litter samples.
Table 3 Effect of the composition of DES on the extraction of Cd, Cu, Zn, and Fe (n = 3)
DES composition (ChCl : MA) |
Extraction recovery (%) |
Cd |
Cu |
Fe |
Zn |
1 : 2 |
91.1 ± 1.2 |
85.5 ± 1.8 |
78.5 ± 2.0 |
84.7 ± 1.2 |
1 : 1.5 |
92.5 ± 1.8 |
86.3 ± 1.5 |
79.8 ± 1.3 |
82.0 ± 2.1 |
1 : 1 |
102.6 ± 1.8 |
87.0 ± 1.5 |
91.2 ± 1.6 |
93.3 ± 2.0 |
2 : 1 |
100.3 ± 1.6 |
88.7 ± 1.7 |
85.5 ± 1.8 |
92.9 ± 3.0 |
The impact of the extraction process on the quantity of DES employed was also examined. The DES added at a weight in the range of 0.50–3.00 g was used to extract the heavy metal cations from a standard sample with a weight of 0.10 g. The extraction recovery first increased with increasing DES content up to a weight of 1.50 g. The extraction recovery of Cd, Cu, Fe, and Zn was 99.1%, 88.2%, 99.9%, and 99.4%. Notably, excess DES led to a low extraction recovery, which can be attributed to increased viscosity of the mixture. This high viscosity hampers the movement of dissolved species, which affects the solvent's conductivity, mass transfer properties, and heat transfer rate32 (Table 4).
Table 4 Effect of the mass of DES on the extraction of Cd, Cu, Zn, and Fe (n = 3)
Mass of DES (g) |
Extraction recovery (%) |
Cd |
Cu |
Fe |
Zn |
0.5 |
83.2 ± 1.2 |
64.6 ± 2.5 |
86.6 ± 1.6 |
79.9 ± 1.5 |
1.0 |
97.9 ± 1.5 |
76.7 ± 2.6 |
93.0 ± 1.4 |
96.3 ± 1.3 |
1.5 |
99.0 ± 2.1 |
88.4 ± 1.3 |
104.8 ± 1.8 |
99.3 ± 1.7 |
2.0 |
82.0 ± 1.6 |
71.7 ± 2.4 |
97.4 ± 2.0 |
83.2 ± 1.4 |
3.0 |
65.5 ± 1.8 |
70.4 ± 1.9 |
84.9 ± 2.0 |
72.6 ± 1.8 |
3.4 Effects of temperature and extraction time
The use of extractants with low viscosity is desirable for extraction because viscosity affects mass transfer between phases. The viscosity not only depends on the chemical structure of the substances in the DES, but also relates to the temperature. As shown in Table 5, the extraction efficiencies of all elements increased as the temperature was increased from 60 to 70 °C, which can be attributed to a decrease in the viscosity of the DES. The decrease in viscosity promotes the dispersion of the metallic elements in the DES phase and increases the proton activity. Separation efficiency tends to decrease with temperatures above 70 °C. This effect is attributed to the destabilization of the hydrogen bonding of DES at high temperatures, which reduces the proton activity in the solution.33
Table 5 Effect of temperature on the extraction of Cd, Cu, Zn, and Fe (n = 3)
Temperature (°C) |
Extraction recovery (%) |
Cd |
Cu |
Fe |
Zn |
60 |
84.8 ± 1.5 |
81.4 ± 1.2 |
93.3 ± 2.1 |
86.8 ± 1.8 |
70 |
89.0 ± 2.1 |
83.7 ± 1.8 |
96.4 ± 1.3 |
90.9 ± 1.7 |
80 |
79.7 ± 1.6 |
75.4 ± 2.0 |
89.5 ± 1.5 |
80.1 ± 1.4 |
90 |
69.1 ± 1.8 |
69.2 ± 2.0 |
88.7 ± 2.5 |
69.9 ± 1.8 |
100 |
82.6 ± 2.2 |
73.6 ± 2.5 |
86.2 ± 1.9 |
82.5 ± 2.4 |
The effect of the extraction time on the DES system was also studied. As shown in Table 6, over a longer extraction time, the extraction efficiency increased at first and then decreased. The highest extraction efficiency was 97.2% achieved at 45 min. Choosing an extraction time of 45 min and extraction temperature of 70 °C as the optimal conditions for separating the metal elements in the analyte.
Table 6 Effect of time on the extraction of Cd, Cu, Zn, and Fe (n = 3)
Time (min) |
Extraction recovery (%) |
Cd |
Cu |
Fe |
Zn |
30 |
97.4 ± 1.2 |
82.5 ± 1.6 |
80.5 ± 2.0 |
89.4 ± 1.5 |
45 |
98.3 ± 1.3 |
89.6 ± 1.6 |
86.1 ± 1.8 |
90.4 ± 2.6 |
60 |
80.7 ± 2.1 |
87.5 ± 1.8 |
86.2 ± 1.9 |
78.4 ± 1.7 |
75 |
78.0 ± 1.6 |
89.1 ± 2.0 |
86.3 ± 1.6 |
78.3 ± 1.4 |
90 |
70.9 ± 1.8 |
86.3 ± 2.0 |
82.1 ± 2.1 |
73.7 ± 1.8 |
3.5 Analytical performance
The linear density, precision and repeatability of the DES-mediated extraction method were examined to evaluate the accuracy of this method. In addition to the litterfall, using the GBW10022 standard, garlic powder, to determine the accuracy of the extraction method and the heavy metal cation concentration were also tested. As shown in Table 7, the recovery of heavy metal elements was greater than 95%, indicating that the obtained results were in agreement with the certified values. A t-test was used to determine the statistical significance of the results. Further analysis showed that there was no significant difference between the measured values and the reference values (confidence intervals all within 95%).
Table 7 Determination of Cu, Fe, Zn, and Cd in GBW 10022 by the proposed method: experimental conditions: volume of 1
:
1(ChCl
:
maleic), 1.5 g; extraction time: 45 min; temperature: 70 °C; sample mass: 100 mg
Element |
Certified/information values (mg kg−1) |
Obtained values (mg kg−1) |
t-test |
Recovery |
Cd |
0.06 ± 0.01 |
— |
— |
— |
Cu |
4.63 ± 0.43 |
4.43 ± 0.06 |
1.16 |
0.95 |
Fe |
205.02 ± 18.32 |
198.29 ± 9.20 |
2.15 |
0.96 |
Zn |
21.72 ± 1.42 |
21.60 ± 1.54 |
0.15 |
0.99 |
The method detection limit (MDL) and LOQ were also calculated. Under the optimal experimental conditions, determining 10 blank sample solutions and used the concentration of the elements to be measured corresponding to 3 times the standard deviation (3SD) of the response value of the blank sample solution as the detection limit. The LOQ was 10 times as high as that of the standard deviation (10SD) of the response value in the blank sample solution. The MDL for Cd, Cu, Fe, and Zn was 0.04, 0.18, 0.70, and 0.59 mg kg−1, which is lower than for the conventional wet digestion methods.34 The LOQ was 0.13, 0.60, 2.30, and 1.96 mg kg−1 for each element.
The reproducibility of the methodology was established by measuring the elemental content in ten labeled samples, all of which had RSD values below 3.40. The reproducibility of the method was assessed by analyzing labeled samples using different instruments and at various time intervals, with RSD values below 6.44 for all elements across all conditions. The parameters of the study are listed in Table 8.
Table 8 Analytical characteristics of the developed technique
Element |
Linear range, (mg kg−1) |
LOD |
LOQ |
R2 |
Repeatability (RSD, n = 10), % |
Reproducibility (RSD, n = 10), % |
Cd |
0.2–200 |
0.04 |
0.13 |
0.99 |
2.32 |
5.76 |
Cu |
1.0–300 |
0.18 |
0.60 |
0.99 |
1.14 |
2.47 |
Fe |
2.0–1000 |
0.70 |
2.30 |
0.99 |
3.40 |
6.44 |
Zn |
0.2–500 |
0.59 |
1.96 |
0.99 |
2.06 |
3.44 |
3.6 Application of the method to the analysis of real samples
The analytical power of the method was demonstrated through its application to the determination of the presence of metals in samples of actual plant litter. The collected litter samples were processed according to the procedure described in the sample pretreatment section. Each sample was individually treated with wet digestion and the DES based on choline chloride–maleic acid followed by ICP-OES analysis, with three repetitions for each sample. As shown in Table 9, the reproducibility of the results obtained based on the ChCl–maleic extraction process was better than that of wet digestion, and the results determined by the DES system and wet digestion were consistent. The accuracy of the present method was also verified. As indicated in Table 9, the extraction efficiency of all elements reached more than 90%. At the same time, the results showed good agreement at the concentrations determined when the sample was dissolved by either DES or wet digestion. In most cases, the reproducibility of the results obtained based on the ChCl–maleic extraction process was better than that obtained by wet digestion. The accuracy of the present method was further verified by comparing it with the method of wet digestion. The Student's t-test showed no significant disparity between the outcomes derived from the developed approach and the wet method at a 95% confidence level. These results indicate that the method of extracting trace heavy metals cations from litterfall by the DES system is reliable.
Table 9 Determination of Cu, Fe, Zn, and Cd contents in litter samples by the DES extraction and wet digestion (concentration in mg kg−1). Experimental conditions: volume of 1
:
1 (ChCl
:
maleic); 1.50 g, extraction time: 45 min; temperature: 70 °C; sample mass: 100 mg
Found metals, mg kg−1 |
Element |
Tamarisk litter |
Bamboo litter |
Deep eutectic solvent-mediated extraction |
Wet digestion |
t-test |
Recovery |
Deep eutectic solvent-mediated extraction |
Wet digestion |
t-test |
Recovery |
Cd |
<LOD |
<LOD |
— |
— |
0.57 ± 0.04 |
0.62 ± 0.02 |
0.58 |
0.91 |
Cu |
30.15 ± 1.56 |
31.22 ± 2.81 |
2.88 |
0.96 |
31.25 ± 0.63 |
33.43 ± 0.71 |
1.50 |
0.93 |
Fe |
466.91 ± 16.52 |
478.21 ± 15.36 |
1.77 |
0.97 |
336.52 ± 4.31 |
369.42 ± 17.42 |
0.91 |
0.91 |
Zn |
33.19 ± 2.47 |
32.69 ± 3.44 |
1.56 |
1.00 |
41.80 ± 0.53 |
41.63 ± 0.57 |
2.63 |
1.01 |
Notably, the energy consumption of this method is only one-fifth than that of the wet digestion method. The energy consumption of the two methods was calculated based on the power of the laboratory equipment and the operating time of the process.35 The energy consumption of the wet digestion method is calculated as:
W = P1 × t = 3 (kW) × 2 (h) = 6 (kW h) |
where
P1is the output power of the graphite ablation furnace and
t is the time for wet digestion.
We also calculated the energy consumption of the DES system as:
W = P2 × (t1 + t2) = 0.85 (kW) × 1.25 (h) = 1.062 (kW h) |
where the
P2 is the output power of the magnetic stirrer;
t1 is the time for the DES synthesis time; and
t2 is the DES extraction time.
The DES-based extraction process was safer because it does not involve the use of high concentrations of acids and high-pressure processes. Thus, the developed approach is a green, safe, and accurate sample preparation method for determination of trace metal elements in environmental samples.
3.7 Comparison with existing methods
The results of the developed method were compared with other reported protocols (as shown in Table S1†) These methods include ultrasound-assisted dispersion liquid–liquid microextraction with flame atomic absorption spectrometry,36 deep eutectic solvent microwave-assisted digestion,24 ternary deep eutectic solvent-based dispersion liquid–liquid microextraction,37 and deep eutectic solvent-based reversed-phase dispersion liquid–liquid microextraction.38 The newly developed method showed good reproducibility compared to the above methods and the ICP-OES based determination showed relatively good LOD. The most valuable advantage of this method is the avoidance of classical organic solvents and the use of green, low eutectic solvents.
4. Conclusions
In summary, the study developed a green, safe, and accurate sample preparation method for the extraction of trace heavy metals in litterfall. A carboxylic acid-based DES showed the highest extraction efficiency of Cd, Cu, Fe, and Zn. The maleic acid-based DES achieved the highest extraction efficiency among all the carboxylic acid-based DES studied. The extraction recovery of the maleic acid-based DES for Cd, Cu, Fe, and Zn was 98.5%, 88.4%, 90.2%, and 93.7%. Under the optimized conditions, the extraction results of GBW10022 were in general agreement with the reference values, confirming the accuracy and precision of the method. The elemental extraction method based on ChCl–maleic (1
:
1) had the shortest extraction time and the lowest energy consumption compared with other digestion methods. Therefore, this novel method addresses two key issues. This method can be used to detect various trace elements in different environmental samples, and provide a reference for environmental risk assessment.
Data availability
Data will be made available on request.
Author contributions
Junlei Zhong: investigation writing – original draft, visualization, methodology, data analysis. Zhikun Wang: investigation. Liang Chen: conceptualization, supervision, writing – review & editing.
Conflicts of interest
The authors declare that they have no known competing financial interests or personal relationships that could have appeared to influence the work reported in this paper.
Acknowledgements
The authors acknowledge National Natural Science Foundation of China (12074341 & 32101493) and Zhejiang Public Welfare Public Research Program (LGC22B010001).
References
- H. Kamyab, M. SaberiKamarposhti, H. Hashim and M. Yusuf, Carbon Lett., 2023, 34, 265–289 CrossRef
. - S. Yavari, H. Kamyab, T. S. Binti Abd Manan, S. Chelliapan, R. Asadpour, S. Yavari, N. B. Sapari, L. Baloo, A. B. C. Sidik and I. Kirpichnikova, Chemosphere, 2022, 303, 134957 CrossRef CAS PubMed
. - M. P. Krishna and M. Mohan, Energy, Ecol. Environ., 2017, 2, 236–249 CrossRef
. - L. El Hosry, N. Sok, R. Richa, L. Al Mashtoub, P. Cayot and E. Bou-Maroun, Foods, 2023, 12, 895 CrossRef CAS PubMed
. - D. d. S. Silva, C. S. dos Santos, L. A. Pando, M. S. R. Gomes, C. G. Novaes, W. N. L. dos Santos and M. A. Bezerra, Food Chem., 2019, 273, 71–76 CrossRef CAS PubMed
. - N. Ncube, Y. Tancu and N. Mketo, J. Food Compos. Anal., 2024, 131, 106201 CrossRef CAS
. - K. Li, C. Jiang, S.-I. Han, S. Kang, J. Chen, D. Won, Y. Kang, B. Bae, Y.-E. Choi, H. S. Kim and J. Lee, Food Chem., 2024, 449, 139196 CrossRef CAS PubMed
. - A. Sheehan and A. Furey, J. Food Compos. Anal., 2024, 9, 100309 Search PubMed
. - R. Serrano, G. Grindlay, L. Gras and J. Mora, Talanta, 2024, 271, 125666 CrossRef CAS PubMed
. - S. Zhao, A. Samadi, Z. Wang, J. M. Pringle, Y. Zhang and S. D. Kolev, Chem. Eng. J., 2024, 481, 148792 CrossRef CAS
. - L. K. S. Gujjala, D. Kundu, D. Dutta, A. Kumar, M. Bal, A. Kumar, E. Singh, R. Mishra, S. Kumar and D.-V. N. Vo, J. Mol. Liq., 2024, 396, 123896 CrossRef CAS
. - M. Khraisheh, F. AlMomani, M. Inamdar, M. K. Hassan and M. A. Al-Ghouti, J. Mol. Liq., 2021, 337, 116421 CrossRef CAS
. - S. Rajendran, S. Hong Loh, M. Mohd Ariffin and W. Mohd Afiq Wan Mohd Khalik, J. Mol. Liq., 2022, 367, 120411 CrossRef CAS
. - S. Dong, G. Huang, Q. Hu, L. Shi, Y. Yao and T. Huang, Chromatographia, 2014, 77, 923–931 CrossRef CAS
. - M. Atilhan and S. Aparicio, Energy Fuels, 2021, 35, 6402–6419 CrossRef CAS
. - S. Yavari, R. Asadpour, H. Kamyab, S. Yavari, S. R. M. Kutty, L. Baloo, T. S. B. A. Manan, S. Chelliapan and A. B. C. Sidik, Clean Technol. Environ. Policy, 2021, 24, 251–260 CrossRef
. - S. Magina, A. Barros-Timmons, S. P. M. Ventura and D. V. Evtuguin, J. Hazard. Mater., 2021, 412, 125215 CrossRef CAS PubMed
. - X. Yang, C. Yan, Y. Sun, Y. Liu, S. Yang, Q. Deng, Z. Tan and X. Wen, TrAC, Trends Anal. Chem., 2022, 149, 116555 CrossRef CAS
. - Q. Xia, Y. Liu, J. Meng, W. Cheng, W. Chen, S. Liu, Y. Liu, J. Li and H. Yu, Green Chem., 2018, 20, 2711–2721 RSC
. - A. Shishov, S. Savinov, N. Volodina, I. Gurev and A. Bulatov, Microchem. J., 2022, 179, 107456 CrossRef CAS
. - J. M. Matong, L. Nyaba and P. N. Nomngongo, Ecotoxicol. Environ. Saf., 2017, 135, 152–157 CrossRef CAS PubMed
. - I. Wazeer, H. F. Hizaddin, M. A. Hashim and M. K. Hadj-Kali, J. Environ. Chem. Eng., 2022, 10, 108574 CrossRef CAS
. - R. Su, S. Tang, M. Zhang and M. Guo, J. Environ. Chem. Eng., 2024, 12, 113200 CrossRef CAS
. - K. Ghanemi, M.-A. Navidi, M. Fallah-Mehrjardi and A. Dadolahi-Sohrab, Anal. Methods, 2014, 6, 1774–1781 RSC
. - A. Shishov, N. Volodina, E. Semenova, D. Navolotskaya, S. Ermakov and A. Bulatov, Food Chem., 2022, 373, 131456 CrossRef CAS PubMed
. - H. Altundag and M. Tuzen, Food Chem. Toxicol., 2011, 49, 2800–2807 CrossRef CAS
. - R. Gautam, N. Kumar and J. G. Lynam, J. Mol. Struct., 2020, 1222, 128849 CrossRef CAS
. - J. M. Hartley, C.-M. Ip, G. C. H. Forrest, K. Singh, S. J. Gurman, K. S. Ryder, A. P. Abbott and G. Frisch, Inorg. Chem., 2014, 53, 6280–6288 CrossRef CAS PubMed
. - Z. Yuan, H. Liu, W. F. Yong, Q. She and J. Esteban, Green Chem., 2022, 24, 1895–1929 RSC
. - A. P. Abbott, D. Boothby, G. Capper, D. L. Davies and R. K. Rasheed, J. Am. Chem. Soc., 2004, 126, 9142–9147 CrossRef CAS
. - S. Mishra, A. Pandey, K. K. Pant and B. Mishra, J. Mol. Liq., 2023, 383, 122142 CrossRef CAS
. - I. D. Boateng, Food Chem., 2023, 406, 135079 CrossRef CAS PubMed
. - J. González-Rivera, C. Pelosi, E. Pulidori, C. Duce, M. R. Tiné, G. Ciancaleoni and L. Bernazzani, Curr. Res. Green Sustainable Chem., 2022, 5, 100333 CrossRef
. - I. O. Akinyele and O. S. Shokunbi, Food Chem., 2015, 173, 682–684 CrossRef CAS
. - B. S. Singh, H. R. Lobo, D. V. Pinjari, K. J. Jarag, A. B. Pandit and G. S. Shankarling, Ultrason. Sonochem., 2013, 20, 287–293 CrossRef CAS
. - M. Rajabi, S. Asemipour, B. Barfi, M. R. Jamali and M. Behzad, J. Mol. Liq., 2014, 194, 166–171 CrossRef CAS
. - S. M. Sorouraddin, M. A. Farajzadeh and H. Dastoori, Talanta, 2020, 208, 120485 CrossRef CAS PubMed
. - S. M. Sorouraddin, M. A. Farajzadeh and T. Okhravi, J. Food Compos. Anal., 2020, 93, 103590 CrossRef CAS
.
|
This journal is © The Royal Society of Chemistry 2024 |
Click here to see how this site uses Cookies. View our privacy policy here.