DOI:
10.1039/D4RA05861E
(Paper)
RSC Adv., 2024,
14, 30873-30885
Utilizing perhalopyridine-based alkynes as suitable precursors for the synthesis of novel poly(1,2,3-triazolyl)-substituted perhalopyridines†
Received
12th August 2024
, Accepted 20th September 2024
First published on 27th September 2024
Abstract
A novel series of poly(1,2,3-triazolyl)-substituted perhalopyridines 5a–f were successfully synthesized from the click reaction of the terminal alkynes (drived from the nucleophilic substitution reactions of PFP 1a and PCP 1b with excess amounts of propargyl alcohol) with aryl azides 4a–c under ultrasonic irradiation. Likewise, the sonication of reaction mixtures containing pyridyl cores 3, alkyl bromides 6a,b, and NaN3 under one-pot conditions afforded their respective aliphatic 1,2,3-triazoles 7a–d in yields ranging from 71% to 83%. We next developed an effective method for the regioselective preparation of 2,3,4,5-tetrachloro-6-(prop-2-yn-1-yloxy)pyridine 3c through SNAr reaction of PCP with propargyl alcohol without the utilization of any catalyst. It was then used to fabricate several ((1,2,3-triazol-4-yl)methoxy)-3,4,5,6-tetrachloropyridines 8a–c under the reaction conditions. Finally, the Pd(PPh3)4-catalyzed SMC reaction of tris-triazoles 5b,e with arylboronic acids 9a–c offered a practical method for the synthesis of biaryl-embedded poly(1,2,3-triazoles) 10a–f in good yields.
1. Introduction
Organofluorines (OFs) exhibit a wide range of applications in liquid crystals,1 agrochemicals,2 electro-optic devices,1a,3 and 19F-magnetic resonance imaging (MRI).4,5a Proteins and peptides tagged with the fluorine-18 isotope are also applied as radiotracers in positron emission tomography (PET).5 On the other hand, Organochlorines (OCs) find extensive use as building blocks for chemical research and industrial uses.6–10 Among these, perhalogenated pyridines offer a high degree of structural diversity and have proven to be useful for searching new materials and therapeutic leads. Using perhalopyridines instead of pyridine itself is a suitable alternative method for the synthesis of substituted-perhalopyridine derivatives. In this regard, the nucleophilic aromatic substitution reactions (SNAr) of pentafluoropyridne (PFP) and pentachloropyridine (PCP) with different species including oxygen,11 nitrogen,12 sulfur,12e,13 and carbon-centered12e–14 nucleophiles have been explored. The site-selectivity of reactions is overally determined by several factors including the positioning of halogen atoms on the pyridine core, solvent, the reaction conditions, as well as the strength and nature of nucleophile and base. In general, the replacement of halogen atoms on the pyridine cores takes place in a selective and step-by-step manner.12e,15 While substitution reaction of PFP with stoichiometric amount of nucleophiles under mildly basic conditions occurs solely at the C-4 position, it can be substituted sequentially at two positions (C-2, C-6) using strong nucleophiles under harshly basic conditions or elevated temperatures. On the basis, Iacono et al. succeeded to synthesize 2,3,5,6-tetrafluoro-[N-(3-aminopropyl)-ε-caprolactam]-4-pyridine via the site-selective reaction of PFP with 1,8-diazabicyclo[5.4.0]undec-7-ene with PFP.16 The controlled regio-selective SNAr reaction of PFP with 4-ethynylphenol and phenol has also been led to the formation of 2,6-bis(4-ethynylphenoxy)-3,5-difluoro-4-phenoxypyridine, as a unique polymer precursor suitable for thermal polymerization.17 Likewise, fluoropyridyl silicone-based oils and network elastomers have been synthesized through hydrosilylation of the derived monomers through regio-controlled functionalization of PFP with alcohols possessing terminal alkenes.18 Alongside these, semifluorinated trifunctional-ene monomers, which play a crucial role in thiol–ene thermoset materials, are synthesized via the site-selective SNAr reactions of PFP with 4-penten-1-ol or eugenol.19 PFP has also been assessed as an activator in the synthesis of acyl fluoride from carboxylic acids.20 Recently, there has been research conducted on the reaction of pentachloropyridine. o-Perhalopyridines have been utilized as amination agents under photocatalyst conditions.21 Among these research, 2′,3′,5′,6′-tetrachloro-4-(dimethylamino)-[1,4′-bipyridin]-1-ium treated with oxygen and nitrogen nucleophile.11e,22 Our team has previously evaluated the site reactivity of PCP with indoles12c and N-substituted sulfonamides12d as potential bioactive candidates. Another report is presented on the intramolecular cyclization of 4-phenylsulfonyl-2,3,5,6-tetrachloropyridine, which results in the rapid formation of a range of novel benzothienopyridine-S,S-dioxide frameworks under gentle reaction conditions.23 Ehlers et al.24 investigated the site-selective reaction of pentachloropyridine and aryl boronic acid for the synthesis of mono- and disubstituted pyridine rings. The cross-coupling reaction between cyclobutanone O-perchloropyridin-4-yl oxime and nitrostyrene presents an environmentally sustainable approach for the production of cyanoalkylated alkenes.25
1,2,3-Triazoles are receiving increasing interest of both academia and industry for their applications in the synthesis of dyes,26 photographic materials,27 agrochemicals,28 photo-stabilizers,29 and as linkers for binding two biologically potent scaffolds.30 Although 1,2,3-triazole motifs are not normally found in nature, versatile biological activities have been specified for them.31–37 Copper(I)-catalyzed 1,3-dipolar cycloaddition (CuAAC) reaction of organic azides with terminal alkynes has become an efficient and rapid method for the regioselective synthesis of 1,4-disubmitted 1,2,3-triazoles (Scheme 1a).30d,38 On the other hand, the introduction of polytriazole moieties into chemical structures has been proved to be a beneficial strategy in the synthesis of macromolecular 1,2,3-triazole-based functional materials and drug design.39 For instance, a number of synthesized bistriazoles have been considered as potent antibacterial, antifungal and plasmin inhibitors.39a,40 Xiong et al. have succeeded to synthesize a 1,3,5-tri(1H-benzo[d]imidazole-2-yl)benzene(TBB)-based tris(1,2,3)triazole as a selective and highly sensitive fluorescent chemosensor for the detection of picric acid (Scheme 1b).41 Gallic acid-based tris(1,2,3-triazole)s have also been made by a click reaction to form their respective supramolecular columnar liquid crystals (Scheme 1c).42 likewise, tris-(benzyltriazolylmethyl)amines have been offered as powerful stabilizing ligand for copper(I).39g Moreover, various state-of-the-art click chemistry strategies have been developed for the formation of poly(1,2,3-triazole)-based materials with interesting application in molecular recognition, chemical sensing, drug chemistry, biochemistry, and conducting materials.39c–e,43 Despite all these achievement and progress, there are a few reports on the synthesis of non-polymeric poly(1,2,3-triazole) analogues. Furthermore, the incorporation of perhalopyridine units in 1,2,3-triazole scaffolds opens new avenues for seeking new ploytriazoles with sought after functions in materials science and medicinal chemistry. On the basis and in continuation of our ongoing research on perhalopyridines,11a,b,44 we were interested to report our findings on the synthesis of a novel series of tris(1,2,3-triazolyl)-substituted perhalopyridines (Scheme 1d).
 |
| Scheme 1 An overview. | |
2. Results and discussion
2.1. Synthesis poly(1,2,3-triazolyl)-substituted perhalopyridine derivatives
Our initially efforts were directed towards the synthesis of perhalopyridine-based alkyne precursors. As depicted in Table 1, 3,5-difluoro-2,4,6-tris(prop-2-yn-1-yloxy)pyridine 3a and 3,5-dichloro-2,4,6-tris(prop-2-yn-1-yloxy)pyridine 3b were synthesized through the nucleophilic substitution reactions of PFP 1a and PCP 1b with propargyl alcohol 2, respectively. We first aimed to find the best reaction conditions for the preparation of precursor 3a. In this respect, the reaction of 1a and propargyl alcohol was carried out under different conditions and the results are shown in Table 1. Stirring a mixture of PFP (1 mmol), 2 (3 mmol), and K2CO3 (3.9 mmol) in various solvents including CH3CN, DMF, DMSO, and THF at room temperature for 48 hours, led to the producing of 3a in yield ranging from 28% to 60% (entries 1–4). Hence, performing the reaction in DMF solvent using higher molar ratios of reactants was investigated to improve the efficiency of the reaction. While, the treatment of PFP (1 mmol) with 2 (6 mmol) and K2CO3 (7.8 mmol) in DMF at r.t for 48 hours afforded product 3a in the yield of 81% (entry 5), a similar efficacy was also observed when PFP and 2 were used in a 1
:
9 molar ratio (entry 6). However, we succeeded to isolate product 3a in a yield of 93% by lengthening the reaction time to 72 hours from the r.t reaction of PFP (1 mmol) with 2 (6 mmol) and K2CO3 (7.8 mmol) in DMF (entry 7). In continuation, the influence of several factors such as solvent, reactants' molar ratio, and reaction temperature was studied on the reaction of PCP and 2 in the presence of K2CO3 as base (Table 1, entries 8–15). Given the corresponding optimal conditions (Table 1, entry 15), a mixture of PCP (1 mmol), 2 (9 mmol), and K2CO3 (11.7 mmol) in DMF was heated at 60 °C for 24 hours and product 3b was obtained in yield of 83%. The validation of chemical structures for 3a and 3b was verified through IR and NMR, analyses. The IR spectrum of 3a and 3b showed an absorbance bond for the corresponding terminal acetylenic group, respectively in 2127, and 2123 cm−1. The NMR spectrum of these cores was in good agreement with the assigned structures. Appearing a single resonance at δ = −164.37 ppm of the 19F-NMR spectrum of 3a indicates the displacement of the propargyl alcohol in three positions of the PFP ring. The 1H-NMR spectra of 3a and 3b exhibit a high degree of resemblance, except for certain variations observed in their chemical shifts. For example, the 1H-NMR spectrum of 3b shows two triplet peaks at δ = 3.67, and δ = 3.56, with a relative integration ratio of 1
:
2, which are due to the terminal acetylenic hydrogens. Furthermore, the presence of two doublet peaks at δ = 4.95, and δ = 5.07 ppm are attributed to methylenic hydrogens attached to oxygens. The 13C-NMR spectrum of compound 3b showed 9 distinct signals in agreement with the proposed target molecule. Two appeared signals at δ = 55.54 and δ = 61.29 ppm are related to the methylenic groups, and three signals in the range of 78.05–80.32 ppm were reported due to the presence of the acetylenic carbons. The structure elucidation of the product 3a was also done by single-crystal X-ray analysis (Scheme 2).
Table 1 Screening on the reaction of perhalopyridine 1 with propargyl alcohol 2

|
Entry |
1 (mmol) |
2 (mmol) |
K2CO3 (mmol) |
Solvent |
Time (h) |
T (°C) |
Yielda (%) |
Isolated yield. |
1 |
1a (1) |
3 |
3.9 |
CH3CN |
48 |
r.t |
50 |
2 |
1a (1) |
3 |
3.9 |
DMF |
48 |
r.t |
60 |
3 |
1a (1) |
3 |
3.9 |
DMSO |
48 |
r.t |
56 |
4 |
1a (1) |
3 |
3.9 |
THF |
48 |
r.t |
28 |
5 |
1a (1) |
6 |
7.8 |
DMF |
48 |
r.t |
81 |
6 |
1a (1) |
9 |
11.7 |
DMF |
48 |
r.t |
89 |
7 |
1a (1) |
6 |
7.8 |
DMF |
72 |
r.t. |
93 |
8 |
1b (1) |
3 |
3.9 |
CH3CN |
72 |
r.t |
23 |
9 |
1b (1) |
3 |
3.9 |
DMF |
72 |
r.t |
28 |
10 |
1b (1) |
3 |
3.9 |
DMSO |
72 |
r.t |
25 |
11 |
1b (1) |
3 |
3.9 |
THF |
72 |
r.t |
12 |
12 |
1b (1) |
3 |
3.9 |
DMF |
24 |
60 |
50 |
13 |
1b (1) |
3 |
3.9 |
DMF |
24 |
80 |
35 |
14 |
1b (1) |
6 |
7.8 |
DMF |
24 |
60 |
80 |
15 |
1b (1) |
9 |
11.7 |
DMF |
24 |
60 |
83 |
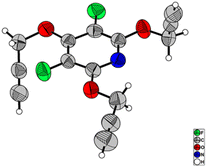 |
| Scheme 2 Crystal structure of 3a. | |
The chemical community is interested in preparing poly-triazoles for various applications.39–43 On the other hand, incorporation of halogen atom(s) into organic compounds and polymers have been introduced as a powerful method to change or improve the chemical, physical and/or biological activity of a desired molecule. On the basis, it was objected to produce a novel series of poly(1,2,3-triazolyl)-substituted perhalopyridines. First, the required aryl azides 4a–c for producing triazolyl scaffolds were prepared using methods described in the literature.45 Then, the reaction between 3a and benzyl azide 4a for the clickable synthesis of compound 5a was chosen as a model and the effect of parameters such as solvent, copper catalysts, and reaction conditions were investigated on the efficiency of the reaction. The results are summarized in Table 2. The reaction of precursor 3a (3.5 mmol) with benzyl azide 4a (11.5 mmol) in the presence of CuSO4·5H2O (5 mol%) and NaAsc (15 mol%) in CH3CN at room temperature afforded a trace amount of 2,4,6-tris((1-benzyl-1H-1,2,3-triazol-4-yl)methoxy)-3,5 difluoropyridine 5a after 24 hours. While performing this reaction in DMF solvent led to the production of 5a in 23% yield, utilizing DMF/H2O (1
:
1) provided an improvement in the yield of reaction (entries 1 and 2). Accordingly, carrying out the model reaction in several aqueous medium involving THF/H2O (1
:
1), t-BuOH/H2O (1
:
1), and CH2Cl2/H2O (1
:
1) was done and the product 5a was obtained in yields ranging from 43% to 90% (entries 4–7). On this basis, CH2Cl2/H2O (1
:
1) was considered as the best solvent of choice (entry 6). Our next experiments showed that the replacement of CuSO4·5H2O with other copper catalysts including CuI and Cu(OAc)2 reduces the yield of product 5a. Furthermore, the importance of copper catalyst for the construction of triazole scaffolds was demonstrated when no reaction progress was observed in the treatment of 3a with 4a in the absence of copper catalysts (entry 8). As reported in the literature, the necessity of Cu(II) for this reaction is due to its insitu reduction to Cu(I) required for the Copper-Catalyzed Azide–Alkyne Cycloaddition (CuAAC) reactions. Furthermore, the model reaction was carried out under ultrasonic irradiation, and the sonication procedure was identified to be a powerful alternative to the conventional method. In general, the product 5a was obtained in higher yields and shorter reaction times (entries 1–10). When we increased the amount of copper catalyst, the reaction yield did not change (entry 7). Accordingly, we proceeded to investigate the preparation of tris(1,2,3-triazolyl)-substituted perhalopyridines via the reaction of precursors 3 (3.5 mmol) with aryl azides 4a–c (11.5 mmol) in the presence of CuSO4·5H2O (5 mol%) and NaAsc (15 mol%) in CH2Cl2/H2O (1
:
1) under ultrasonic irradiation. Lukily, treatment of 3a with 1-(azidomethyl)-4-bromobenzene 4b and 1,4-bis(azidomethyl)benzene 4c under optimal conditions afforded tris(1,2,3-triazolyl)-substituted perfluoropyridines 5b and 5c in 95% and 90% yields, respectively. In a similar way, tris(1,2,3-triazolyl)-substituted perchloropyridines 5d–f were successfully obtained through the reaction of precursor 3b and aryl azides 4a–c in yields ranging from 90–94% (Scheme 3). The newly synthesized compounds (5a–f) were characterized based on their infrared spectrum as well as 1H, 13C, and 19F-NMR spectra. For example, the structure of tris-triazolyl 5a was demonstrated by the absence of acetylenic bond in the FT-IR spectrum. The 1H-NMR spectrum of 5a shows a single sharp peak at δ = 8.28 ppm due to the triazolyl-CH groups, and three singlets at δ = 5.40 (s, 2H, CH2–N) and δ = 5.44 (s, 4H, CH2–N), δ = 5.59 (s, 6H, CH2O) which are related to the three nonequivalent series of methylene bridges. Similarly, the tris-1,2,3-triazole system exhibits a consistent arrangement of aromatic patterns in all instances (refer to the experimental section for more details).
Table 2 Optimization of the conditions for the reaction of 3a and 4a to form 1,2,3-triazole 5aa
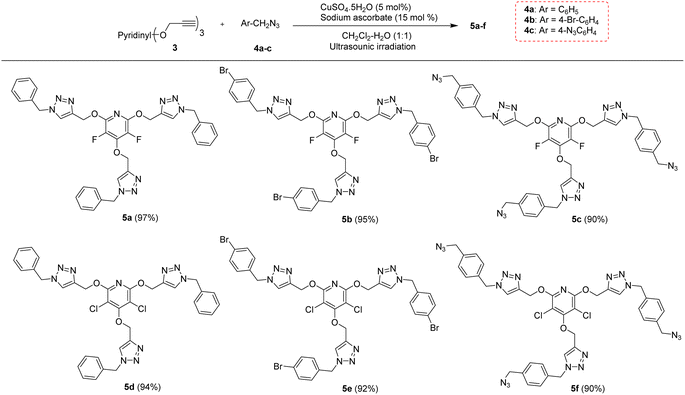 |
| Scheme 3 The chemical structures of synthesized tris(1,2,3-triazolyl)-substituted perhalopyridines 5a–f. | |
Encouraged by the above results, it was hypothesized that this methodology could be expanded to generate tris(1,2,3-triazoles) containing perhalopyridine moieties using aliphatic triazides 6. To achieve aliphatic triazoles 7, the reaction of precursor 3a and ethyl 2-azidoacetate 6a was studied to find the optimal reaction conditions. It was found tris(triazole) 7a does not form a satisfactory yield applying the optimal conditions used for the synthesis of tris(1,2,3-triazoles) 5a–f. So, we decided to improve the yield of product 7a through the one-pot reaction of 3a with a small excess amount of sodium azide and aliphatic bromide in the presence of a copper(II) catalyst with 5 mol% loading under sonication, and the results are shown in Table 3. The corresponding tris(triazole) 7a was obtained in the highest yield via the one-pot click reaction 3a (1 mmol), sodium azide (3.6 mmol), ethyl 2-bromoacetate 6a (3 mmol), CuSO4·5H2O (5 mol%) and sodium ascorbate (15 mol%) in tBuOH/H2O (1
:
3) under ultrasonic irradiation for 8 hours at 60 °C (Table 3, entry 5). Under these conditions, precursor 3a was also reacted with 3-bromoprop-1-ene 6b for 8 hours and the corresponding aliphatic triazole 7b was isolated in 83% yield (Scheme 3). Similarly, pyridyl core 3b was submitted to the reaction with ethyl 2-bromoacetate 6a and bromoprop-1-ene 6b, and their respective tris(triazoles) 7c and 7d were obtained in yields of 78% and 71%, respectively (Scheme 4). This study highlighted the challenges and outcomes of synthesizing tris(triazoles) with perfluoropyridine moieties, emphasizing the importance of reaction conditions and reactant selection for achieving the desired products, efficiently.
Table 3 Investigation of the optimal conditions for the reaction of 3a with ethyl 2-bromoacetate 6a
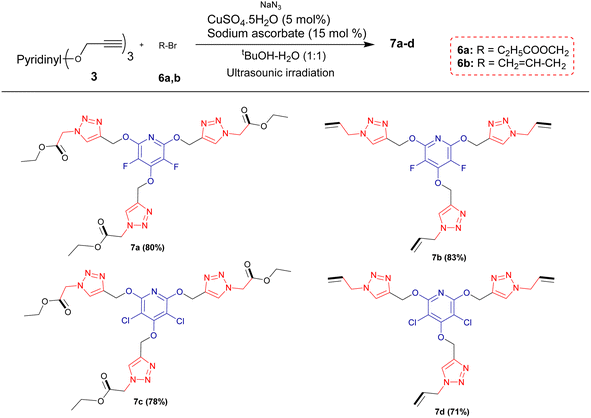 |
| Scheme 4 Synthesis of aliphatic tris(1,2,3-triazoles) bearing perhalopyridine moieties. | |
2.2. Synthesis of 2-((1-benzyl-1H-1,2,3-triazol-4-yl)methoxy)-3,4,5,6-tetrachloropyridine derivatives
Due to the significance of site-selective nucleophilic substitution reactions involving pentachloropyridine,44 particularly position 4 as the primary preference against nucleophilic attacks, herein, we describe, for the first time, the direct execution of nucleophilic substitution at the C-2 position of pentachloropyridine without the utilization of any catalyst. In this respect, we were interested in synthesizing 2,3,4,5-tetrachloro-6-(prop-2-yn-1-yloxy)pyridine 3c through regioselective nucleophilic substitution reaction of pentachloropyridine with propargyl alcohol under those conditions reported in section 2.1 but using PCP and propargyl alcohol in a 1
:
1 molar ratio. Accordingly, the reaction of PCP (1 mmol) with 2 (1 mmol) in the presence of K2CO3 (1.3 mmol) in DMF at 60 °C for 24 hours afforded product 3c in 83% yield (Scheme 5). The derived product 3c (1 mmol) was, then, submitted to the reactions with aryl azides 4a–c (1.2 mmol) in the presence of CuSO4·5H2O (5 mol%) and NaAsc (15 mol%) in CH2Cl2/H2O (1
:
1) under ultrasonic irradiation at 60 °C for 8 hours and their respective ((1,2,3-triazol-4-yl)methoxy)-3,4,5,6-tetrachloropyridines 8a–c were synthesized in yields of 78–89% (Scheme 6). Furthermore, the structure of 8b was confirmed by X-ray crystallographic data (Scheme 7). The structure elucidation of 3a was first performed by infrared and NMR spectroscopy. In the FT-IR spectrum of product 3c, the absorption band of the acetylenic group is observed at 2132 cm−1. The 1H-NMR spectrum of 3c shows two distinct peaks at δ = 3.65, and δ = 5.05 ppm which are attributed to the acetylenic and methylenic hydrogens, respectively. In the 13C-NMR, five signals appearing in the region δ = 117.04–155.94 ppm are related to the fourth type carbons of the pyridine ring, and three distinct signals in the δ = 79.19, δ = 78.36, and δ = 56.46 ppm can be attributed to the presence of acetylenic and methylenic groups, respectively.
 |
| Scheme 5 Site-selective synthesis of 2,3,4,5-tetrachloro-6-(prop-2-yn-1-yloxy)pyridine 3c. | |
 |
| Scheme 6 Synthesis of ((1,2,3-triazol-4-yl)methoxy)-3,4,5,6-tetrachloropyridine 8a–c derivatives. Reaction conditions: 3c (1 mmol) was, then, aryl azides 4a–c (1.2 mmol), CuSO4·5H2O (5 mol%), NaAsc (15 mol%) in CH2Cl2/H2O (1 : 1) under ultrasonic irradiation at 60 °C for 8 hours. | |
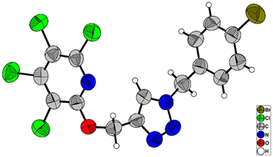 |
| Scheme 7 X-ray crystal structure of 8b. | |
2.3. Synthesis of biaryl-embedded perhalopyridine-based poly(1,2,3-triazoles)
Biaryls are an integral part of various natural products46 and biologically active compounds.47 They are also fundamental building blocks46d in organic synthesis and material chemistry. Biaryl scaffolds are traditionally formed via the transition-metal catalyzed Suzuki–Miyaura coupling (SMC) reactions of aryl boronic acids with aryl halides.48 After the successful synthesis of tris(1,2,3-triazoles) 5b or 5e, we aimed to synthesis their respective biaryl-embedded 1,2,3-triazoles. Therefore, the Suzuki–Miyaura cross-coupling (SMC) reaction of tris-triazole 5b and phenylboronic acid 9a in the presence of Pd(PPh3)4 was chosen as a model, and the effect of different variants including solvent, base, molar ratios of substrates and catalyst loading was explored under thermal conditions. The results are listed in Table 4. In the best conditions, the coupling product 10a was obtained in yield of 72% through stirring a mixture of 5a (1 mmol), phenylboronic acid 9a (3.8 mmol), Cs2CO3 (4.5 mmol), and [Pd(PPh3)4] (0.045 mol%) in DMF/H2O (1
:
3) at 70 °C for 24 hours. Under these conditions, tris(1,2,3-triazolyl)-substituted perfluoropyridine 5b was also reacted with 2-naphthylboronic acid 9b to achieve the corresponding biaryl-embedded perfluoropyridine-based poly(1,2,3-triazoles) 10b in 70% yield. However, in the treatment of 5b with 4-formylphenylboronic acid 9c under optimized conditions, the coupling reaction took place at only one position, and product 10c gained in yield of 62% (Scheme 7). In a similar way, the reaction of tris(1,2,3-triazolyl)-substituted perchloropyridine 5e with arylboronic acids 9a–c afforded their respective biaryl-embedded perchloropyridine-based poly(1,2,3-triazoles) 10d and 10e in good yields (Scheme 8). As similar to 5b, aryl bromide 5e was coupled with 4-formylphenylboronic acid 9c at only one position under optimal conditions, and the corresponding product 10f was isolated in a yield of 56%. The structures of coupling products were confirmed using FT-IR and NMR analyses.
Table 4 Screening of the reaction conditions for the SMC reaction of compound 5b with phenylboronic acid 9aa
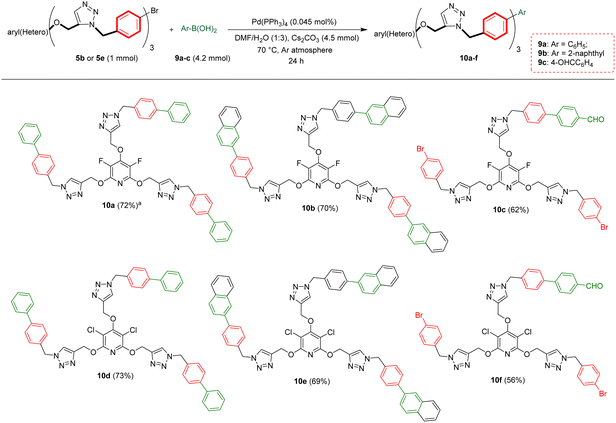 |
| Scheme 8 Pd(PPh3)4 catalyzed Suzuki–Miyaura coupling reaction of aryl bromides 5b and 5e with arylboronic acids 9a–c. aIsolated yield. | |
3. Experimental details
3.1. General information
All chemicals purchased from Merck chemical company and used without further purification unless specified. The advancement of the reactions was tracked through TLC analysis on polyester sheets coated with silica gel-60 and fluorescent indicator (F-252) obtained from Merck. Melting points were conducted on a Stuart SMP2 apparatus and left uncorrected. IR spectra were recorded on a Nicolet-Impact 400D spectrophotometer using KBr pellets. 1H-, 13C-, and 19F-NMR spectra were recorded on a Bruker Ultrashield-400 NMR spectrometer using DMSO-d6 as a solvent, while those for 19F were reported in ppm relative to CFCl3 as the standard. The ultrasonic device used was an UP 400 S instrument from Dr Hielscher GmbH. An S3 immersion horn emitting 24 kHz ultrasound at intensity levels tunable to maximum sonic power density of 460 W cm2 was used. Sonication was carried out at 100% (maximum amplitude 210 lm). A 3 mm long sonotrode (maximum immerse depth of 90 mm) was immersed directly into the reaction mixture.
3.2. Synthesis of 3,5-difluoro-2,4,6-tris(prop-2-yn-1-yloxy)pyridine 3a
In a 25 mL round-bottomed flask, a mixture of propargyl alcohol (6 mmol), K2CO3 (7.8 mmol), and DMF (5 mL) was stirred at room temperature for 30 minutes. Next, PFP (1 mmol) was added and the resulting mixture was stirred at room temperature for 72 hours. Then, the reaction mixture was poured into 10 mL water and extracted with ethyl acetate (2 × 30 mL). The organic phase was dried over MgSO4, and the solvent evaporated. The pure product 3a was obtained in 93% yield after washing with hexane.
Light yellow solid. Yield 93%. MP 118–120 °C; IR (KBr)
3283, 2943, 2127, 1632 cm−1. 1H NMR (500 MHz, DMSO-d6) δ 5.08 (d, J = 2.4 Hz, 2H, CH2–O), 5.02 (d, 4H, J = 2.4 Hz, CH2–O), 3.72 (t, 1H, J = 4.9 Hz, acetylene-H), 3.54 (t, J = 4.9 Hz, 2H, acetylene-H) ppm. 13C-NMR (126 MHz, DMSO-d6) δ 144.16 (dm, 2JCF = 10.90 Hz, C2,6-py), 143.45 (d, 2JCF = 10.40 Hz, C4-py), 134.24 (d, 1JCF = 248.9 Hz, C3,5-py), 80.53 (acetylene-CH), 79.20 (acetylene-C), 78.42 (acetylene-CH), 78.13 (acetylene-C), 61.55 (CH2–O), 54.81 (CH2–O) ppm. 19F NMR (470 MHz, DMSO-d6) δ −164.37 (2F, F3,5-py).
3.3. Synthesis of 3,5-dichloro-2,4,6-tris-prop-2-ynyloxy-pyridine 3b
In a 25 mL round-bottomed flask, a mixture of propargyl alcohol (9 mmol), K2CO3 (11.7 mmol), and DMF (5 mL) was stirred at room temperature for 30 minutes. Next, PCP (1 mmol) was added and the resulting mixture was stirred at 60 °C for 24 hours. Then, the reaction mixture was poured into 10 mL water and extracted with ethyl acetate (2 × 30 mL). The organic phase was dried over MgSO4, and the solvent evaporated. The pure product 3b was obtained in 83% yield after washing with hexane.
Light brown solid. Yield 83%. MP 121–124 °C. IR (KBr)
3277, 2513, 2123, 1796 cm−1. 1H NMR (500 MHz, DMSO-d6) δ 5.07 (d, 4H, J = 2.4 Hz, CH2–O), 4.95 (d, 2H, J = 2.4 Hz, CH2–O), 3.67 (t, 1H, J = 5 Hz, acetylene-H), 3.56 (t, 2H, J = 5 Hz, acetylene-H) ppm. 13C NMR (126 MHz, DMSO-d6) δ 160.30, 155.04, 104.19, 80.32 (acetylene-CH), 79.07 (acetylene-C), 78.53 (acetylene-CH), 78.05 (acetylene-C), 61.29 (CH2–O), 55.54 (CH2–O) ppm.
3.4. General procedure for the synthesis of aryl azides 4a–c (ref. 45)
Aryl azides 4a–c were prepared according to the reported method in the literature.41 To a stirred solution of NaN3 (5.5 mmol) in DMSO (5.0 mL) was added benzyl bromide (5.0 mmol). The resulting mixture was stirred at 80 °C overnight. The reaction mixture was then cooled to room temperature and diluted with water (15 mL), it was extracted with diethyl ether (3 × 10 mL) and washed with brine, dried over MgSO4, and concentrated under a vacuum to give the products 4a–c as colorless liquids in quantitative yields. They were used directly without further purification.
3.5. General procedure for the synthesis of tris(1,2,3-triazoles) 5a–f under ultrasonic irradiation
Precursors 3a,b (3.5 mmol) was dissolved in CH2Cl2/H2O (5 mL, 1
:
1), aryl azides 4a–c (11.5 mmol), CuSO4·5H2O (5 mol%) and NaAsc (15 mol%) were added and the resulting mixture was sonicated at 60 °C for 8 hours. The completion of the reaction was controlled by TLC until the consumption of precursors 3. Then, H2O (5 mL) was added to the mixture, and the precipitate was collected by filtration, washed thoroughly with H2O, and CH2Cl2, and dried under vacuum. The crude was purified by column chromatography using CH2Cl2/MeOH (95/5) as eluent to obtain products 5a–f.
2,4,6-Tris((1-benzyl-1H-1,2,3-triazol-4-yl)methoxy)-3,5-difluoropyridine 5a. White solid. Yield 97%. MP 165–168 °C. IR (KBr)
3726, 3630, 3448, 3137, 3087, 3032, 2956 cm−1. 1H NMR (500 MHz, DMSO-d6): δ 8.29 (s, 3H, tetrazole-H), 7.26–7.40 (m, 15H, Ar–H), 5.59 (s, 6H, CH2O), 5.44 (s, 4H, CH2–N), 5.40 (s, 2H, CH2–N). 13C NMR (126 MHz, DMSO-d6): δ 144.90 (dm, 2JCF = 10.01 Hz, C2,6-py), 143.94 (d, 2JCF = 8.67 Hz, C4-py), 143.03 (triazole-C), 142.38 (triazole-C), 136.42 (Ar–C), 134.0 (d, 1JCF = 247.5 Hz, C3,5-py), 129.21 (Ar–CH), 129.18 (Ar–CH), 128.61 (Ar–CH), 128.56 (Ar–CH), 128.46 (Ar–CH), 128.17 (Ar–CH), 125.9 (alkene-CH), 125.4 (alkene-CH), 66.67 (CH2–O), 60.03 (CH2–O), 53.32 (CH2–N), 53.26 (CH2–N) ppm. 19F NMR (470 MHz, DMSO-d6) δ −164.61 (2F, F3,5-py).
2,4,6-Tris((1-(4-bromobenzyl)-1H-1,2,3-triazol-4-yl)methoxy)-3,5-difluoropyridine 5b. White solid. Yield 95%. MP 184–186 °C; IR (KBr)
3725, 3431, 1626, 1491 cm−1. 1H NMR (500 MHz, DMSO-d6) δ 8–30 (s, 3H, tetrazole-H), 7.55 (d, J = 8.3 Hz, 6H, Ar–H), 7.27 (d, J = 8.4 Hz, 4H), 7.20 (d, J = 8.5 Hz, 2H), 5.58 (s, 6, CH2O), 5.46 (s, 4H, CH2–N), 5.40 (s, 2H, CH2–N) ppm. 13C NMR (125 MHz, DMSO-d6): 144.91 (m, C2,6-py), 144.01 (m, C4-py), 143.08, 142.43, 135.78, 135.01 (d, 1JCF = 247.5 Hz, C3,5-py), 133.03, 132.12, 130.66, 130.49, 125.84 (alkene-CH), 125.45, 121.90, 121.87 (alkene-CH), 66.65 (CH2–O), 60.01 (CH2–O), 52.58 (CH2–N), 52.55 (CH2–N) ppm. 19F NMR (470 MHz, DMSO-d6) δ −164.62 (2F, F3,5-py).
2,4,6-Tris((1-(4-(azidomethyl)benzyl)-1H-1,2,3-triazol-4-yl)methoxy)-3,5-difluoropyridine 5c. White solid. Yield 90%. MP 200 °C (dec); IR (KBr)
3726, 3630, 3448, 3137, 2927, 2099, 1626 cm−1. 1H NMR (500 MHz, DMSO-d6) δ 8–26 (s, 3H, tetrazole-H), 7.33–7.35 (m, 12H, Ar–H), 5.60 (s, 6, CH2O), 5.45 (s, 4H, CH2–N), 5.40 (s, 2H, CH2–N), 4.46 (s, 2H, CH2–N3), 4.41 (s, 4H, CH2–N3) ppm. 13C NMR (126 MHz, DMSO-d6) 162.71, 143.87 (m), 142.99, 142.37 (m), 142.33, 136.28, 136.10, 135.99 134.18 (d, 1JCF = 203.75 Hz, C3,5-py), 129.17, 128.73, 128.50, 125.39 (alkene-CH), 66.61 (CH2–O), 60.01 (CH2–O), 53.72 (CH2–N), 52.92 (CH2–N), 36.19, 31.20 ppm. 19F NMR (470 MHz, DMSO-d6) δ −164.62 (2F, F3,5-py).
2,4,6-Tris((1-benzyl-1H-1,2,3-triazol-4-yl)methoxy)-3,5-dichloropyridine 5d. White solid. Yield 94%. MP 175–177 °C; IR (KBr)
3726, 3449, 3132, 3066, 3032, 2953, 1576 cm−1. 1H NMR (500 MHz, DMSO-d6) δ 8.33–8.35 (3H, tetrazole-H), 7.27–7.36 (m, 15H, Ar–H), 5.60 (6, CH2O), 5.50 (4H, CH2–N), 5.22 (2H, CH2–N) ppm. 13C-NMR (126 MHz, DMSO-d6) δ 160.79, 155.75, 142.89, 136.45, 136.40,129.20 (Ar–CH), 129.15 (Ar–CH), 128.87 (Ar–CH), 128.60 (Ar–CH), 128.52 (Ar–CH), 128.43 (Ar–CH), 128.15 (alkene-CH), 125.99, 125.42, 103.67 (alkene–CH), 66.57 (CH2–O), 60.77 (CH2–O), 53.31 (CH2–N), 53.23 (CH2–N) ppm.
2,4,6-Tris((1-(4-bromobenzyl)-1H-1,2,3-triazol-4-yl)methoxy)-3,5-dichloropyridine 5e. Light brown solid. Yield 92%. MP 200–203 °C; IR (KBr)
3841, 3725, 3448, 3061, 2953 cm−1. 1H NMR (500 MHz, DMSO-d6): δ 8–30 (s, 3H, tetrazole-H), 7.51–7.55 (m, 6H, Ar–H), 7.19–7.29 (m, 6H, Ar–H), 5.58 (s, 6, CH2O), 5.51 (s, 4H, CH2–N), 5.21 (s, 2H, CH2–N) ppm. 13C-NMR (126 MHz, DMSO-d6) δ 155.74, 142.93, 135.76, 132.12 (Ar–CH), 132.07 (Ar–CH), 130.68 (Ar–CH), 130.46 (Ar–CH), 126.01 (Ar–CH), 125.45 (Ar–CH), 121.90 (alkene-CH), 103.69 (alkene-CH), 66.56 (CH2–O), 60.75 (CH2–O), 52.58 (CH2–N), 52.54 (CH2–N) ppm.
2,4,6-Tris((1-(4-(azidomethyl)benzyl)-1H-1,2,3-triazol-4-yl)methoxy)-3,5-dichloropyridine 5f. Light brown solid. Yield 90%. MP 250 °C (dec); IR (KBr)
3726, 3424, 3136, 2949, 2098, 1561. 1H NMR (500 MHz, DMSO-d6) δ 8.33 (s, 3H, tetrazole-H), 7.22–7.43 (m, 12H, Ar–H), 5.61–5.48 (m, 12H, CH2O, CH2–N), 5.22 (s, 2H, CH2N3), 4.41 (s, 4H, CH2N3) ppm. 13C-NMR (126 MHz, DMSO-d6) δ 135.76, 132.16, 132.11 (Ar–CH), 131.89, 130.60 (Ar–CH), 130.48, 129.07 (Ar–CH), 128.33 (Ar–CH), 127.80 (Ar–CH), 127.55, 125.37, 125.36 (Ar–CH), 121.87 (alkene-CH), 115.68 (alkene-CH), 67.90 (CH2–O), 60.03 (CH2–O), 52.56 (CH2–N), 52.55 (CH2–N), 38.58, 30.27 ppm.
3.6. General procedure for the synthesis of tris(1,2,3-triazoles) 7a–d under ultrasonic irradiation
To a mixture of precursors 3a,b (1 mmol), aliphatic bromides 6a,b (3 mmol), and NaN3 (3.6 mmol) in tBuOH/H2O (8 mL, 1
:
3 v/v), was added CuSO4·5H2O (5 mol%) and AscNa (15 mol%). The mixture was then sonicated at 60 °C for the appropriate time. Finally, the crude product was purified by column chromatography using CH2Cl2/MeOH (95
:
5) as eluent to obtain the pure products 7a–d.
Triethyl 2,2′,2′′-((((3,5-difluoropyridine-2,4,6-triyl)tris(oxy))tris(methylene))tris(1H-1,2,3-triazole-4,1-diyl))triacetate 7a. White solid. Yield 80%. MP 153–155 °C; IR (KBr)
3726, 3146, 2964, 2100, 1743, 1626 cm−1. 1H-NMR (500 MHz, DMSO-d6): δ 8.26 (s, 3H, tetrazole-H), 5.52 (s, 4H, CH2O), 5.47 (s, 2H, CH2–O), 5.39 (s, 6H, CH2–N), 4.14–4.19 (q, 6H, J = 7.9 Hz, CH2), 1.20 (t, 9H, J = 7.6 Hz, CH3) ppm. 13C NMR (126 MHz, DMSO-d6) δ 167.56, 144.90 (dm, 2JCF = 11.25 Hz, C2,6-py), 144.05, 143.96, 142.76, 142.12, 133.96 (d, 1JCF = 245 Hz, C3,5-py), 126.99, 126.68, 66.57, 61.93, 60.00, 50.86, 14.36 ppm. 19F NMR (470 MHz, DMSO-d6) δ −164.83 (2F, F3,5-py).
2,4,6-Tris((1-allyl-1H-1,2,3-triazol-4-yl)methoxy)-3,5-difluoropyridine 7b. Light brown liquid. Yield 83%. IR (KBr)
3726, 3375, 2926, 2139, 1657, 1625. 1H NMR (500 MHz, DMSO-d6) δ 8.21 (s, 3H, tetrazole-H), 5.95–6.09 (m, 3H, CH), 5.51 (s, 4H, CH2O), 5.44 (s, 2H, CH2–O), 5.16–5.25 (ddt, 6H, CH
), 5.01–5.05 (m, 6H, CH2–N) ppm. 13C-NMR (126 MHz, DMSO-d6) δ 144.94 (dm, 2JCF = 10 Hz, C2,6-py), 144.53, 144.02 (m), 143.18, 142.89, 142.24, 134.21 (d, 1JCF = 208.5 Hz, C3,5-py), 125.50, 125.11, 119.25, 66.64 (CH2–O), 60.01 (CH2–O), 55.20 (CH2–N), 52.18 (CH2–N) ppm. 19F NMR (470 MHz, DMSO-d6) δ −164.80 (2F, F3,5-py).
Triethyl 2,2′,2′′-((((3,5-dichloropyridine-2,4,6-triyl)tris(oxy))tris(methylene))tris(1H-1,2,3-triazole-4,1-diyl))triacetate 7c. White solid. Yield 78%. MP 168–170 °C; IR (KBr)
2923, 2855, 1638, 1420 cm−1. 1H-NMR (500 MHz, DMSO-d6): δ 8.33 (s, 3H, tetrazole-H), 5.62 (s, 4H, CH2–O), 5.45 (s, 6H, CH2–N), 5.30 (s, 2H, CH2–O), 4.14–4.24 (q, 6H, J = 7.8 Hz, CH2), 1.23 (t, 9H, J = 6.1 Hz CH3) ppm. 13C NMR (126 MHz, DMSO-d6) δ 167.69, 167.57, 160.94, 155.79, 142.66, 142.13, 127.24, 126.84, 103.69, 66.63, 61.99, 60.78, 50.90, 14.41 ppm.
2,4,6-Tris((1-allyl-1H-1,2,3-triazol-4-yl)methoxy)-3,5-difluoropyridine 7d. Light brown liquid. Yield 71%. IR (KBr)
3652, 3418, 2923, 1720, 1578. 1H NMR (500 MHz, DMSO-d6) δ 8.21–8.27 (s, 3H, tetrazole-H), 5.98–6.09 (m, 3H, CH), 5.55 (s, 4H, CH2O), 5.50 (s, 2H, CH2–O), 5.20–5.26 (ddt, 6H, CH
), 5.00–5.04 (m, 6H, CH2–N) ppm. 13C-NMR (126 MHz, DMSO-d6): δ 155.79, 142.73, 142.71, 133.13, 125.74, 125.21 (Ar–C), 124.46, 119.31, 118.94, 103.73, 66.70 (CH2–O), 60.80 (CH2–O), 52.17 (CH2–N), 52.06 (CH2–N) ppm.
3.7. Synthesis of 2,3,4,5-tetrachloro-6-(prop-2-yn-1-yloxy)pyridine 3c
In a 25 mL round-bottomed flask, a mixture of propargyl alcohol (1 mmol), K2CO3 (1.3 mmol), and DMF (2 mL) was stirred at room temperature for 30 minutes. Next, PCP (1 mmol) was added and the resulting mixture was stirred at 60 °C for 24 hours. Then, the reaction mixture was poured into 10 mL water and extracted with ethyl acetate (2 × 30 mL). The organic phase was dried over MgSO4, and the solvent evaporated. The pure product 3c was obtained in 83% yield after washing with hexane.
Brown solid. Yield 83%. IR (KBr)
3394, 3143, 2919, 2132, 1627. 1H NMR (500 MHz, DMSO-d6) δ 5.05 (d, 2H, CH2–O), 3.65 (t, 1H, acetylene-H) ppm. 13C-NMR (125 MHz, DMSO-d6) δ 155.94, 143.79, 143.54, 117.04, 79.19 (acetylene-C), 78.36 (acetylene-CH), 56.46 (CH2–O) ppm.
3.8. Synthesis of ((1,2,3-triazol-4-yl)methoxy)-3,4,5,6-tetrachloropyridine 8a–c derivatives
Precursors 3c (1 mmol) were dissolved in CH2Cl2/H2O (5 mL, 1
:
1). Aryl azides 4a–c (1.2 mmol), CuSO4·5H2O (5 mol%), and NaAsc (15 mol%) were added and the resulting mixture was sonicated at 60 °C for 8 hours. The completion of the reaction was controlled by TLC until the consumption of precursors 3c. Then, H2O (5 mL) was added to the mixture, and the precipitate was collected by filtration, washed thoroughly with H2O, and CH2Cl2, and dried under vacuum. The crude was purified by column chromatography using EtOAc/Hexane (50/20) as eluent to obtain products 8a–c.
2-((1-Benzyl-1H-1,2,3-triazol-4-yl)methoxy)-3,4,5,6-tetrachloropyridine 8a. White solid. Yield 89%. MP 135–137 °C; IR (KBr)
3416, 2924, 2853, 1618, 1497 cm−1. 1H-NMR (500 MHz, DMSO-d6) δ 8.37 (s, 1H, tetrazole-H), 7.28–7.36 (m, 5H, Ar-CH), 5.63 (s, 2H, CH2–O), 5.33 (s, 2H, CH2–N) ppm. 13C-NMR (126 MHz, DMSO-d6) δ 144.43, 143.35, 135.81, 133.21, 132.11, 131.28, 130.62, 125.31, 121.88, 59.68 (CH2–O), 52.56 (CH2–N) ppm.
2-((1-(4-Bromobenzyl)-1H-1,2,3-triazol-4-yl)methoxy)-3,4,5,6-tetrachloropyridine 8b. White crystal. Yield 78%. MP 146–147 °C; IR (KBr)
3726, 3423, 2929, 2513, 1796, 1721, 1639 cm−1. 1H-NMR (500 MHz, CDCl3) δ 7.67 (s, 1H, tetrazole-H), 7.52–7.55 (d, 2H, Ar–H), 7.18–7.20 (d, 2H, Ar–CH), 5.56 (s, 2H, CH2–N), 5.52 (s, 2H, CH2–O) ppm. 13C-NMR (125 MHz, CDCl3) δ 156.20, 144.09, 143.75, 142.97, 133.38, 132.35, 129.75, 123.95, 123.07, 122.40, 117.30, 61.43 (CH2–O), 53.56 (CH2–N) ppm.
2-((1-(4-Bromobenzyl)-1H-1,2,3-triazol-4-yl)methoxy)-3,4,5,6-tetrachloropyridine 8c. Light brown solid. Yield 86%. MP 159–162 °C; IR (KBr)
3726, 3416, 2924, 2853, 1629, 1497 cm−1. 1H-NMR (500 MHz, DMSO-d6) δ 8.36 (1H, tetrazole-H), 6.90–7.64 (m, 4H, Ar–H), 5.60–5.70 (m, 2H, CH2–O), 5.31–5.45 (m, 2H, CH2–N), 3.55 (s, 2H, CH2–N3), 5.28–5.48 (m, 2H, CH2–N) ppm. 13C-NMR (125 MHz, DMSO-d6): δ 143.57, 141.96, 136.25, 132.17, 132.04, 129.29, 129.16, 128.87, 125.87, 61.83 (CH2–O), 53.62 (CH2–N) ppm.
3.9. General procedure for SMC reactions of triazolyl bromides 5b,e with arylboronic acids 9a–c under thermal condition
A mixture of triazolyl bromides 5a,e (1 mmol), phenylboronic acid 9a (3.8 mmol), Cs2CO3 (4.5 mmol), and [Pd(PPh3)4] (0.045 mol%) in DMF/H2O (1
:
3) was stirred at 70 °C under argon atmosphere for 24 hours. The catalyst was separated by filtration and the crude product was purified by column chromatography on silica gel using CH2Cl2–MeOH (9
:
1) as eluent to obtain the pure coupling products 10a–f.
2,4,6-Tris((1-([1,1′-biphenyl]-4-ylmethyl)-1H-1,2,3-triazol-4-yl)methoxy)-3,5-difluoropyridine 10a. White solid. Yield 72%. MP 203–207 °C; IR (KBr)
3062, 2957, 2929, 1723, 1626 cm−1. 1H-NMR (500 MHz, DMSO-d6) δ 8.17 (s, 3H, tetrazole-H), 7.58–7.66 (m, 12H, Ar–H), 7.33–7.43 (m, 10H), 7.19–7.27 (m, 4H), 5.55–5.64 (m, 6H, CH2O), 5.40–5.48 (m, 4H, CH2–N), 5.25 (s, 2H, CH2–N) ppm. 13C NMR (126 MHz, DMSO-d6) δ 167.40, 144.00 (m), 143.05, 142.43, 142.36 (m), 140.50, 140.00, 135.77, 135.51, 134.04 (d, 1JCF = 247.5 Hz, C3,5-py) 132.20, 132.11 (d, 2JCF = 8.67 Hz, C4-py), 131.98, 130.65, 130.57 (Ar–C), 130.49, 129.35 (Ar–CH), 129.08 (Ar–CH), 129.03 (Ar–CH), 128.97 (Ar–CH), 128.89 (Ar–CH), 128.02 (Ar–CH), 127.84, 127.49, 127.12 (alkene-CH), 125.81, 125.42, 124.80 (alkene-CH), 67.89 (CH2–O), 60.03 (CH2–O), 53.00 (CH2–N), 52.54 (CH2–N) ppm. 19F NMR (470 MHz, DMSO-d6) δ −164.65 (2F, F3,5-py).
3,5-Difluoro-2,4,6-tris((1-(4-(naphthalen-2-yl)benzyl)-1H-1,2,3-triazol-4-yl)methoxy)pyridine 10b. White solid. Yield 70%. MP 263–265 °C; IR (KBr)
3424, 3052, 2952, 1626, 1593, 1491 cm−1. 1H-NMR (500 MHz, DMSO-d6) δ 8.37 (s, 3H, tetrazole-H), 8.29–8.30 (d, J = 6 Hz, 2H), 8.00–8.05 (m, 9H), 7.95 (d, J = 7.8 Hz, 2H), 7.52–7.66 (m, 12H, Ar–H), 7.25 (d, J = 8.1 Hz, 4H), 7.20 (d, J = 8.7 Hz, 2H), 5.57–5.58 (s, 6, CH2O), 5.45 (s, 4H, CH2–N), 5.40 (s, 2H, CH2–N) ppm. 13C NMR (126 MHz, DMSO-d6) δ 143.46 (m), 143.03, 142.40 (m), 136.74 (d, 1JCF = 245.0 Hz, C3,5-py), 135.63, 133.86, 132.77 (triazole-C), 132.12 (triazole-C), 132.10, 130.65 (Ar–C), 130.49, 128.98 (Ar–CH), 128.67 (Ar–CH), 127.95 (Ar–CH), 126.90, 126.63, 126.03 (alkene-CH), 125.82, 125.70, 125.42 (alkene-CH), 121.86, 66.62 (CH2–O), 60.01 (CH2–O), 52.57 (CH2–N), 52.54 (CH2–N) ppm. 19F NMR (470 MHz, DMSO-d6) δ −164.65 (2F, F3,5-py).
4′-((4-(((2,6-Bis((1-(4-bromobenzyl)-1H-1,2,3-triazol-4-yl)methoxy)-3,5-difluoropyridin-4-yl)oxy)methyl)-1H-1,2,3-triazol-1-yl)methyl)-[1,1′-biphenyl]-4-carbaldehyde 10c. White solid. Yield 62%. MP 285–288 °C; IR (KBr)
3725, 3424, 3136, 3061, 2958, 28.60, 1724. 1H-NMR (500 MHz, DMSO-d6) δ 10.07 (s, 1H, CHO), 8.21–8.34 (s, 3H, tetrazole-H), 8.03 (d, 2H, J = 8.4 Hz, Ar), 7.99 (d, 2H, J = 8.2 Hz), 7.84–7.87 (d, J = 8.2 Hz, 2H), 7.52–7.55 (m, 6H, Ar–H), 7.25–7.28 (m, 4H), 7.20 (d, 2H, J = 8.4 Hz), 5.58 (s, 6, CH2O), 5.45 (s, 4H, CH2–N), 5.40 (s, 2H, CH2–N). 13C-NMR (126 MHz, DMSO-d6) δ 193.14, 144.88 (dm, 2JCF = 10.01 Hz, C2,6-py), 144.06 (m), 143.96, 135.74, 134 (d, 1JCF = 242.5 Hz, C3,5-py), 132.18, 132.10, 131.96 (triazole-C), 130.65 (triazole-C), 130.60 (Ar–C), 130.52, 129.10 (Ar–CH), 128.33 (Ar–CH), 127.96 (Ar-CH), 127.80, 127.57, 125.43 (alkene-CH), 121.90, 115.67 (alkene-CH), 67.90 (CH2–O), 60.01 (CH2–O), 58.91 (CH2–N), 52.59 (CH2–N) ppm. 19F NMR (470 MHz, DMSO-d6) δ −164.61 (2F, F3,5-py).
2,4,6-Tris((1-([1,1′-biphenyl]-4-ylmethyl)-1H-1,2,3-triazol-4-yl)methoxy)-3,5-dichloropyridine 10d. White solid. Yield 73%. MP 232–234 °C; IR (KBr)
3060, 2952, 1578, 1561, 1489 cm−1. 1H-NMR (500 MHz, DMSO-d6) δ 8.24 (s, 3H, tetrazole-H), 7.60–7.66 (m, 5H, Ar), 7.53–7.56 (m, 5H, Ar), 7.42–7.47 (m, 5H, Ar–H), 7.334–7.40 (m, 6H, Ar), 7.24–7.29 (m, 4H, Ar), 7.20–7.23 (d, 2H, J = 8.2 Hz), 5.55–5.63 (m, 6, CH2O), 5.45–5.54 (m, 4H, CH2–N), 5.37–5.44 (m, 2H, CH2–N) ppm. 13C NMR (126 MHz, DMSO-d6) δ 146.05, 142.95, 135.88, 135.77, 134.94, 132.13, 132.08, 130.68 (triazole-C), 130.63 (triazole-C), 130.47 (Ar–C), 129.35, 129.06 (Ar–CH), 128.81 (Ar–CH), 128.02 (Ar–CH), 127.84 (Ar–CH), 127.50 (Ar–CH), 127.12 (Ar–CH), 126.26, 125.99, 125.42 (alkene-CH), 125.12, 121.90, 121.83 (alkene-CH), 66.59 (CH2–O), 60.79 (CH2–O), 52.58 (CH2–N), 52.49 (CH2–N) ppm.
3,5-Dichloro-2,4,6-tris((1-(4-(naphthalen-2-yl)benzyl)-1H-1,2,3-triazol-4-yl)methoxy)pyridine 10e. White solid. Yield 69%. MP 274–276 °C; IR (KBr)
3449, 3052, 1580, 1490, 1412 cm−1. 1H-NMR (500 MHz, DMSO-d6) δ 8.37 (s, 3H, tetrazole-H), 8.29 (d, J = 6 Hz, 3H), 8.00–8.05 (m, 8H), 7.95 (d, J = 7.8 Hz, 4H), 7.53–7.56 (m, 12H, Ar–H), 7.26 (d, J = 8.1 Hz, 4H), 7.20 (d, J = 8.7 Hz,2H), 5.59 (s, 6, CH2O), 5.51 (s, 4H, CH2–N), 5.22 (s, 2H, CH2–N) ppm. 13C NMR (126 MHz, DMSO-d6) δ 143.91, 143.21, 141.99, 137.72, 135.76, 137.73, 133.86,132.77, 132.74, 132.13 (triazole-C), 132.08 (triazole-C), 130.68 (Ar–C),128.99, 128.67, 127.96 (Ar–CH), 126.91 (Ar–CH), 126.64 (Ar–CH), 126.30, 126.04, 125.71 (alkene-CH), 125.44, 121.90 (alkene-CH), 66.97 (CH2–O), 60.74 (CH2–O), 52.59 (CH2–N), 52.44 (CH2–N) ppm.
4′-((4-(((2,6-Bis((1-(4-bromobenzyl)-1H-1,2,3-triazol-4-yl)methoxy)-3,5-dichloropyridin-4-yl)oxy)methyl)-1H-1,2,3-triazol-1-yl)methyl)-[1,1′-biphenyl]-4-carbaldehyde 10f. White solid. Yield 56%. MP 280 °C (dec); IR (KBr)
3726, 3448, 3138, 2951, 2841, 1694. 1H NMR (500 MHz, DMSO-d6). δ 10.07 (s, 3H, CHO), 8.30 (s, 3H, tetrazole-H), 8.03 (d, 2H, J = 8.4 Hz), 7.99 (d, 2H, J = 8.2 Hz), 7.49–7.57 (m, 6H), 7.24–7.31 (m, 4H), 7.21 (d, 2H, J = 8.5 Hz), 5.56–5.62 (m, 6, CH2O), 5.480–5.54 (m, 4H, CH2–N), 5.24 (s, 2H, CH2–N) ppm. 13C NMR (126 MHz, DMSO-d6): δ 193.19, 155.74, 144.83, 142.93, 142.35, 136.25, 135.78, 132.13, 132.08, 130.69 (triazole-C), 130.62 (Ar–C), 130.47, 128.35 (Ar-CH), 127.81 (Ar–CH), 125.47, 121.92, 103.69, 67.56 (CH2–O), 60.76 (CH2–O), 52.58 (CH2–N), 52.51 (CH2–N) ppm.
4. Conclusions
In summary, perhalopyridine-based alkyne precursors 3a and 3b were prepared through the SNAr reaction of PFP and PCP with excess amounts of propargyl alcohol. We have accordingly developed the click reaction of the derived alkynes with aryl azides 4a–c under ultrasonic irradiation as an effective method for synthesizing poly(1,2,3-triazolyl)-substituted perhalopyridines 5a–f. However, aliphatic 1,2,3-triazole analogues 7a–d were formed via the sonication reaction of pyridyl cores 3, alkyl bromides 6a,b, and NaN3 under one-pot conditions. Utilizing 2,3,4,5-tetrachloro-6-(prop-2-yn-1-yloxy)pyridine 3c, drived from the regioselective SNAr reaction of PCP with propargyl alcohol, we also succeeded to furnish several ((1,2,3-triazol-4-yl)methoxy)-3,4,5,6-tetrachloropyridines 8a–c under the reaction conditions. Finally, biaryl-embedded perfluoropyridine-based poly(1,2,3-triazoles) 10a–f were produced in good yields via the SMC reaction of tris-triazole 5b,e with arylboronic acids 9a–c under Pd(PPh3)4 catalysis.
Author contributions
Fereshteh Khorasani: conceptualization, investigation, methodology, data analysis, writing – review & editing; Reza Ranjbar-Karimi and Kazem Mohammadiannejad: investigation, writing – review & editing; Reza Ranjbar-Karimi: supervision.
Conflicts of interest
There are no conflicts to declare.
Acknowledgements
The authors are grateful to the research council of the Vali-e-Asr University of Rafsanjan for financial support of this work.
References
-
(a) P. Kirsch, J. Fluorine Chem., 2015, 177, 29–36 CrossRef CAS;
(b) C. P. Umesh, A. T. Marcelis and H. Zuilhof, Liq. Cryst., 2014, 41, 1911–1922 CrossRef CAS;
(c) M. Hird, Chem. Soc. Rev., 2007, 36, 2070–2095 RSC.
-
(a) T. Hiyama, Organofluorine Compounds: Chemistry and Applications, Springer-Verlag, Berlin, 2000 CrossRef;
(b) Y. Ogawa, E. Tokunaga, O. Kobayashi, K. Hirai and N. Shibata, iScience, 2020, 23, 101467 CrossRef CAS PubMed;
(c) P. Jeschke, ChemBioChem, 2004, 5, 570–589 CrossRef CAS PubMed.
-
(a) A. C. Stuart, J. R. Tumbleston, H. Zhou, W. Li, S. Liu, H. Ade and W. You, J. Am. Chem. Soc., 2013, 135, 1806–1815 CrossRef CAS PubMed;
(b) B. Qiu, S. Chen, L. Xue, C. Sun, X. Li, Z.-G. Zhang, C. Yang and Y. Li, Front. Chem., 2018, 6, 413, DOI:10.3389/fchem.2018.00413.
-
(a) E. Hequet, C. Henoumont, R. N. Muller and S. Laurent, Future Med. Chem., 2019, 11, 1157–1175 CrossRef CAS PubMed;
(b) D. Cosco, E. Fattal, M. Fresta and N. Tsapis, J. Fluorine Chem., 2015, 171, 18–26 CrossRef CAS.
-
(a) V. P. Reddy, Organofluorine Compounds in Biology and Medicine, Elsevier, 2015 Search PubMed;
(b) K. R. Scroggie, M. V. Perkins and J. M. Chalker, Front. Chem., 2021, 9, 687678, DOI:10.3389/fchem.2021.687678.
- L. J. Blus, Organochlorine pesticides, in Handbook of Ecotoxicology, 2000 Search PubMed.
- Y. Zhou, H. J. Wu, Y. H. Zhang, H. Y. Sun, T. M. Wong and G. R. Li, Toxicol., 2011, 290, 295–304 CrossRef PubMed.
- I. I. Salakhov, V. G. Kozlov, L. B. Sosnovskaya, I. E. Nifant'ev and P. V. Ivchenko, Polym. Sci., Ser. B, 2023, 65, 79–102 CrossRef CAS.
- F. Zhu, W. Ruan, L. Liu, R. Lai, F. Zeng and G. Ouyang, Microchem. J., 2013, 110, 280–284 CrossRef.
- R. Jayaraj, P. Megha and P. Sreedev, Interdiscip. Toxicol., 2016, 9, 90–100 CrossRef CAS PubMed.
-
(a) Z. Vari, R. Ranjbar-Karimi and K. Mohammadiannejad, New J. Chem., 2023, 47, 10645–10658 RSC;
(b) R. Ranjbar-Karimi, T. Davodian and H. Mehrabi, J. Heterocycl. Chem., 2017, 55, 475–480 CrossRef;
(c) M. B. Houck, T. J. Fuhrer, C. R. Phelps, L. C. Brown and S. T. Iacono, Macromolecules, 2021, 54, 5586–5594 CrossRef CAS;
(d) R. Ranjbar-Karimi, A. Karbakhsh-Ravari and A. Poorfreidoni, J. Iran. Chem. Soc., 2017, 14, 2397–2405 CrossRef CAS;
(e) A. Schmidt and T. Mordhorst, Synthesis, 2005, 781–786 CrossRef CAS.
-
(a) S. Anga, S. Chandra, P. Sarkar, S. Das, D. Mandal, A. Kundu, H. Rawat, C. Schulzke, B. Sarkar, S. K. Pati, V. Chandrasekhar and A. Jana, Eur. J. Org Chem., 2020, 7445–7449 CrossRef CAS;
(b) G. Podolan, P. Jungk, D. Lentz, R. Zimmer and H. U. Reissig, Adv. Synth. Catal., 2015, 357, 3215–3228 CrossRef CAS;
(c) T. Moradi, R. Ranjbar-Karimi, A. Poorfreidoni and H. Mehrabi, Polycyclic Aromat. Compd., 2022, 42, 1610–1619 CrossRef CAS;
(d) R. Hosseini, R. Ranjbar-Karimi and K. Mohammadiannejad, J. Fluorine Chem., 2020, 233, 109507 CrossRef CAS;
(e) G. Sandford, Halogenated Heterocycles, 2011, pp. 1–31 Search PubMed.
-
(a) S.-C. Lee, C.-M. Hsu, H. Kao, L.-Y. Li, Z.-N. Tsai, Y.-T. Tsao, Y.-L. Hsu, S. Miñoza, C.-C. Chiu and H.-H. Liao, ChemRxiv, 2021, preprint, DOI:10.26434/chemrxiv-2021-fdd2c;
(b) A. Nova, R. Mas-Balleste and A. Lledos, Organometallics, 2012, 31, 1245–1256 CrossRef CAS;
(c) H.-H. Liao, S.-C. Lee, H. Kao, Y.-L. Hsu, C.-M. Hsu, Y.-T. Tsao, S. Miñoza, L.-Y. Li, Z.-N. Tsai, K.-C. Chang, C.-K. Cheng, C.-L. Chan, Y.-S. Chien and C.-C. Chiu, Cell Rep. Phys. Sci., 2022, 3, 101010 CrossRef CAS;
(d) S. Gahlot, A. Gradone, M. Roy, M. Giorgi, S. Conti, P. Ceroni and M. Gingras, Chem. Eur J., 2022, 28, e202200797 CrossRef CAS PubMed.
-
(a) D. Mandal, S. Chandra, N. I. Neuman, A. Mahata, A. Sarkar, A. Kundu, S. Anga, H. Rawat, C. Schulzke, K. R. Mote, B. Sarkar, V. Chandrasekhar and A. Jana, Chem. Eur J., 2020, 26, 5951–5955 CrossRef CAS PubMed;
(b) R. D. Chambers, J. Hutchinson and W. K. R. Musgrave, J. Chem. Soc., 1964, 722, 3736 RSC;
(c) R. D. Chambers, J. A. Jackson, W. K. R. Musgrave and R. A. Storey, J. Chem. Soc., 1968, 2221–2227 CAS.
-
(a) R. Hosseini, R. Ranjbar-Karimi and K. Mohammadiannejad, J. Fluorine Chem., 2020, 233, 109507 CrossRef CAS;
(b) A. Schmidt and T. Mordhorst, Z. Naturforsch. B, 2006, 61, 396–405 CrossRef CAS;
(c) A. M. Sipyagin, S. V. Pal'tsun, I. A. Pomytkin and N. N. Aleinikov, Chem. Heterocycl. Compd., 1994, 30, 56–59 CrossRef;
(d) R. D. Chambers, P. R. Hoskin, G. Sandford, D. S. Yufit and J. A. Howard, J. Chem. Soc., Perkin Trans. 1, 2001, 2788–2795 RSC.
- C. M. Friesen, N. J. Weeks and S. T. Iacono, Molbank, 2024, 22, M1777 CrossRef.
- C. A. Corley, K. Kobra, A. J. Peloquin, K. Salmon, L. Gumireddy, T. A. Knoerzer and S. T. Iacono, J. Fluorine Chem., 2019, 228, 109409 CrossRef.
- K. A. Stewart, D. Shuster, M. Leising, I. Coolidge, E. Lee, C. Stevens and S. T. Iacono, Macromol., 2021, 54, 4871–4879 CrossRef CAS.
- L. M. Moore, K. T. Greeson, K. A. Stewart, D. A. Kure, C. A. Corley, A. R. Jennings, S. T. Iacono and K. B. Ghiassi, Macromol. Chem. Phys., 2020, 221, 2000100 CrossRef CAS.
- L. N. Beardmore, S. L. Cobb and W. D. Brittain, Org. Biomol. Chem., 2022, 20, 8059–8064 RSC.
- L. Zheng, Y. E. Qian, Y. Z. Hu, J. A. Xiao, Z. P. Ye, K. Chen and H. Yang, Org. Lett., 2021, 23, 1643–1647 CrossRef CAS PubMed.
- A. Schmidt, J. C. Namyslo and T. Mordhorst, Tetrahedron, 2006, 62, 6893–6898 CrossRef CAS.
- Z. J. Wang, Y. Zheng, K. Tang, J. P. Guan, Z. P. Ye, J. T. He and H. Yang, J. Org. Chem., 2022, 87, 4732–4741 CrossRef CAS PubMed.
-
(a) P. Ehlers, S. Reimann, S. Erfle, A. Villinger and P. Langer, Synlett, 2010, 1528–1532 CAS;
(b) S. Reimann, P. Ehlers, A. Petrosyan, S. Kohse, A. Spannenberg, A. E. Surkus and P. Langer, Adv. Synth. Catal., 2014, 356, 1987–2008 CrossRef CAS.
- J. Gao, Z. P. Ye, Y. F. Liu, X. C. He, J. P. Guan, F. Liu and H. Yang, Org. Lett., 2022, 24, 4640–4644 CrossRef CAS PubMed.
-
(a) D. Brunel and F. Dumur, New J. Chem., 2020, 44, 3546–3561 RSC;
(b) T. Horsten, F. de Jong, D. Theunissen, M. Van der Auweraer and W. Dehaen, J. Org. Chem., 2021, 86, 13774–13782 CrossRef CAS PubMed;
(c) T. Duan, K. Fan, Y. Fu, C. Zhong, X. Chen, T. Peng and J. Qin, Dyes Pigm., 2012, 94, 28–33 CrossRef CAS.
- W. Q. Fan and A. R. Katritzky, in Comprehensive Heterocyclic Chemistry II, ed. A. R. Katritzky, C. W. Rees and E. F. Scriven, ElsevierScience, Oxford, 1996, vol. 4, p. 1 Search PubMed.
-
(a) V. P. Krivopalov and O. P. Shkurko, Russ. Chem. Rev., 2005, 74, 339–379 CrossRef CAS;
(b) C. A. Franco, T. I. da Silva, M. G. Dias, B. W. Ferreira, B. L. de Sousa, G. M. Bousada and E. V. Vieira Varejão, J. Agric. Food Chem., 2022, 70, 2806–2816 CrossRef CAS PubMed.
-
(a) P. M. Miladinova and T. N. Konstantinova, J. Chem. Technol. Metall., 2015, 50, 229–239 Search PubMed;
(b) C. W. Tornoe, C. Christensen and M. Meldal, J. Org. Chem., 2002, 67, 3057–3064 CrossRef CAS PubMed.
-
(a) D. Lengerli, K. Ibis, Y. Nural and E. Banoglu, Expert Opin. Drug Discovery, 2022, 17, 1209–1236 CrossRef CAS PubMed;
(b) S. G. Agalave, S. R. Maujan and V. S. Pore, Chem.–Asian J., 2011, 6, 2696–2718 CrossRef CAS PubMed;
(c) K. Suryanarayana, S. Maddila, K. Nagaraju and S. B. Jonnalagadda, J. Mol. Struct., 2022, 1250, 131713 CrossRef CAS;
(d) U. K. Patel, P. Tiwari, R. Tilak, G. Joshi, R. Kumar and A. Agarwal, RSC Adv., 2024, 14, 17051–17070 RSC;
(e) N. Ramu, T. M. Krishna, R. Kapavarapu and S. Narsimha, RSC Adv., 2024, 14, 8921–8931 RSC;
(f) B. F. Abdel-Wahab, B. M. Kariuki, H. A. Mohamed, M. S. Bekheit, H. M. Awad and G. A. El-Hiti, J. Mol. Struct., 2023, 1294, 136528 CrossRef CAS;
(g) Y. Nural, S. Ozdemir, M. S. Yalcin, B. Demir, H. Atabey, Z. Seferoglu and A. Ece, Bioorg. Med. Chem. Lett., 2022, 55, 128453 CrossRef CAS PubMed;
(h) Q. Guan, S. Xing, L. Wang, J. Zhu, C. Guo, C. Xu and H. Sun, J. Med. Chem., 2024, 67, 7788–7824, DOI:10.1021/acs.jmedchem.4c00652.
-
(a) X. L. Wang, K. Wan and C. H. Zhou, Eur. J. Med. Chem., 2010, 45, 4631–4639 CrossRef CAS PubMed;
(b) M. J. Genin, D. A. Allwine, D. J. Anderson, M. R. Barbachyn, D. E. Emmert, S. A. Garmon, D. R. Graber, K. C. Grega, J. B. Hester, D. K. Hutchinson, J. Morris, R. J. Reischer, C. W. Ford, G. E. Zurenko, J. C. Hamel, R. D. Schaadt, D. Stapert and B. H. Yagi, J. Med. Chem., 2000, 43, 953 CrossRef CAS PubMed;
(c) S. K. Gandham, A. A. Kudale, T. R. Allaka, K. Chepuri and A. Jha, J. Mol. Struct., 2024, 1295, 136714 CrossRef CAS;
(d) B. F. Abdel-Wahab, E. Abdel-Latif, H. A. Mohamed and G. E. A. Awa, Eur. J. Med. Chem., 2012, 52, 263–268 CrossRef CAS PubMed.
-
(a) I. Seck, I. Ciss, A. Diédhiou, M. Baldé, S. Ka, L. A. Ba and M. Seck, Med. Chem. Res., 2023, 32, 158–164 CrossRef CAS;
(b) R. M. C. Souza, L. M. L. M. Pimentel, L. K. M. Ferreira, V. R. A. Pereira, A. C. D. S. Santos, W. M. Dantas and R. N. De Oliveira, Eur. J. Med. Chem., 2023, 255, 115400 CrossRef PubMed.
-
(a) Y. Sun, D. Feng, Z. Zhou, T. Zhang, E. De Clercq, C. Pannecouque and X. Liu, Bioorg. Med. Chem., 2023, 96, 117484 CrossRef CAS PubMed;
(b) R. Alvarez, S. Velazquez, F. San, S. Aquaro, C. De, C. F. Perno, A. Karlesson, J. Balzarini and M. J. Camarasa, J. Med. Chem., 1994, 37, 4185 CrossRef CAS PubMed;
(c) C. S. Takeuchi, B. G. Kim, C. M. Blazey, S. Ma, H. W. B. Johnson, N. K. Anand, A. Arcalas, T. G. Baik, C. A. Buhr, J. Cannoy, S. Epshteyn, A. Joshi, K. Lara, M. S. Lee, L. Wang, J. W. Leahy, J. M. Nuss, N. Aay, R. Aoyama, P. Foster, J. Lee, I. Lehoux, N. Munagala, A. Plonowski, S. Rajan, J. Woolfrey, K. Yamaguchi, P. Lamb and N. Miller, J. Med. Chem., 2013, 56, 2218–2234 CrossRef CAS PubMed;
(d) L. S. Feng, M. J. Zheng, F. Zhao and D. Liu, Arch. Pharm., 2021, 354, 2000163 CrossRef CAS PubMed.
- K. B. Gangurde, R. A. More, V. A. Adole and D. S. Ghotekar, J. Mol. Struct., 2024, 1299, 136760 CrossRef CAS.
- D. J. Leaver, R. M. Dawson, J. M. White, A. Polyzos and A. B. Hughes, Org. Biomol. Chem., 2011, 9, 8465–8474 RSC.
-
(a) E. Irrou, Y. A. Elmachkouri, S. El Haddad, Y. Riadi, A. Oubella, A. Auhmani and M. L. Taha, J. Mol. Struct., 2024, 1308, 138025 CrossRef CAS;
(b) W. T. De Paula, N. Glanzmann, I. K. Da Costa Nunes, L. P. Bento, H. Marcelo Gualberto Pereira, E. M. De Souza-Fagundes and A. D. Da Silva, ChemistrySelect, 2024, 9, e202303321 CrossRef CAS;
(c) Y. Guo, J. Hou, H. Wu, Y. Chen, G. Liu, D. Wang and T. Wang, J. Mol. Struct., 2024, 1298, 137042 CrossRef CAS;
(d) İ. Şahin, M. Çeşme, N. Yüce and F. Tümer, J. Biomol. Struct. Dyn., 2023, 41, 1988–2001 CrossRef PubMed;
(e) W. T. De Paula, N. Glanzmann, I. K. Da Costa Nunes, L. P. Bento, H. Marcelo Gualberto Pereira, E. M. De Souza-Fagundes and A. D. Da Silva, ChemistrySelect, 2024, 9, e202303321 CrossRef CAS;
(f) Y. Guo, J. Hou, H. Wu, Y. Chen, G. Liu, D. Wang and T. Wang, J. Mol. Struct., 2024, 1298, 137042 CrossRef CAS.
- V. Banoji, K. K. Angajala, S. Vianala, S. Manne, K. Ravulapelly and J. Vannada, Results Chem., 2024, 7, 101294 CrossRef CAS.
-
(a) M. S. Yadav, S. Rajkhowa, S. K. Singh, M. K. Jaiswal and V. K. Tiwari, ChemistrySelect, 2024, 9, e202400776 CrossRef CAS;
(b) J. F. Xiong, S. H. Luo, J. P. Huo, J. Y. Liu, S. X. Chen and Z. Y. Wang, J. Org. Chem., 2014, 79, 8366–8373 CrossRef CAS PubMed;
(c) J. F. Xiong, J. X. Li, G. Z. Mo, J. P. Huo, J. Y. Liu, X. Y. Chen and Z. Y. Wang, J. Org. Chem., 2014, 79, 11619–11630 CrossRef CAS PubMed;
(d) B. Ghanti, R. Kamble, S. Roy and S. Banerjee, J. Polym. Sci., 2023, 61, 1792–1806 CrossRef CAS;
(e) W. D. Castro-Godoy, A. A. Heredia, L. C. Schmidt and J. E. Arguello, RSC Adv., 2017, 7, 33967–33973 RSC;
(f) A. K. Feldman, B. Colasson and V. V. Fokin, Org. Lett., 2004, 6, 3897–3899 CrossRef CAS PubMed.
-
(a) Y. Tan, J. Li, J. Huo, F. Xue and Z. Wang, Synth. Commun., 2014, 44, 2974–2987 CrossRef CAS;
(b) H. Jingpei, W. Xinping, M. Guangzhen, P. Pai, Z. Mingli, C. Renhong and W. Zhaoyang, Chin. J. Org. Chem., 2014, 34, 92–106 CrossRef;
(c) Y. Shi, X. Cao and H. Gao, Nanoscale, 2016, 8, 4864–4881 RSC;
(d) X. Hou, a C. Ke and J. Fraser Stoddart, Chem. Soc. Rev., 2016, 45, 3766–3780 RSC;
(e) J. Huo, H. Hu, M. Zhang, X. Hu, M. Chen, D. Chen, J. Liu, G. Xiao, Y. Wang and Z. Wen, RSC Adv., 2017, 7, 2281–2287 RSC;
(f) Y. Nural, S. Ozdemir, M. S. Yalcin, B. Demir, H. Atabey, A. Ece and Z. Seferoglu, ChemistrySelect, 2021, 6, 6994–7001 CrossRef CAS;
(g) Y. Nural, I. Acar, D. Yetkin, C. Efeoglu, Z. Seferoğlu and F. Ayaz, Bioorg. Med. Chem. Lett., 2022, 69, 128800, DOI:10.1016/j.bmcl.2022.128800;
(h) T. R. Chan, R. Hilgraf, K. B. Sharpless and V. V. Fokin, Org. Lett., 2004, 6, 2853–282855 CrossRef CAS PubMed.
-
(a) Y. Shi and C.-H. Zhou, Bioorg. Med. Chem. Lett., 2011, 21, 956–960 CrossRef CAS PubMed;
(b) V. Haridas, S. Sahu and P. Venugopalan, Tetrahedron, 2011, 67, 727–733 CrossRef CAS;
(c) S. M. Saupe and T. Steinmetzer, J. Med. Chem., 2012, 55, 1171–1180 CrossRef CAS PubMed;
(d) M. Kwon, Y. Jang, S. Yoon, D. Yang and H. B. Jeon, Tetrahedron Lett., 2012, 53, 1606–1609 CrossRef CAS.
- J.-F. Xiong, J.-X. Li, G.-Z. Mo, J. Huo, J.-Y. Liu, X.-Y. Chen and Z.-Y. Wang, J. Org. Chem., 2014, 79, 11619–11630 CrossRef CAS PubMed.
- J.-F. Xiong, S.-H. Luo, J.-P. Huo, J.-Y. Liu, S.-X. Chen and Z.-Y. Wang, J. Org. Chem., 2014, 79(17), 8366–8373 CrossRef CAS PubMed.
-
(a) C. Ju, C. Meng, J. Ma, X. Zhang and S. Ding, Chem. Commun., 2020, 56, 3955–3958 RSC;
(b) E. Zhao, H. Li, J. Ling, H. Wu, J. Wang, S. Zhang, J. W. Y. Lam, J. Z. Sun, A. Qin and B. Z. Tang, Polym. Chem., 2014, 5, 2301–2308 RSC;
(c) L. Ye, L. Wan and F. Huang, New J. Chem., 2017, 41, 4424–4430 RSC.
-
(a) F. Rezazadeh-Jabalbarezi, R. Ranjbar-Karimi, K. Mohammadiannejad and F. Atabaki, J. Iran. Chem. Soc., 2023, 20, 2917–2922 Search PubMed;
(b) Z. Vari, R. Ranjbar-Karimi and K. Mohammadiannejad, J. Mol. Struct., 2024, 1302, 137360 CrossRef CAS.
-
(a) J. Xu and Q. Song, Org. Chem. Front., 2017, 4, 938 RSC;
(b) S. Jana, S. Adhikari, M. R. Cox and S. Roy, Chem. Commun., 2020, 56, 1871–1874 RSC.
-
(a) S. A. Baechler, M. Fehr, M. Habermeyer, A. Hofmann, K. H. Merz, H. H. Fiebig, D. Marko and G. Eisenbrand, Bioorg. Med. Chem., 2013, 21, 814–823 CrossRef CAS PubMed;
(b) T. A. Mansoor, P. M. Borralhoa, X. Luo, S. Mulhovo, C. M. P. Rodrigues and M. J. U. Ferreiraa, Phytomedicine, 2013, 20, 923–929 CrossRef CAS PubMed;
(c) J. Vrba, P. Dolezel, J. Vicar, M. Modriansky and J. Ulrichova, Toxicol. in Vitro, 2008, 22, 1008–1017 Search PubMed;
(d) H. Aldemir, R. Richarz and T. A. Gulder, Angew. Chem., Int. Ed. Engl., 2014, 53, 8286–8293 Search PubMed.
-
(a) J. S. Nair, T. Sheikh, A. L. Ho and G. K. Schwartz, Anticancer Res., 2013, 33, 1307–1316 CAS;
(b) L. J. Farmer, M. W. Ledeboer, T. Hoock, M. J. Arnost and R. S. Bethiel, J. Med. Chem., 2015, 58, 7195–7216 CrossRef CAS PubMed;
(c) H. Jamali, H. A. Khan, C. C. Tjin and J. A. Ellman, ACS Med. Chem. Lett., 2016, 7, 847–851 CrossRef CAS PubMed;
(d) M. R. Witten, L. Wissler, M. Snow, S. Geschwindner, J. A. Read, N. J. Brandon, A. C. Nairn, P. J. Lombroso, H. Käck and J. A. Ellman, J. Med. Chem., 2017, 60, 9299–9319 CrossRef CAS PubMed;
(e) F. F. Fleming, L. Yao, P. C. Ravikumar, L. Funk and B. C. Shook, J. Med. Chem., 2010, 53, 7902–7917 CrossRef CAS PubMed.
- D. Shen, Y. Xu and S. L. Shi, J. Am. Chem. Soc., 2019, 141, 14938–14945 CrossRef CAS PubMed.
Footnote |
† Electronic supplementary information (ESI) available: General experimental procedures and spectral data for all compounds. CCDC 2375380 and 2375381. For ESI and crystallographic data in CIF or other electronic format see DOI: https://doi.org/10.1039/d4ra05861e |
|
This journal is © The Royal Society of Chemistry 2024 |
Click here to see how this site uses Cookies. View our privacy policy here.