DOI:
10.1039/D4RA07165D
(Review Article)
RSC Adv., 2024,
14, 36713-36732
Novel interfaces for internet of wearable electrochemical sensors
Received
5th October 2024
, Accepted 21st October 2024
First published on 18th November 2024
Abstract
The integration of wearable devices, the Internet of Things (IoT), and advanced sensing platforms implies a significant paradigm shift in technological innovations and human interactions. The IoT technology allows continuous monitoring in real time. Thus, Internet of Wearables has made remarkable strides, especially in the field of medical monitoring. IoT-enabled wearable systems assist in early disease detection that facilitates personalized interventions and proactive healthcare management, thereby empowering individuals to take charge of their wellbeing. Until now, physical sensors have been successfully integrated into wearable devices for physical activity monitoring. However, obtaining biochemical information poses challenges in the contexts of fabrication compatibility and shorter operation lifetimes. IoT-based electrochemical wearable sensors allow real-time acquisition of data and interpretation of biomolecular information corresponding to biomarkers, viruses, bacteria and metabolites, extending the diagnostic capabilities beyond physical activity tracking. Thus, critical heath parameters such as glucose levels, blood pressure and cardiac rhythm may be monitored by these devices regardless of location and time. This work presents versatile electrochemical sensing devices across different disciplines, including but not limited to sports, safety and wellbeing by using IoT. It also discusses the detection principles for biomarkers and biofluid monitoring, and their integration into devices and advancements in sensing interfaces.
1. Introduction
The convergence of wearable devices, the Internet of Wearable Things and advanced sensing platforms heralds a transformative era in technology and human interactions. Wearable sensing devices have progressed in recent years and have demonstrated their potential for integrated systems that span various domains. These encompass future communications, wearable medical sensors, epidermal skin technology, smart glasses, health monitoring, microchip technology and the Internet of Things (IoT) technology. At the heart of this convergence lies the fusion of sensing devices with advanced materials. Specifically, wirelessly connected electrochemical sensors are seamlessly integrated into wearable devices, enabling robust real-time data collection and analysis of biomarkers, such as electrolytes, metabolites (such as potassium ions), proteins, hormones, as well as viruses and bacteria. Biomarkers exist in body fluids and tissues as well as in tears and sweat. They contain valuable information for diagnostics and offer insights beyond mere physical activity tracking. Biomarkers are detected by electrochemical sensors integrated with flexible substrates in the form of lenses, smart fabrics, skin patches and accessories. Hence, wearable electrochemical sensors, at the forefront of technological innovation, are not only reshaping the landscape of biomedicine, security and wellness as sensing platforms but are also poised to play a pivotal role in the emerging field of the Internet of Wearable Electrochemical Sensors connected by a web of the Internet of Things, termed IoT.1,2 This IoT-based technology holds immense promise in impacting many disciplines with applications ranging from health and fitness, through environmental monitoring, safety and security, to entertainment and augmented social interactions.
Recent innovations in wearable electrochemical sensors became possible via a synergy of several key advancements, including proliferation of wireless connectivity, development in materials science and engineering, advancement in energy harvesting technologies and the miniaturization of sensor electronics.3 Development in materials and nanoscience technology has facilitated the construction of flexible electrodes and substrates for circuit printing and signal recognition via modern microfabrication techniques.4,5 Advancements in the field of IoT have enabled the unified integration of sensing, processing and feedback units, to furnish real-time, continuous monitoring and data analysis (Fig. 1). The Internet of Wearables (IoW) signifies a paradigm shift in the use of networking technology, and has significant implications in improving the quality of life.3,12 Thus, within the realm of connected intelligence, a significant breakthrough has emerged as a category of incredibly efficient smart wearable devices known as IoW. These devices are non-invasive, inexpensive, portable, easy to use and capable of enabling us to counter many real-life challenges.
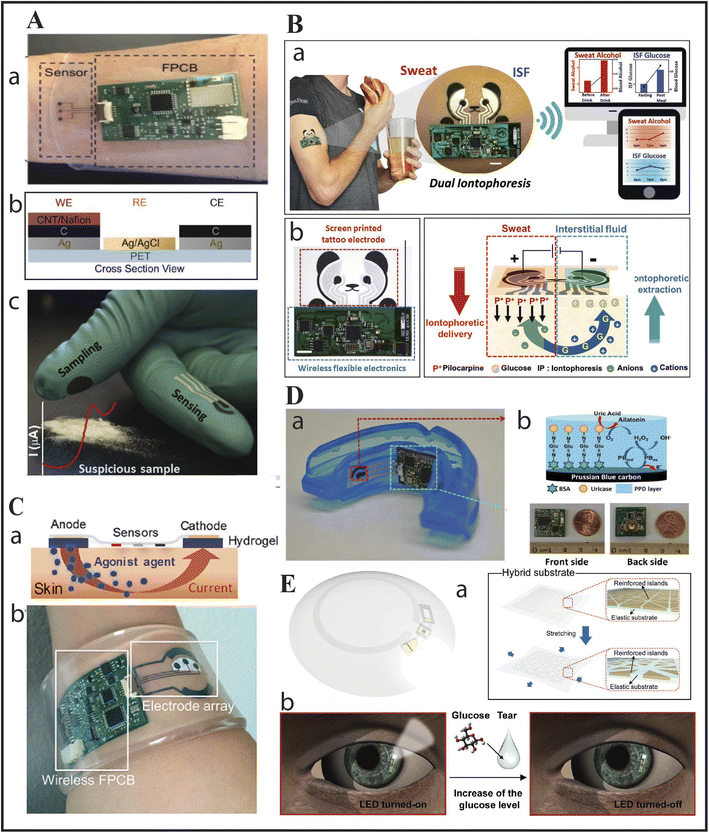 |
| Fig. 1 Body wearable devices: (A) voltammetric sensors: (a) as sweat patch regulating the drug methylxanthine; (b) schematic of caffeine sensing band; and (c) fentanyl detecting gloves with square-wave voltammetry. Reproduced from ref. 6 with permission from Wiley-VCH, copyright 2018 & reproduced from ref. 7 with permission from Elsevier, copyright 2019. (B) Another wearable dual iontophoretic biosensor based on a tattoo, to simultaneously detect glucose and sweat: (a) dual iontophoretic biosensor for ISF glucose and sweat alcohol detection on a human subject with data being transmitted wirelessly to a mobile device; (b) screen printed electrode with wireless circuit on which the iontophoretic operation takes place. Reproduced from ref. 8 with permission from Wiley-VCH, copyright 2018. (C) Illustration of drug delivery via the iontophoretic technique: (a) and (b) showcase an integrated wristband for the detection of sweat via iontophoretic technique (sensors based on detecting cystic fibrosis electrochemically). Reproduced from ref. 9 with permission from the National Academy of Sciences, copyright 2017. (D) (a) Biosensor as a mouthguard with wireless circuit board integrated to an amperometric genosensor for detection of UA; (b) carbon WE modified with Prussian Blue attached to Uricase via BSA for detection of UA in saliva and photos showing wireless circuits from front and back side. Reproduced from ref. 10 with permission from Elsevier, copyright 2015. (E) (a) Schematics of a smart contact lens composed of a hybrid substrate which is embedded with stretchable conductors and functional devices to detect glucose in human tears in real-time; (b) schematics showing working of the lens by wireless transmission of power which activates the LED pixel and thus the sensor for detecting glucose. As the glucose level in the tear reaches a threshold level the LED pixel switches off. Reproduced from ref. 11 with permission from the American Association for the Advancement of Science, copyright 2018. | |
The term IoW refers to electronic platforms designed to connect individuals with the digital world. It detects the interactions of the user with the surrounding environment, subsequently transferring the obtained data to a processing element that relays customized information to users, thereby improving their performance and overall quality of life. Multiple physical and chemical sensors are integrated together to form a full real-time picture of a person's health condition. However, despite its figures of merit, challenges persist regarding the selection of viable substrate materials for effective wearable sensors. Thus, the identification, quantification and analysis of biomarkers, as well as signal detection and sampling challenges, hinder reliable functioning of these devices. This can be addressed by improving constituents, fabrication techniques and design schemes.13 To mention a few examples, carbon nanotubes (CNTs) and graphene have been reported to demonstrate their suitability as ideal materials for skin-compatible electrochemical platforms of IoW sensors.5
Miniaturized electrochemical sensors are of special interest, as they play an essential role in IoW. These chemical sensors use the electrode–analyte interface to measure electrical properties such as current, impedance or potential, for obtaining information about the target analyte while keeping all operations and functionalities intact as the size is reduced. In essence, electrochemical systems provide information regarding chemical and biochemical systems by establishing a relationship between chemical/biological and electrical parameters. These devices may be integrated into everyday objects for the detection of various human health parameters such as blood oxygen saturation and heart rate.14–22 Examples of such devices include microneedle sensing platforms,23,24 electronic skin-based pressure sensors25 and in vivo microsystems.26 Karyakin and coworkers have developed a flow-through wearable biosensor based on noninvasive detection of hypoxia and diabetes in sweat.27,28 Similarly, Wang et al. highlighted the development of wearable sensing techniques in sweat for the immediate detection of alcohol without the need for performing an invasive sampling of blood. These biosensors employ two enzymes: alcohol oxidase (AOx) and alcohol dehydrogenase (ADH) to catalyze alcohol redox reactions, resulting in a substantial increase in sensitivity for alcohol detection. The detected concentrations of alcohol in sweat were in agreement with simultaneous alcohol levels examined through blood testing, thus supporting the idea of developing sweat-based wearable biosensors for the detection of alcohol.8,29,30 Most of the reported papers for the miniaturization of electrodes have used Whatman grade 1 filter paper, which are printed with photoresist or wax to define specific regions for the detection of electroactive products.31 These miniaturized microelectrodes may be integrated with microfluidic devices modified with smart materials and are promising for future IOT-based devices. Further to this discussion, organic electrochemical transistor biosensors allow the detection of both electroactive and inactive molecules via electrostatic interactions. These OECTs have a low working voltage and are easily fabricated. These sensors can easily be miniaturized to detect biomolecules, as they are not affected if the size of the device is changed. An OECT sensor integrated into a flexible microfluidic system that comprises a Au gate and a DNA probe with a detection limit for DNA as 10 pM is reported.32 Similarly a diaper embedded biosensor for detecting glucose in simulated urine was connected to a mobile device via Bluetooth and has been successful in collecting signals.33
Generally, all types of electrochemical wearable biosensor devices contain seven elements, namely a transducer that is comprised of a three-electrode system (working, counter and reference electrodes) where the surface of working electrode is immobilized by a bio-receptor, an amplifier, an electrical circuit, an analyzer, a remote transmission segment, an output device and software. The substrate part connects with a person's body, while the transducer receives signals from bio-chemical reactions triggered by the bio-receptor when it reacts with the target analyte. The amplifier circuits detect and enhance this signal from the transducer, which is further interpreted by remote transmission segment and output device. The substrate materials employed in skin sensors can be organic such as polyethylene terephthalate (PET) and polydimethyl siloxane (PDMS), economic and ecological, i.e. Ecoflex (a compostable plastic) and natural substances such as fabrics and cellulose paper. Natural substances are incorporated when sensors are needed to overlay on clothes rather than the skin.34
The principal advantages of different types of wearable sensors working in conjunction with wireless communication include portability and real-time data acquisition. However, the compatibility of materials and substrates for construction and sensing is the main challenge associated with this technology. For instance, complementary metal–oxide–semiconductor (CMOS) interfaces have been reported to be compatible when used in physical sensors. However, chemical sensors are usually incompatible with CMOS and many other microelectronic processes, necessitating frequent replacement or calibration.35,36 Thus, there is a pressing need to address the challenges emerging in the realm of chemical sensing, especially electrochemical sensing, which is the main subject of discussion in this review. Moreover, the current review specifically uncovers IoW as portable devices for electrochemical sensing in various fields of life, such as health, sport, safety at work, social interactions, military, and beyond. The focal point of this article is to present the key challenges emerging in the realm of chemical sensing, especially electrochemical sensing, as well as providing potential solutions specifically via utilization of advanced materials for the design of novel sensing interfaces.
2. Applications of wearable sensors
The applications of wearable electrochemical sensors mostly revolve around healthcare, diagnosis and fitness. However, there is growing interest in areas such as safety and security, defense and hazard mitigation, quality analysis of food and the environment, entertainment and social networking, and augmented learning. Wearable sensors lately attracted considerable attention in applications regarding fitness and healthcare since they offer instantaneous information about health performance of the wearer. Perpetual monitoring of chemical parameters is significant as it helps in the prediction of impending health risks. Several research groups have carried out advanced non-invasive innovations for monitoring biomarkers such as metabolites and heavy metals in biofluids (for example, in saliva).37 Such biofluids can be potentially used to create a pain-free route for monitoring biomarkers without blood samples, allowing immediate tracing of analytes. There have been review articles based on wearable electrochemical sensors describing the detection of mycotoxins,38 porphyrin and graphene-based sensors,39 the detection of plant molecules,40 and molecular imprinted polymer-based sensors for the detection of pesticides.41 Additionally, there are articles on wearable device sensors based on biomarkers for health monitoring. However, these articles focus on only one aspect of the topic. In contrast, this review comprehensively tries to capture novel electrochemical interfaces specifically used in IOT integrable wearable devices, which have not been previously focused on previously, while emphasizing its applications. The following are some applications of electrochemical sensors to detect biomarkers of significance that play a crucial role in healthcare and diagnosis while the ongoing research aims to convert them into wearable devices.
2.1. Healthcare and diagnosis
The most important application of health-related wearable chemical sensors is to aid in early detection and prevention of various lifestyle-related ailments such as cardiovascular diseases and diabetes mellitus.42,43 Blood glucose is usually quantified using colorimetric or amperometric biosensors, which employ dehydrogenase or oxidase enzymes to facilitate the measurement of glucose oxidation.44 Long-term stability issues associated with such enzyme-based systems have prompted researchers to conduct active research on enzyme-free glucose sensors. Despite this, glucose monitors have advanced significantly over time.45 For instance, they can detect blood glucose via interstitial fluids within a two-week timeframe,46 signifying a marked improvement in the quality of life for diabetic patients. As the saying goes a sound body portrays a sound mind, which makes fitness and sports the utmost priority for everyone with a need to measure physical fitness via wearables. The main goal of sports-related wearables is to optimize athletic performance by enabling the early detection or prevention of injuries. These devices use sweat as a real-time medium to monitor the exertion levels and hydration status during physical activities, thus aiding in maintaining peak performance.47,48 Consequently, effective management of sweat measurements necessitates the implementation of smart strategies. Some approaches use microfluidics,49–51 while others rely on lateral flow membranes or absorbents.52 Early diagnosis of biomarkers such as cytokines and creatine kinase indicative of muscle damage is essential to prevent injuries in professional sports. For example, label-free monitoring of interleukin-6 (IL-6) has been reported to measure artificial sweat via electrochemical impedance spectroscopy.53 Furthermore, monitoring dehydration is crucial in sports as even a dehydration level exceeding 2% of an individual's net body weight can lead to a significant decline in sports performance.54 Additionally, lactate and glucose can be measured in sweat using amperometric biosensors, where sweat lactate serves as an indirect indicator of physical or sweat-based gland activity.55,56
2.2. Environmental analysis
In the era of severe climate change, environmental sensing systems coupled with wearing devices allow the monitoring of CO2 emissions and detect a safe operating space. Moreover, these systems are capable of identifying hazardous environments containing volatile organic compounds, toxic gases and industrial vapors enhancing safety and environmental awareness. Wearable sensing devices have also been shown to provide early warnings regarding possible COVID-19 contamination, detecting symptoms before serious illness sets in. Additionally, real-time virus detection has been achieved using metal–organic frameworks or MOF. User-readable Radio Frequency Identification (RFID) tags integrated with smart bandages have been reported for wearable electrochemical sensor applications. Furthermore, ultra-wide band (UWB) radio tags with incorporated multiwall carbon nanotubes (MWCNTs) combined with a conductometric Cu electrode enable the detection of nitrogen dioxide levels in the environment.
In a previous report, an enhanced laser-induced graphene (LIG) printing method has been used to fabricate a sensor that can detect within 15.6 seconds with six sensors in a single set. It is a lightweight sensor, which can be linked to any portable electrochemical workstation or smartphone for real-time detection of salicylic acid, pH and neonicotinoid insecticides.57 In another work, a glove-based wearable sensor has been reported for the investigation of perilous substances such as 4-nitrophenol and picric acid. This electrochemical sensor offers real-time monitoring of contaminants assisting in public safety and a clean environment.58 Zhang, Qing, et al. reported the use of smart technology-based wearable sensors in plants for the detection of an organo-phosphorus pesticide known as methyl parathion, which is harmful for human health if in excess concentrations. This microfluidic sensor was constructed with LIG technology, which offers excellent LOD.59
2.3. Security and defense
In the realm of security and defense, technologies mirroring those utilized in sports-related wearables are often employed despite the stringent demands for reliability and robustness. For instance, smart garments and fingertip sensing devices with integrated electrochemical sensors can be used for the determination of nerve agents, nitroaromatic explosives, gunshot residues, and security threats in marine environment, highlighting their critical role in enhancing security and safety.60–62 Moreover, ensuring security in high-throughput public areas such as airports demands an efficient system for the swift detection of, and response to, toxic materials and vapors, chemical agents and oxidizers. This need is addressed by RFID-based chemical sensing systems, which enable rapid identification and mitigation of potential threats enhancing overall safety and security measures. The biochemical sensors integrated into these wearables help to monitor the level of fitness of workers, similar to applications in sports, leading to the prevention of injuries and accidents.63 Various risk factors, i.e. drugs and stress along with physical exertion and hydration level, can be detected non-invasively through saliva, sweat, urine and tears. The emotional state of workers can be non-invasively assessed by measuring the level of specific hormones (dopamine, cortisol and serotonin). This approach contributes to the safety and well-being of workers.51,64 Moreover, key environmental pollutants such as CH4, SO2 and NO2 may be detected using compact, wearable gas detection systems, and wearable air quality monitors can gather pollution data from, e.g., urban centers, to yield safe environments for healthy functioning.
3. Wearable electrochemical sensors for biomarkers
More recently, advancements that offer non-invasive monitoring of biomarkers find applications in health risk prediction and diagnosis. These sensors monitor biomarkers in biofluids such as saliva and sweat as well as cellular biomarkers. The flexible sensors fabricated with nanomaterials enable on-spot biomarker detection and fast analyte tracing. For example, these wearable sensors monitor nitric oxide (NO) released from endothelial cells, offering insights into vascular functions. The ongoing research aims to improve accuracy, sensitivity and specificity for biomedical applications.
3.1. Glucose detection
Glucose is one of the most commonly detected biomarkers for people suffering from diabetes. Monitoring the glucose levels in interstitial fluid helps to manage and control diabetes in real time.65 However, other physiological fluids, like urine, breath, and sweat, can also contain biomarkers for glucose and may be explored for concurrent detection of glucose.66 Wearable glucose sensors and smart watches based on graphene and gold composites are one of the most popular noninvasive glucose monitoring devices in the market. A bilayer formed from graphene doped with Au and Au mesh results in enriched performance as compared to undoped graphene. This is detected electrochemically using a stretchable device for monitoring glucose in sweat. This stretchable patch is then connected to a smart phone for receiving electrical signals. Besides minimizing temperature, pH, and humidity, the patch comprises polymeric microneedles for the delivery of metformin to control glucose levels. The microneedles are thermally activated.13,14 Similarly, a stretchable microfluidic device integrated into a nanoporous Au electrode in a substrate of PDMS is employed for the collection of sweat and monitoring of glucose. For onsite detection, the sensing platform in the form of patch was connected to an electrochemical analyzer via Cu wires and then wirelessly monitored via smart phone applications.67 A sensor was developed to detect glucose in saliva by incorporating it in a mouthguard support, which was initially tested in a phantom jaw. This constitutes a working Pt electrode coated with glucose oxidase enzyme (GOX) and a reference electrode formed on polyethylene terephthalate glycol and seamlessly integrated to a wireless system. The sensor can work in the range of 5–1000 μm L−1 of glucose and hence offers a painless method of sensing and monitoring glucose in dental patients.68 A non-invasive wireless sensor powered by radio frequency (RF) waves was developed by immobilizing activated GOX for determining the glucose levels in tears seamlessly. This innovative sensor was tested on a polymer-based model eye and also for protein fouling, ageing and temperature fluctuations, demonstrating good repeatability within the range of 0–2 mM.69 Remarkably, the sensor was able to read out signals over a distance of several centimeters, highlighting its potential for remote monitoring.
3.2. H2O2 detection
In vitro monitoring of cellular biomarkers in cancer cells offers significant benefits by providing insights into the process of signal transmission within the cells. This approach offers valuable insights for both cancer diagnosis and treatment aiding in the understanding of cancer mechanisms and its progression, and hence developing more effective therapeutic strategies. Among biomarkers, H2O2 serves as a reactive oxygen species (ROS) precursor secreted by liver cells, providing information about oxidative stress and cellular signaling pathways implicated in cancer development and progression. As cancer cells produce more H2O2 than normal cells as a result of their abnormal growth and proliferation, it continues to accumulate within the cancer cell, potentially leading to the development of serious diseases.61 Various flexible wearable electrochemical sensors are fabricated using specific nanomaterials for sensing H2O2 released from different cells. For example, paper-based electrochemical systems integrated with different nanomaterials are used for on-spot diagnosis.70,71 In such systems, flexible microelectrodes are preferred over rigid electrodes to prevent damage or puncture of the cell membrane. For instance, self-supporting graphene paper electrodes,72 3D graphene assemblies such as graphene aerogel, graphene network, and graphene foam73,74 as well as fiber-based microelectrodes are commonly utilized.75 The fiber-based microelectrodes appear to be particularly compatible with soft tissues for intercellular and near-cellular detection.76,77
Moreover, various fabrication materials are employed to ensure the system's sensitivity. PDMS is engaged as a substrate for stretchable membranes in flexible electronic devices.78 Similarly, carbon nanotubes (CNTs) serve as reasonable fabrication materials for flexible microelectrodes owing to their exceptional mechanical strength and large surface area.79 As CNTs show relatively low conductivity, conductive metal materials such as Au nanostructures are often electrodeposited onto the CNT films to improve the electrochemical performance for H2O2 monitoring. A flexible PDMS layered electrode was fabricated following a sequential procedure involving CNTs and Au nanostructures for immediate on-spot determination of H2O2 from human umbilical vein endothelial cells (HUVECs) and HeLa cells (Fig. 2(A)).81 While noble metallic catalysts are expensive catalytic materials, non-noble metallic catalysts can be employed as cost-effective alternatives and serve as an active electrochemical platform for H2O2 detection in liver cells. For instance, NiCo2S4@CoS2 nanohybrids, synthesized by employing NiCo2S4 with CoS2, exhibit improved redox properties as a result of its high conductivity and mixed valence state.82 Currently, the MOFs have been considered suitable fabrication materials because of the enhanced electronic conductance and provision of more active sites for catalysis. Co-based nitrogen-doped CNT materials have also been used for electrochemical H2O2 detection with consistent linearity values ranging from 0.4 to 7.2 mM. The sensor has exhibited a high sensitivity of 388 mA cm−2 mM−1, as demonstrated in experiments involving M. D. Anderson-Metastasis breast cancer commonly known as MDA-MB-231 cells and HeLa cells.83
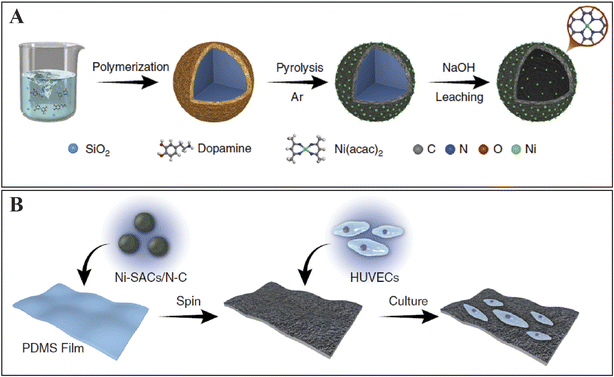 |
| Fig. 2 (A) A flexible film electrode with nano-Au/CNTs/PDMS for the onsite analysis of hydrogen peroxide production from HUVECs in the stretched state. (B) Graphical representation of (A) Ni SACs/NC composite synthesis and (B) the sensor to sense NO and HUVECs culture. Reproduced from ref. 80 with permission from Springer Nature Limited, copyright 2020. | |
Moreover, many researchers have designed numerous nanomaterials for the fabrication of microelectrodes aimed to detect H2O2 released from living tissues with an ultralow detection limit. Notable examples that have been employed for the same include graphene zero-dimensional (0D) materials and graphene quantum dots (GQDs), exhibiting peroxidase-like activity,84 such as peroxidase GQDs with AuPd nanoparticles (NPs).85 Similarly, nitrogen-doped CNT composites with AuNPs,86 rose flower-like structures (VS2@VC@NC flowers) fabricated by PdNPs,87 and coral-resembled assemblies of carbon nanospheres incorporating Pt NPs wrapped around hollow carbon tubes,88 have also been employed for detection of H2O2. Furthermore, the combination of MnO2 nanowires (NWs) on a graphene fiber (GF) modified with AuNPs (MnO2-NWs@Au-NPs@GF)89 and an N, B co-doped GF electrode90 have been used to distinguish electrochemically between cancer and tumor cells depending upon the difference in the amount of H2O2 released from these cells. However, it could be said that a substantial body of research is directed toward the expansion of advanced nanomaterials to construct flexible sensors, which serve as a foundation for constructing highly efficient electrochemical sensing platforms for monitoring H2O2 in the tumor cells and tissues. The work is still ongoing to improve the accuracy and sensitivity of H2O2 detection in biomedical applications.
3.3. NO monitoring
The release of NO molecules from endothelial cells endogenously plays a significant role in regulating vascular homeostasis and immune processes in the body. Its production appears to increase under mechanical forces, i.e. shear stress and cyclic stretch, highlighting its role in responding to physiological stimuli and maintaining vascular functions.91 However, various effective nanoparticles, i.e. metal NWs, NPs, CNTs and graphene, have been used in stretchable electronics as flexible substrates. These substrates fulfill the need for constructing efficient electrochemical sensors capable of detecting mechanically deformed cells.92,93 Au NPs applied on the PDMS membrane were employed for NO detection by consuming ecologically sound ultraviolet ray-supported technology. The approach demonstrated a significant linear detection range from 0.010 to 1.295 mM applicable in both non-stretched and stretched states.94 Furthermore, owing to improved electrochemical activity and biocompatibility, single-atom catalysts (SACs)66 have emerged as new nanomaterials of interest in various applications including electrochemical sensing, medical treatment and enzymatic catalysis.95,96 For example, N-doped hollow carbon spheres with dispersed N single atoms were layered on a flexible PDMS surface. HUVECs were then cultured on this surface to monitor NO release under stretching. It showed a high sensitivity of 430.6 nA cm−2 mM−1 and a substantially low sensitivity limit of 1.8 nM. This work unlocks an innovative pathway to manufacture a flexible sensor based on SACs for in situ determination. Following the addition of phorbol-12-myristate-13-acetate (PMA) in phosphate buffer saline (PBS), the release of H2O2 from HUVECs by comparing these conditions with and without catalase was conducted (refer to Fig. 2(B)).80
3.4. Metal ion detection
Tugba Ozer and coworkers presented an IOT-based wearable and wireless potassium sensor in sweat. Numerous types of graphite were subsequently modified on Stencil-printed carbon electrodes (SPCEs) to get the best sensor response and then added with carbon black to boost their performance. A small printed circuit board (PCB) readout device was connected with a low-power Wi-Fi for instantaneous data transmission to a cellular device app in order to monitor potassium levels using a software algorithm. The sensor displayed a potassium-selective response (<11 s) with an enhanced sensitivity of 56.1 ± 0.7 mV decade−1 and a limit of detection (LOD) of 1 × 10−5 M showing linearity in the range of 10−4–10−1 M. The cost of analysis was also reduced (<$25) as compared to other read-out devices. However, the undertaking indicates a crucial step toward the integration of both detection and data display simultaneously, making potassium sensing convenient in daily life.97 Similarly, a noninvasive sweat-based electrochemical sensor was reported, which was integrated with multiplexed flexible skin patches to monitor calcium ions (Ca2+), potassium ions (K+), and pH with IoT connectivity. This set of sensors revealed selective and repeatable tendency. Its sensitivity came out to be high, i.e. 38.33 mV dec−1 for Ca2+ ions, 64.17 mV dec−1 for K+ ions and – 46.33 mV dec−1 for pH levels. Moreover, the sensor set underwent on-body testing during a 60 min running exercise. The results indicated decreased Ca2+ ion levels and increased concentrations of K+ ions. The pH in real sweat was consistent with expectations. This newly developed sensor holds promise as an essential tool for remotely testing ionic components and studying pH levels in sweat.98
3.5. Aldehyde detection
The outbreak of pandemic diseases such as coronavirus disease (COVID-19) has underscored the urgent need for reviving our conventional healthcare analysis system, with a focus on reducing the production of waste and subsequent environmental pollution. This can be achieved with the help of electronic technology and modern computing tools such as IoT platforms, large data analysis, very large scale integration (VLSI), machine learning, deep learning, and artificial intelligence or AI to develop a real-life health analysis system.99 A cloud-based IoWT sensing system was developed to measure exposure to aldehydes (known airway irritants) among asthma patient's in real-life scenarios. This system employed a wrist-watch like sensor capable of monitoring the concentration of formaldehyde (30 ppb to 10 ppm) in air uninterruptedly, without recharging for 7 days using fuel cell technology. Additionally, the sensor was designed to operate seamlessly for an entire 7 days period, providing uninterrupted monitoring. This sensor was able to upload data wirelessly to a cellphone technology with an android operating system through Bluetooth Low Energy (BLE). This smartphone technology acted as a portal to a cloud-based informatics system for managing the sensor's analytics, data storage and its management. Moreover, the smartphone acted as a doorway to cloud-based IOWT system. This IoT sensing system could further be used to study the epidemiology of asthma, refine asthma management strategies and conduct environmental analysis.100
4. Monitoring of analytes in biofluids
It is worth mentioning here that biofluids such as saliva, sweat, and tears provide a wealth of information about an individual's health and hence can provide valuable insights into his well-being. Monitoring analytes using electrochemical sensors, coupled with IoT connectivity has emerged as a powerful approach. These biofluids contain essential information about physiological processes, disease markers and metabolic changes. Thus, the synergy of electrochemical sensing and IoT transforms biofluids into dynamic diagnostic tools, enhancing personalized healthcare and disease management.
4.1. Sweat
The growing occurrence of wearable devices has lately gained interest in immediate health monitoring and tracking body's physiological parameters. A recent investigation has been completed to develop a cost-effective device for sweat analysis (glucose and potassium ions) using selective electrochemical methods and microfluidic techniques. The wearable device monitors the levels of potassium and glucose ions in sweat when the body is engaged in any physical activity. It then issues a warming signal when the ion level reaches an experimentally set threshold concentration of 7.5 mM for potassium ions and 60 or 120 μM for glucose. The signal notifies the users of possible hypoglycemia and dehydration. Such advancements in wearable technology are potentially significant for customized health management and safeguarding, upgrading overall welfare, and adjusting performance in physical performances. Recently, enzyme-free glucose detection in sweat was reported by employing a flexible integrated wristband-based wearable sensor. This sensor was also able to transfer information to an app through Bluetooth for management regarding remote health. Moreover, during glucose detection, the evolution of hydrogen bubbles restricts the reproducibility of the electrode, which was solved by using Co MOFs encapsulating Pd NPs (Pd@ZIF-67-Zinc Imidazolite framework) as electrocatalysts. This was integrated into a sweatband along with a pliant printed circuit board for the onsite analysis of glucose in sweat.101 The device was reported to sense glucose under physiological pH conditions unlike stated in reports before, by nonenzymatic glucose sensors that operate in alkaline buffers. Additionally, it enabled wearable, maintenance-free tracking of glucose in perspiration over an extended period and holds promise for non-invasive clinical analysis and application in sports.
4.2. Saliva
Saliva is one of the non-invasive fluids with potentially significant biomarkers for unveiling physiological conditions. It majorly consists of proteins, aqueous, organic and inorganic elements. Saliva is used as a biofluid substitute for assessing disease biomarkers by employing simple, non-invasive testing without instigating pain. The changes in the concentration of saliva can be used to diagnose diseases and for health check in detailed analysis since blood constituents can pass into saliva via facilitated diffusion.102 To date, it is challenging to monitor electrolytes in saliva using a sensor. However, researchers are employing electrochemical sensors in devices for this purpose. A study reported fully integrated wearable sensor arrays for real-time measurements of targeted analytes in saliva. Further, the COVID-19 pandemic episode highlighted the urgency to revamp our healthcare systems where a lot of environmental toxic wastes are generated, which results in infection outbreaks among patients who have to visit a healthcare official. Hence, to address these issues, nano-biosensors have been developed for robust and sensitive detection of diseases. These biosensors are in the form of wearables that enable remote health monitoring by using IoT and machine learning, and hence, help in real-time diagnostics.99,103 A study reported fully integrated wearable sensor array for the real-time measurement of targeted analytes in saliva. The fully integrated sensor for remote, non-intrusive, and concurrent measurement of several metabolites and electrolytic ions such as Na+ and H+ in saliva or sweat was developed by combining sensing, electronic and microfluidic units in a continuous mode. The efficacy of the developed sensor was assessed for a multitude of electrolytes in healthy individuals and gout active patients by administering a purine-rich meal and medicated-care control regime, respectively. Such monitoring of numerous electrolytes and metabolites through single universal integrated on-body sensors might lead to better comprehension of the biomarkers in a human body with an added benefit of personal health management103 In a recent study, an electrochemical sensor based on graphitic carbon nitride was developed to detect interleukin-8 in saliva. Interleukin-8 is a cytokine linked to oral cancer. The sensor is highly sensitive with an excellent LOD and is a great advancement towards point-of-care diagnostics.104 In another study, a stationery electrochemical power-angle paper-based sensor was reported to test iodide and dopamine concentrations in saliva samples. Following low-cost miniaturization, the hydrophobic layouts on paper substrates were simple and robustly drawn with a crayon pencil devoid of cutting-edge tools.102
4.3. Tears
Non-invasive detection of biofluids is possible by various sensing platforms, while analyte monitoring for different physiological biomarkers can be carried out on different body sites.100 The tear is an interstitial fluid comprised of metabolites, electrolytes, and proteins obtained from glands such as goblet cells, lacrimal glands and ocular surface epithelial cells.105,106 Although there are generic differences between the composition of blood and human tears, they correlate due to leakage of certain metabolites into tears while blood is supplied to the brain. This makes it easier to create tear proxies to simulate metabolites' blood level. A study has reported the use of either functionalized contact lenses or merging them with miniscule sensors (such as glaucoma detection) for diagnostic monitoring of tear metabolites.107 Recent developments in biosensors, transparent electrode materials and telecom technologies have led to intelligent contact lenses that are controlled remotely and able to provide continuous tear-glucose readings (research done by Google and Novartis). Yao et al.,108 have also reported polyethylene terephthalate (PET) contact lenses for glucose monitoring using a simple three-electrode electrochemical sensor.109 Photoresist and thermal evaporation of titanium and platinum on a PET-coated wafer was used to make the electrodes. The glucose oxidase was immobilized by covering the contact lens surface with a passivation layer of titanium oxide. Probable interferences from ascorbic acid (AA) and lactate were reduced using a Nafion layer.110 Wearable electrochemical sensors potentially measure biomarkers (cortisol, glucose, etc.) in individuals assisting health information management and fitness. These function by operating at low power by applying low potentials, and hence, they are safe for various applications. Basically, electrochemical sensors employ electrodes to convert a chemical reaction into an electrical signal. In order to enhance electrode performance, transducers are immobilized with bioreceptors (enzymes, antibodies, aptamers, etc.) such that their activity is not hindered. Consequently, these transducer materials are required to have a high electrical conductivity that could detect small electrical changes at the electrode–electrolyte interface. Moreover, these can be miniaturized with ease, are economic and robust, and exhibit compatibility with wearable electronics that makes them ideal for the body.
5. Novel interfaces and advanced materials
The signal transmission and transduction in biosensing systems are significant for stable, accurate and sensitive outgoing signals. The electrical conductivity of a material is considered a basis for ensuring standard signal transmission. Moreover, the flexibility and adhesiveness of materials need to be investigated, as wearable devices are supposed to fix tightly to human skin to prevent signal interference and distortion during transmission. The hydrophobicity of the materials acts as a support in preventing interferences due to sweat. Consequently, fabricating engineered materials possessing any of the above-mentioned desired qualities is necessary for wearable sensing systems. In this regard, researchers have been getting inspiration from nature as numerous organisms occurring in nature have evolved as ingenious, complex and robust sensing systems. For instance, the lateral line system of fishes can sense changes such as velocity and viscosity of nearby running water, assisting in finding direction and detecting objects in water.111 Consequently, the enhancement of integration capability and stability is significant for the advancement of the sensor system with regard to advanced materials for wearable electrochemical sensors, as listed in Table 1 in Section 5.5.
Table 1 Wearable electrochemical sensors for tailored health monitoring
Sr. No. |
Analyte |
Modification |
Detection technique |
LOD |
Ref. |
1 |
Tyrosinase |
CP/catechol-agar |
Chronoamperometry (CA) |
Not listed |
112 |
2 |
Lactate |
CNTs/Ti3C2Tx/PB/CFMs/chitosan-LDH-BSA |
CA |
Not listed |
113 |
GO NSs/PA/PANHS/LOx |
EIS |
1 Mm |
114 |
3 |
Glucose |
CNTs/Ti3C2Tx/PB/CFMs/chitosan-GOx-BSA |
CA |
Not listed |
113 |
CNFs/chitosan-GO/GOx |
Colorimetry |
0.1 mM |
115 |
PET/CoWO4/CNTs/PANI/Silbione |
CA |
1.3 μM |
116 |
PB-CI/BSA-AOx/PU/PVA |
CA |
Not listed |
117 |
Multiplayer PB/GOx |
CA |
1 μM |
118 |
GOx-AuNPs/N-GQDs-PMOF |
Amperometric |
0.7 μM |
119 |
GOD-GA-Ni/Cu-MOFsFET |
Amperometric |
0.51 μM |
120 |
4 |
Radial muscle contraction |
PLA/paper-Ti3C2Tx/PLA |
CA, frequency |
10.2 Pa |
121 |
5 |
Urea |
CNFs/chitosan-GO/urease |
Colorimetry |
30 mM |
115 |
6 |
Potassium |
CI/PDMS/LSG/MWCNTs |
Potentiometry |
10−4.9 |
122 |
7 |
Cortisol |
PDMS/Ecoflex/AgNPs/MIP/NIP |
Conductivity |
2 ng mL−1 |
123 |
8 |
Ph |
PET/CoWO4/CNTs/PANI/Silbione |
Potentiometry |
Not listed |
116 |
9 |
Alcohol |
PB-CI/chitosan-Gox/agarose |
CA |
Not listed |
117 |
10 |
Hydrogen peroxide |
PMMA/PB-CI/Whatman/PMMA |
CV, CA |
Not listed |
124 |
11 |
SARS-CoV2 virus |
Apt/polyUiO-66 @AgNPs |
DPV |
Not listed |
125 |
12 |
H1N1 virus |
Ab/poly UiO-66 @AgNPs |
DPV |
49.4 fg mL−1 |
126 |
13 |
Tuberculosis antigen |
Aptamer/HRP/AuNP/UiO-66-NH2 |
DPV |
10.0 fg mL−1 |
127 |
14 |
mecA and nuc gene |
UiO-66/BMZIF-derived NPCs |
DPV |
3.7 fM |
128 |
15 |
Escherichia coli K12 |
MOFs-Ab-E. coli-AuNP |
CV |
1.0 cfu mL−1 |
129 |
Ab/Cu3(BTC)2-PANI |
Impedimetric |
2.0 cfu mL−1 |
130 |
16 |
Vibrio parahaemolyticus |
Fe3O4@NMOF-Apt |
SWV |
3.0 cfu mL−1 |
131 |
17 |
Pseudomonas aeruginosa |
Cu-ZrMOF@Aptamer@DNA |
DPV |
Not listed |
132 |
18 |
Carcinoembryonic antigen |
Pt@CuMOFs-hGq-GOx |
Impedimetric |
0.023 pg mL−1 |
133 |
19 |
T4 polynucleotide kinase |
Fe-MOF/AuNPs/DNA |
DPV |
3.5 × 10−4 U mL−1 |
133 |
FeMOF@AuNPs-hairpin |
CV |
2.344 × 10−4 |
134 |
20 |
Mucin |
Cu-MOF-GO |
DPV |
0.033 pM |
135 |
21 |
Thrombin |
AP II/AuNPs/Ni-MOF |
DPV |
0.016 pM |
136 |
22 |
Uric acid |
Au@NC@GC |
DPV |
10.0–150.0 μM |
137 |
23 |
Dopamine |
ZIF-8/PPy@SiO2-MEPCM and laccase |
DPV |
0.0069 μM |
138 |
Mn-BDC@MWCNTs |
DPV |
0.002 μM |
139 |
24 |
Lactose |
Co-hemin MOF/chitosan/PcCDH |
Amperometric |
4.0 mM |
140 |
25 |
Carbohydrate antigen 125 |
MOF-808/CNT/Ab |
DPV |
0.5 pg mL−1 |
141 |
26 |
Ciprofloxacin |
NH2–UiO-66/RGO |
ASV |
6.67 nM |
142 |
5.1. Metal–organic frameworks (MOFs)
Despite their low electrical conductivity and fragile mechanical characteristics, MOFs are widely used for chemical sensing owing to their selectivity and precision for the detection of analytes, for their outstanding catalytic capabilities and energy generation. For instance, Shuqi Wang and coworkers developed fiber-like Nickel triphenylene-fused metal catecholate (NiCAT4) on CNT fiber-ion selective electrode (CNT@ISE)-NiCAT4@CNTF-RE and Nafion/NiCAT4@CNTF-ISE, for fabricating a sensor band intended to be worn on the head in order to monitor continuous flow of sweat without direct interaction of the skin, as shown in Fig. 3(A).106 This device showed biocompatibility, good flexibility, and mechanical stability. A small electrochemical workstation attached with this device allowed it to connect the on-spot potentiometric signals to the smart phone via wireless connection. In this way, the wearable sensor monitored the sweat with smooth and spikes-free signal responses. The calculated sodium concentration ranges up to 80 mmol L−1 in sweat, which is in line with the normal Na+ level (10–90 mmol L−1) during active sweating session. To assess the stability of the as-fabricated material, constant-current chronopotentiometry was employed to study Nafion/NiCAT4@CNTF-ISE and Nafion@CNTF-ISE. Furthermore, improved sensing performance was observed, attributed to enhanced double-layer capacitance and improved conductivity of solid-contact transducers based on NiCAT4 and Nafion. The negative potential shift was observed for both NiCAT@CNTF-ISE and CNTF-ISE in the interfering ion solution as compared with the primary ion solution, indicating the presence of hydration spheres at the electrode and solution interface.106
 |
| Fig. 3 Illustration of (A) a wearable band-based sensor to detect on-body sweat. Reproduced from ref. 106 with permission from Elsevier, copyright 2022. Wearable levodopa sweat sensors with construction details. (B) Levodopa sensor. (C) Functionalized electrodes. Reproduced from ref. 143 with permission from the American Chemical Society, copyright 2019. Mechanism of uric acid sensing. (D) Real-time uric acid detection in sweat with high-purine food intake. (E) Sensing process of the uric acid sensor. Reproduced from ref. 144 with permission from Elsevier, copyright 2022. | |
Similarly, Jingyu Xiao and coworkers displayed a wearable sweat levodopa (LD) sensor. The LD is the first-line drug for the treatment of Parkinson's disease. The sensor analyzes sweat and contains a flexible electrode to analyze LD that is integrated into a minute electronic circuit for its wireless analysis (Fig. 3B). The WE was fabricated by immobilizing several layers of GO/MOF/enzyme on the surface of the electrode, in the form of a composite using screen printing technology to ensure the accuracy and selectivity for LD in sweat. The functional surface of the electrode was modified with tyrosinase coated on ZIF-8/GO. The role of ZIF-8/GO composite was to provide a wide interface for the incorporation of tyrosinase in order to enhance the sensing performance, while tyrosinase was used to catalyze the oxidation of LD to dopaquinone. This monitoring of LD was carried out in real time by studying the current response of this reaction.107,143 Moreover, Shu and coworkers developed a rapid, uninterrupted, and highly accurate method of detecting glucose concentrations in sweat by fabricating a highly flexible fiber-based wearable sensing device using Ni–Co MOF/Ag/rGO/PU. The Ni–Co MOF's fiber-based sensor displayed a high surface area and outstanding catalytic activity to achieve a linearity of 10 μM–0.66 mM and a sensitivity of 425.9 μA mM−1 cm−2. The sensor was highly selective with excellent storage stability along with mechanical deformation strength, including remarkable bending and stretching stabilities.145 Additionally, Zhao et al., were the first to develop a hybrid core–shell multifunctional MOF-based 3D CNF network (CNFN) by specifically using copper catecholate (Cu-CAT) employing hydrothermal and electrospinning techniques.109 The flexible CuCAT@CNFN-based wearable pressure sensor demonstrated a fast recovery time of 0.24 s, high sensing range from 0.5 kPa to 60 kPa, exceptional stability for over 5000 cycles and reliable air permeability, and can effectively captivate joint movement such as finger gestures. In future, this technology could be applied in smart home gadgets by integrating pressure sensors, supercapacitors (SCs) and solar cells into multipurpose, self-powered systems.
Wearable and flexible real-time uric acid (UA) sensors in sweat were developed by integrating the sensing platform uricase@MAF-7 (3-methyl-1,2,4-triazolate framework (MAF)) with a microchip and wireless board for the collection of sweat and monitoring particularly after consuming high-purine meals, as shown in Fig. 3(D). The UA is a byproduct of metabolism of purine and an increase may indicate consumption of purine-based diets. In this study, MAF-7 was integrated with an urease enzyme, where the composite MOF/GO(graphene oxide)/enzyme was then deposited onto a gold working electrode (WE) to realize a higher sensitivity and sustain enzyme functioning in severe environments.144 The WE was fabricated with several layers of the composite, as shown in Fig. 3(C). The inclusion of MAF-7 improves the sensor's antifouling performance and stability by protecting and loading the enzyme onto the sensor. Further modification with a Nafion layer contributed to these improvements.110,146 Fig. 3(E) depicts the oxidation of UA to allantoin by the respective catalyst uricase. The inaccuracies due to the evaporation of sweat and contamination of skin in conventional sweat sampling were reduced by microfluidics-based sweat sampling.111,147 Embedded on a microfluidic chip was a microchamber and several microchannels (the inlet port) required to collect samples of sweat. Capillary action facilitated the transport of sweat to the microchamber through the microchannels, while excess sweat was drained via the outlet. Furthermore, the feasibility of this microfluidic chip was assessed by introducing a blue colored water drop at the input and checking whether it fills the chamber or not. No visible leakage through the whole chamber ensured reliable sweat sampling. The collected sweat samples were transferred wirelessly to the user's computer or mobile device through Bluetooth.148,149 Similarly, Zhu and coworkers used a Pd core-shelled NP electrocatalyst in Co ZIF-67 for the analysis of wearable glucose perspiration by nonenzymatic, water splitting-based electrochemical sensors.101
5.2. Carbon nanotubes (CNTs)
Various nanomaterials including NPs, CNTs, molecularly imprinted nanostructures, quantum dots, inorganic nanorods/nanowires, and many more have been used widely as fabrication materials to enhance the sensitivity of interface of the sensor for biomedical diagnostics.150–155 Recently, electroactive nanoprobes have been reviewed broadly for the determination of analytes of interest by electrochemical sensing.156–159 They also provide new tools for the diagnosis of hepatocellular carcinoma,160 analysis of living cells integrated with electrochemical cytosensors,161 and detection of cancer cells based on folate receptors.162 Such electrodes have also been used for the analysis of cancer biomarkers and anticancer drugs, showing their great analytical performance.163 Moreover, Pingarron et al., have succeeded in expanding the portfolio of biosensing devices to provide precise information about the specific location of tissues. In this context, nanomaterials have been recognized as outstanding materials for effective drug delivery by preventing biosystems from the non-specific interactions and reducing multi-drug resistance. Additionally, nanomaterials enable direct drug delivery to the ailing tissue in the organism, enhancing therapeutic efficacy.164,165 In addition, thin films of single-walled carbon nanotubes (SWCNTs) were used as stretchable materials in wearable electrochemical health devices. A dry and clean fabrication process was selected for the formation of pristine and high-quality SWCNT films incorporating a protective layer to mitigate the risk of surface contamination from other fabrication processes during electrochemical activity.166 Such electrodes were found to exhibit a low electron transfer rate. In a further study, the electrochemical response of SWCNT electrodes was improved by the successful functionalization of electrodes with a H2SO4 solution using inner redox probes such as Fe(CN)64−/3−. This electrochemical functionalization of the working electrode came out to be effective for improving the limit of detection to about ∼100 nM for the detection of dopamine with such a flexible SWNT electrode.167
5.3. Polymer or polymeric membrane
The demand for innovative and highly efficient modifiers with excellent redox, thermal and chemical resistance for the electrode is often met by using polymers such as thin films of modern stimulus-responsive polymers,168 synthetic polymers169 and polymer nanocomposites.170 Among them, photoactive polymers as well as molecularly imprinted polymers are well designed and environmental friendly polymers having the potential to be used for many selected applications.171 One application of polymer membrane layers used as a sensing platform on the electrode surface is to develop a reliable, portable and low-cost diagnostic platform for biomarkers in human fluids.172 All of these polymer membranes basically act as a protective barrier against interferences caused by human fluids such as serum, blood, urine or plasma samples on the electrode surface, allowing for the monitoring of analytes.173 Additionally, when serving as an external protective layer for electrodes, they ensure accurate measurements of signals originating from the desired molecule by shielding the surface of electrochemical biosensors against unwanted non-specific interactions, fouling, and signal interferences.174 Electropolymerization technique is extensively used for the electrode surface assembly owing to its wide range of advantages including easy implementation, signal enhancement, high reproducibility, compatibility with transducers and protection of bio-recognition elements. Electropolymerized materials on DNA receptor elements have been incorporated successfully to form innovative polyelectrolyte complexes.65 Various polymers including polypyrrole, polyaniline, polyphenazines, polythiophene and their derivatives have been used as sensing materials. Moreover, Au NPs and poly-aminothiophenol175 as well as polymeric and oligomeric forms of lactic acid176 have been electropolymerized for the formation of a biocompatible, cost-effective and durable film on the electrode surface. Another research group introduced a wearable electrochemical sensor based on polyaniline thin films immobilized on the flexible polyethylene terephthalate substrate coated with indium tin oxide (ITO) for sweat analysis. The potentiostatic deposition technique was used to obtain polyaniline thin films by applying a constant potential of +2 V vs. SCE (saturated calomel electrode) initially for 90 s, followed by its modification with reduced graphene oxide at +8 V of constant potential vs. SCE for 200 s. Subsequently, the electrodeposition of polyaniline thin films was conducted to improve the sensor performance. This fabricated electrode showed good response with 62.3 mV pH−1 sensitivity (approximately similar to Nernstian response), improved selectivity among other ions with minimal interference effect and 3.8% reproducibility.175
5.4. Transition metal chalcogenides (TMCs)
Two-dimensional materials are considered promising for the fabrication of stretchable wearable sensors for safety and health monitoring. Conventionally, the materials used are polymers, silicon, and inorganic oxides, but unfortunately such materials have encountered problems such as low conductivity, high cost and rigidity that impede their practical use. The TMCs belong to such a class of 2-D compounds (metal sulfides) that have demonstrated promising results in integrated wearable sensors owing to their unique layered structure. The TMC exhibit adjustable properties such as transparency, band transitions and elasticity, which make them promising materials for wearable sensors.177 Although it is still challenging to manufacture high-quality wearable sensors due to toxic solvents and crystal formation of TMCs, a study reported zwitterion cocamidopropyl betaine (CAB) acting as a surfactant to support liquid-phase exfoliation and homogeneous dispersion of single-phase crystalline TMC in solvents such as water and isopropanol. In the absence of binders or additives, several stable TMC inks were directly produced for deposition on flexible substrates for wearable sensing electronics.175,178
5.5. MXenes
Continuous and real-time sensors have been receiving much attention lately, in biomarker monitoring, therapeutic agent tracking and toxicity assessment. Nevertheless, such sensors are still in development stage regarding on-site applications due to several factors including short service life liability to fouling, poor repeatability, signal drifting, etc. Furthermore, present successful methods suggest more sample pretreatment and timely procurement of testing results. In this regard, a class of novel 2D nanomaterials such as transition metal carbides, nitrides and carbonitrides referred to as MXenes exhibit high electrical conductivity and a large surface area due to abundant terminal groups. These characteristics enable them to serve in various functions and structural designs, rendering them as excellent candidates for the construction of wearable sensing devices. The electrical properties of MXenes can be tailored to selectively adsorb biomolecules (e.g., glucose and dopamine) via surface modification. MXenes have been engaged to fabricate biosensors for various biofluids. For example, an organ-like titanium carbide (Ti3C2Tx) is used as an electrochemical sensing matrix for electrocatalysis and enzyme loading. The first use of enzyme-based detection mechanism of H2O2 via Ti3C2 was reported by Yang and Gao,3 wherein hemoglobin was immobilized on the MXene surface facilitating direct electron transfer and target molecule detection. Hemoglobin immobilization for the detection of nitrite was also reported, wherein Ti3C2Tx was used to fabricate a mediator-free biosensor. Similarly, other enzymes such as acetylcholinesterase (AChE) and tyrosinase were successfully immobilized on MXene surfaces.170,173 Chemical additives have been shown to increase the sensitivity and detection performance of MXenes. For instance, TiO2 nanoparticle-modified Ti3C2 generated highly improved detection of H2O2. Gold-based MXene nanocomposites (Au/MXene) have been reported for biosensing applications via glucose immobilization. The Au/MXene-based platform was shown to possess excellent electrocatalytic properties, and enhanced electron transfer between the GOx enzyme and the WE was observed. Surface treatment such as HF etching has been shown to enhance the electrochemical properties of Ti3C2Tx for the construction of an electrochemical biosensor to detect carbendazim (CBZ).179,180 Similarly, Nafion-coated Ti3C2Tx was employed to construct a high-performance electrochemical detector for the neurotransmitter dopamine, demonstrating an LOD of 3 nM. Nobel metal nanoparticle (Au, Pt and Pb)-based structures were constructed on MXene sheets to yield a superoxide detecting biosensor that exhibits excellent linearity in the range of 0.4–9.5 × 10−6 M and an LOD of 0.2 × 10−6 M with enhanced catalytic interactions during the sensing mechanism. A novel electrochemical biosensor with urease-immobilized MXene nanosheets integrated in a microfluidic chip was developed for analysis of whole blood.19,181 Sensing platforms based on the nanocomposites of MXenes provide improved detection performance, high repeatability and stability, and are constructed by combining Ti3C2Tx with various nanomaterials. As an example, a nanocomposite consisting of platinum, polyaniline and Ti3C2 (Pt/PANI/MXene) was engaged for the detection of H2O2 and lactate. The modified setup provided improved response towards H2O2 with a linear range of 0.0005 to 5.0 mM for lactate. Another hybrid nanocomposite sensor, composed of palladium NPs on MXene sheets, demonstrated electrochemical detection of L-cysteine, with the sensing range of 0.5–10 μM and an LOD of 0.14 μM.182 A study of electrochemical detection of the neurotransmitter epinephrine was conducted using graphene oxide (GOx)/Ti3C2Tx MXene (GMA) sheets situated onto indium tin oxide (ITO) to construct a GMA/ITO electrode. A similar study using an MXene/Graphene hybrid was reported for the detection of glucose from GOx, exhibiting enhanced electrocatalytic capability for glucose sensing. In another study, the non-enzymatic detection of glucose was achieved using a Ti3C2Tx MXene/Ni–Co–double layered hydroxide (LDH) sensor demonstrating excellent linearity in the range of 0.002–4.096 mM. It was further proven that the presence of functional groups on the surface of MXenes enhances their ability to immobilize active analytes.183 Another MXene hybrid combining Ti3C2Tx nanosheets with immobilized tetrahedral DNA structures demonstrated mycotoxin sensing using a gliotoxin aptamer with a sensing range of 5 to 10 pM with an LOD of 5 pM. A high-performance hybrid sensing platform for the detection of acetaminophen was reported where in situ crosslinking of amino-functionalized MWCNTs with polydopamine-functionalized Ti3C2Tx showed increased mechanical stability and excellent electrochemical response. A similar combination of electrocatalytic MXene/NH2-CNT sensor for selective sensing of fisetin was reported, showcasing enhanced surface area. Likewise for monitoring cholesterol, a nanocomposite-based biosensor was developed by using Chit/Ti3C2Tx to immobilize cholesterol oxidase (ChOx) enzyme. The study showed enhanced electrical conductivity, favorable selectivity and practicability. Moreover, Au/MWCNTs/Ti3C2Tx was reported for the detection of HM ions such as Cu and Zn. Furthermore, mixed-phase MXene nanosheets were reported for the simultaneous detection of multiple biomolecules such as AA, dopamine and UA.184
Despite the various reported works exhibiting the development of MXene-based electrochemical biosensors, there is only a single case of wearable biosensor based on MXenes that we could unearth. In the study, a wearable, multifunctional biosensor was presented, designing a unique composite of MXene/Prussian blue (Ti3C2Tx/PB)1 to diagnose glucose and lactate in sweat, in order to overcome issues in sweat-based sensors such as compromised sensitivity and short detection range. The reported novel modular design, showcasing a three-phase CNTs/Ti3C2/PB electrode, exhibited enhanced sensor performance and stability. The sensor was shown to simultaneously measure signals of glucose and lactate with high sensitivity. The overall sensor design consisted of a sweat-collecting layer, sensing layer and covering layer. Therein, sweat collection is followed by pH, glucose, and lactate level detection via the insertion of active sensors. The process is facilitated by soft silicon rubber allowing oxygen diffusion towards the active sensor layer for protection from an open environment. Electrochemical nanobiosensors with MXene additives have shown promise to detect different types of biomolecules such as piroxicam, AA, and UA.185 In another investigation, nanocomposites based on MXene/CNT/PB were designed to fabricate a nanobiosensor for glucose and lactate monitoring.186 Primarily, the sensor was modified with H2O2 secreting enzymes (lactate and glucose) that also cause the ionization of PB dye. The produced ions react with MXenes, initiating a redox reaction with enhanced electrochemical sensing, demonstrating an LOD of 0.33 × 10−6 M for glucose and an LOD of 0.67 × 10−6 M for lactate.
An MXene- and CNT-based CuMOF composite nanosensor had been fabricated to detect tyrosine. Entrenching MXenes with CNTs prevents their aggregation and altering them with CuMOF porous octahedral particles produces richly porous and catalytic composites. Sensors displayed high stability and linearity in the range of 0.53–232.46 μM with an LOD of 0.19 μM.187
An exceedingly responsive electrochemical sensor has been fabricated to detect nitrile via a direct green synthesis procedure. Xylan-based CQDs have been used as in situ reducing agents for the preparation of CQD-covered gold nanoparticles, i.e. Au@CQDs. An electrically conductive MXene had been used as an immobilized matrix for the preparation of an electrode nanocomposite sensor as Au@CQDs/MXene, which was later loaded onto GCE. The fabricated sensor had been amply responsive, showed extensive ranged linearity, and exceptional selectivity because of AuNP and CQD catalytic activity. Under the most favorable conditions, the linearity for the sensor ranged from 1 μM to 3200 μM with an LOD of 0.078 μM. These values have been superior to most sensors that were reported in the literature by a differential pulse voltammetry (DPV) method.188
Table 1 presents a summary of wearable sensors with significant implications for healthcare.
6. Advancement in flexible wearable electrochemical sensing devices
As flexible wearable electrochemical biosensors are quite minute and manageable, they can be directly mounted onto the skin for constant noninvasive analysis of analytes in the humans. Their assembly with integrated circuits has displayed robust electronic responses and effective capabilities of data processing. They also provide an important platform to overcome various problems in real-time monitoring and remote health management, combined with precision medicine and flexible bioelectronics technology.19,181 Moreover, they have been identified as tools to meet complex medical monitoring requirements due to their improved structure and the progress of flexible electronics and nanomaterials. These flexible biosensors not only represent better sensing ability but also display strong practicability, having wide-ranging applications in clinical and commercial health prospects.182,189
The conventional method of sweat analysis has several problems such as cumbersome collection, transportation processes and delayed information. Multiple fabrication techniques have been proved practical for wearable electrochemical sensors to overcome these limitations and offer ways for in situ real-time sweat determination. For instance, the progress of micro-devices has been promoted by microfluidics for developing wearable devices that can gather, sample and analyze sweat.102,176 Furthermore, the application of near-field communication (NFC) technology offers battery-free alternatives in comparison to Bluetooth technology for developing wireless electronic devices for sensing purposes.183 In addition, a steroid hormone was detected by DPV in sweat by employing a flexible cortisol patch device integrated with a NFC chip. It was connected to the surface of skin for data collection that was transported wirelessly to the NFC-based smartphone. However, it provides a solution for analysis of cortisol biomarkers in sweat.184 Similarly, the analysis of glucose levels in sweat is widely reported via wearable biosensors connected with bioelectronics,185,190,191 but the data for nonenzymatic wearable sensors based on bioelectronics and nanomaterials for glucose are rarely reported.
The growth of IoT has made edge computing (involves connecting numerous computing and storage devices to generate a large amount of data) highly significant.187 It can be executed by using multiple technologies, i.e. fog computing, cloudlets and micro data centers, making networking, storage and computation abilities to be installed at the place of data generating unit.188,192 In a healthcare study, a decision-making model and an IoT-based system were opted for sensing and analysis of type 2 diabetes patients by utilizing type 2 neutrosophic and Višekriterijumsko Kompromisno Rangiranje (VIKOR) methods. The results demonstrated the success of the proposed method showing 9.8% execution time reduction and patient's increased survival rates depending upon symptoms and personal data. Nevertheless, with research, the prediction accuracy could be improved by delving more into advanced machine learning methods. For instance, Yang and coworkers187 presented an emotion-alert system by engaging an edge cloud and a personal robot. This system engages users by collecting audio/video data, EEG data, physiological signals and touch data through smart clothing. The system's performance was checked by conducting several tests via a specially developed testbed. The results revealed improved mental health of people by the proposed emotion-alert system. Similarly, Chen and coworkers suggested a healthcare system based on Elliptic Curve Cryptography (ECC), which uses a data-driven approach and edge cognitive computing to investigate data related to the physical health of users. The experimental results displayed superior user data cognition, enhanced energy efficiency, cost effectivity, great resource cognition, and enhanced survival rates attributed to the system's rationalized approach. However, it can be perceived that existing technology combined with this system may offer better care opportunities.
A biosensor employs potentiostat for power but recently researchers have shifted towards wearable electrochemical biosensors that are self-powered by biofuels193 Based on this, Yu et al.,194 developed a self-powered wearable sensing platform for the detection of sweat with integrated micro-SCs. NiCo2O4 has been recognized for its utility in electrochemical sensing devices and SCs, owing to its impressive theoretical specific capacitance and outstanding electrochemical catalytic activity.194,195 Moreover, a micro-SC based on NiCo2O4 was developed for the detection of Na+, K+ and glucose. The resulting sensor exhibited an enhanced sensitivity of 0.5 mA mM−1 and a high capacitance of 18.5 mF cm−2. It was further connected with wireless transfer technology, in order to display the personal state of perspiration on a smartphone for the on-spot management of personal health.196
Additionally, some wearable sensors such as ring or glove-shaped sensors modified with nanomaterials for the detection of small molecules are designed to be worn on hand.7,197 For instance, a ring-shaped plastic device was constructed and implanted with three plastic carbon-based electrodes by a 3D printing technology. Au films electrodeposited onto electrode surfaces serve as active materials for glucose analysis. The resultant e-ring was subsequently integrated with a mini potentiostat for transmitting data to a mobile phone directly.198 In order for sensing devices to completely adapt to the irregular or soft body surfaces, textile industry has been adept at incorporating sensing elements. The collaboration aims to build soft fabrics with improved electrochemical characteristics.199
7. Conclusion
The IoT is a vast technological framework that offers numerous benefits across various domains. It has transformative power in bringing convenience and comfort in our daily lives. The IoT healthcare system is an outstanding service application system that individuals can use by wearing body-worn devices to monitor their health. Enabled by IoT technology, this system integrates various devices and sensors facilitating continuous health monitoring and, hence, helps in regulating human health. This allows for the early detection of health issues and enables personalized interventions. The developments in the IoT and nanotechnology have revolutionized data acquisition in real time, especially in areas with limited resources. It holds great promise for addressing health, welfare and safety concerns. It helps in the early diagnosis of various diseases irrespective of place and time. It facilitates users with quick medical assistance and services. Several sensors can be attached with smartphones, wristbands, tattoos, watches, soft lenses and belts to analyze various aspects of the body including glucose level, body movements, blood pressure and heart rate. The data are then transmitted to the concerned physicians for analysis and monitoring. Moreover, specially designed electrochemical sensing systems are capable of identifying hazardous environments containing volatile organic compounds, toxic gases and industrial vapors, enhancing safety and environmental awareness. The designed electrochemical sensors can offer real-time monitoring of contaminants assisting in public safety and a clean environment. Thus, with the capability to seamlessly gather and analyze data, the IoT can promote proactive healthcare management, safety and security.
Data availability
The authors declare that the data are available in this manuscript in the form of tables and figures.
Conflicts of interest
The authors declare no conflict of interest regarding the publication of this manuscript.
Acknowledgements
We acknowledge the support of Higher Education Commission of Pakistan for supporting this work through NRPU project no. 17576.
References
- E. W. Brown, et al., ACEstat: a diy guide to unlocking the potential of integrated circuit potentiostats for open-source electrochemical analysis, Anal. Chem., 2022, 94(12), 4906–4912 CrossRef CAS.
- E. Monton, et al., Body area network for wireless patient monitoring, IET Commun., 2008, 2(2), 215–222 CrossRef.
- Y. Yang and W. Gao, Wearable and flexible electronics for continuous molecular monitoring, Chem. Soc. Rev., 2019, 48(6), 1465–1491 RSC.
- D. Quesada-González and A. Merkoçi, Nanomaterial-based devices for point-of-care diagnostic applications, Chem. Soc. Rev., 2018, 47(13), 4697–4709 RSC.
- A. Sharma, et al., Involvement of metal organic frameworks in wearable electrochemical sensor for efficient performance, Trends Environ. Anal. Chem., 2023, 38, e00200 CrossRef CAS.
- L. C. Tai, et al., Methylxanthine drug monitoring with wearable sweat sensors, Adv. Mater., 2018, 30(23), 1707442 CrossRef PubMed.
- A. Barfidokht, et al., Wearable electrochemical glove-based sensor for rapid and on-site detection of fentanyl, Sens. Actuators, B, 2019, 296, 126422 CrossRef CAS.
- J. Kim, et al., Simultaneous monitoring of sweat and interstitial fluid using a single wearable biosensor platform, Advanced Science, 2018, 5(10), 1800880 CrossRef.
- S. Emaminejad, et al., Autonomous sweat extraction and analysis applied to cystic fibrosis and glucose monitoring using a fully integrated wearable platform, Proc. Natl. Acad. Sci., 2017, 114(18), 4625–4630 CrossRef CAS PubMed.
- J. Kim, et al., Wearable salivary uric acid mouthguard biosensor with integrated wireless electronics, Biosens. Bioelectron., 2015, 74, 1061–1068 CrossRef CAS PubMed.
- J. Park, et al., Soft, smart contact lenses with integrations of wireless circuits, glucose sensors, and displays, Sci. Adv., 2018, 4(1), eaap9841 CrossRef.
- J. Kim, et al., Wearable biosensors for healthcare monitoring, Nat. Biotechnol., 2019, 37(4), 389–406 CrossRef CAS.
- H. Lee, et al., A graphene-based electrochemical device with thermoresponsive microneedles for diabetes monitoring and therapy, Nat. Nanotechnol., 2016, 11(6), 566–572 CrossRef CAS PubMed.
- M. Padash, C. Enz and S. Carrara, Microfluidics by additive manufacturing for wearable biosensors: A review, Sensors, 2020, 20(15), 4236 CrossRef CAS PubMed.
- S. Ma, et al., Ultra-Sensitive and Stable Multiplexed Biosensors Array in Fully Printed and Integrated Platforms for Reliable Perspiration Analysis, Adv. Mater., 2024, 2311106 CrossRef CAS PubMed.
- J. Min, et al., Wearable electrochemical biosensors in North America, Biosens. Bioelectron., 2021, 172, 112750 CrossRef CAS PubMed.
- Y. Yu, et al., Flexible electrochemical bioelectronics: the rise of in situ bioanalysis, Adv. mater., 2020, 32(15), 1902083 CrossRef CAS.
- I. Jeerapan and S. Poorahong, Flexible and stretchable electrochemical sensing systems: materials, energy sources, and integrations, J. Electrochem. Soc., 2020, 167(3), 037573 CrossRef CAS.
- G. Li and D. Wen, Wearable biochemical sensors for human health monitoring: sensing materials and manufacturing technologies, J. Mater. Chem. B, 2020, 8(16), 3423–3436 RSC.
- C. Legner, et al., Sweat sensing in the smart wearables era: Towards integrative, multifunctional and body-compliant perspiration analysis, Sens. Actuators, A, 2019, 296, 200–221 CrossRef CAS.
- E. Mirtaheri and C.-Z. Li, Wearable biomedical devices: state of the art, challenges, and future perspectives, Electrochem. Soc. Interface, 2019, 28(3), 71 CrossRef CAS.
- X. Wang, Z. Liu and T. Zhang, Flexible sensing electronics for wearable/attachable health monitoring, Small, 2017, 13(25), 1602790 CrossRef.
- G.-S. Liu, et al., Microneedles for transdermal diagnostics: Recent advances and new horizons, Biomaterials, 2020, 232, 119740 CrossRef CAS.
- J. Madden, et al., Biosensing in dermal interstitial fluid using microneedle based electrochemical devices, Sens. Bio-Sens. Res., 2020, 29, 100348 CrossRef.
- A. Dos Santos, et al., Transduction mechanisms, micro-structuring techniques, and applications of electronic skin pressure sensors: a review of recent advances, Sensors, 2020, 20(16), 4407 CrossRef CAS PubMed.
- P. French, In-vivo microsystems: a review, Sensors, 2020, 20(17), 4953 CrossRef CAS PubMed.
- E. V. Karpova and A. A. Karyakin, Noninvasive monitoring of diabetes and hypoxia by wearable flow-through biosensors, Curr. Opin. Electrochem., 2020, 23, 16–20 CrossRef CAS.
- E. V. Karpova, E. E. Karyakina and A. A. Karyakin, Wearable non-invasive monitors of diabetes and hypoxia through continuous analysis of sweat, Talanta, 2020, 215, 120922 CrossRef CAS PubMed.
- A. S. Campbell, J. Kim and J. Wang, Wearable electrochemical alcohol biosensors, Curr. Opin. Electrochem., 2018, 10, 126–135 CrossRef CAS.
- A. Depeursinge, et al., Fusing visual and clinical information for lung tissue classification in high-resolution computed tomography, Artif. Intell. Med., 2010, 50(1), 13–21 CrossRef.
- C. M. Silveira, T. Monteiro and M. G. J. B. Almeida, Biosensing with paper-based miniaturized printed electrodes–a modern trend, Biosensors, 2016, 6(4), 51 CrossRef PubMed.
- P. Lin, Organic electrochemical transistors integrated in flexible microfluidic systems and used for label-free DNA sensing, Adv. Mater., 2011, 35(23), 4035–4040 CrossRef PubMed.
- A. Yang, Fabric organic electrochemical transistors for biosensors, Adv. Mater., 2018, 30(23), 1800051 CrossRef.
- S. Takaloo and M. M. Zand, Wearable electrochemical flexible biosensors: With the focus on affinity biosensors, Sens. Bio-Sens. Res., 2021, 32, 100403 CrossRef.
- E. Ghafar-Zadeh, Wireless integrated biosensors for point-of-care diagnostic applications, Sensors, 2015, 15(2), 3236–3261 CrossRef CAS.
- J. L. Peters, et al., Ultrastructure at carbon fiber microelectrode implantation sites after acute voltammetric measurements in the striatum of anesthetized rats, J. Neurosci. Methods, 2004, 137(1), 9–23 CrossRef PubMed.
- J. F. Hernández-Rodríguez, D. Rojas and A. Escarpa, Electrochemical sensing directions for next-generation healthcare: trends, challenges, and frontiers, Anal. Chem., 2020, 93(1), 167–183 CrossRef PubMed.
- Y. Jiang, Research progress on portable electrochemical sensors for detection of mycotoxins in food and environmental samples, Chem. Eng. J., 2024, 149860 CrossRef CAS.
- D. D. La, A review on advances in graphene and porphyrin-based electrochemical sensors for pollutant detection, Trends Plant Sci., 2024, 100017 Search PubMed.
- S. Zhou, Wearable electrochemical sensors for plant small-molecule detection, Trends Plant Sci., 2024, 29(2), 219–231 CrossRef CAS.
- A. Parihar, Internet-of-Things-integrated Molecularly Imprinted Polymer-Based Electrochemical Nano-Sensors for Pesticide Detection in the Environment and Food Products, Environ. Pollut., 2024, 124029 CrossRef CAS.
- R. Wilson and A. Turner, Glucose oxidase: an ideal enzyme, Biosens. Bioelectron., 1992, 7(3), 165–185 CrossRef CAS.
- J. Wang, Electrochemical glucose biosensors, Chem. Rev., 2008, 108(2), 814–825 CrossRef CAS.
- K. E. Toghill and R. G. Compton, Electrochemical non-enzymatic glucose sensors: a perspective and an evaluation, Int. J. Electrochem. Sci., 2010, 5(9), 1246–1301 CrossRef CAS.
- R. Gifford, Continuous glucose monitoring: 40 Years, what we' ve learned and what's next, ChemPhysChem, 2013, 14(10), 2032–2044 CrossRef CAS.
- U. Hoss and E. S. Budiman, Factory-calibrated continuous glucose sensors: the science behind the technology, Diabetes Technol. Ther., 2017, 19(S2), S44–S50 CrossRef.
- A. Rossi, et al., Effective injury forecasting in soccer with GPS training data and machine learning, PLoS One, 2018, 13(7), e0201264 CrossRef.
- D. R. Seshadri, et al., Wearable devices for sports: new integrated technologies allow coaches, physicians, and trainers to better understand the physical demands of athletes in real time, IEEE pulse, 2017, 8(1), 38–43 Search PubMed.
- A. Koh, et al., A soft, wearable microfluidic device for the capture, storage, and colorimetric sensing of sweat, Sci. Transl. Med., 2016, 8(366), 366ra165 CrossRef PubMed.
- J. Choi, et al., Soft, skin-mounted microfluidic systems for measuring secretory fluidic pressures generated at the surface of the skin by eccrine sweat glands, Lab Chip, 2017, 17(15), 2572–2580 RSC.
- H. Y. Y. Nyein, et al., A wearable microfluidic sensing patch for dynamic sweat secretion analysis, ACS sens., 2018, 3(5), 944–952 CrossRef CAS PubMed.
- S. Garcia, et al., Wearable sensor system powered by a biofuel cell for detection of lactate levels in sweat, ECS J. Solid State Sci. Technol., 2016, 5(8), M3075 CrossRef CAS.
- L. S. Kumar, et al., Label free nano-aptasensor for interleukin-6 in protein-dilute bio fluids such as sweat, Anal. Methods, 2016, 8(17), 3440–3444 RSC.
- S. N. Cheuvront and R. W. Kenefick, Dehydration: physiology, assessment, and performance effects, Compr. Physiol., 2011, 4(1), 257–285 Search PubMed.
- Z. Sonner, et al., The microfluidics of the eccrine sweat gland, including biomarker partitioning, transport, and biosensing implications, Biomicrofluidics, 2015, 9(3) CrossRef CAS.
- P. Moran, et al., The influence of blood lactate sample site on exercise prescription, J. Strength Cond. Res., 2012, 26(2), 563–567 CrossRef.
- G. Chu, A wearable electrochemical sensor for the monitoring of neonicotinoid insecticides, salicylic acid and the pH in plant guttation, Chem. Eng. J., 2024, 155340 CrossRef CAS.
- P. C. Corsato, Electrochemical sensing at the fingertips: Wearable glove-based sensors for detection of 4-nitrophenol, picric acid and diazepam, Chemosphere, 2024, 363, 142771 CrossRef CAS PubMed.
- Q. Zhang, Smartphone-based plant-wearable microfluidic sensor with self driven electrolyte for in-situ detection of methyl parathion, Sens. Actuators, B, 2024, 418, 136254 CrossRef CAS.
- R. K. Mishra, et al., Detection of vapor-phase organophosphate threats using wearable conformable integrated epidermal and textile wireless biosensor systems, Biosens. Bioelectron., 2018, 101, 227–234 CrossRef CAS.
- Z. Wu, et al., Efficient nonenzymatic H2O2 biosensor based on ZIF-67 MOF derived Co nanoparticles embedded N-doped mesoporous carbon composites, Sens. Actuators, B, 2018, 276, 142–149 CrossRef CAS.
- R. K. Mishra, et al., Wearable flexible and stretchable glove biosensor for on-site detection of organophosphorus chemical threats, ACS sens., 2017, 2(4), 553–561 CrossRef CAS.
- E. J. Cone, et al., Sweat testing for heroin, cocaine, and metabolites, J. Anal. Toxicol., 1994, 18(6), 298–305 CrossRef CAS.
- A. J. Steckl and P. Ray, Stress biomarkers in biological fluids and their point-of-use detection, ACS sens., 2018, 3(10), 2025–2044 CrossRef CAS.
- G. Evtugyn and T. Hianik, Electrochemical DNA sensors and aptasensors based on electropolymerized materials and polyelectrolyte complexes, TrAC, Trends Anal. Chem., 2016, 79, 168–178 CrossRef CAS.
- X. Wei, et al., Fe3C-assisted single atomic Fe sites for sensitive electrochemical biosensing, Anal. Chem., 2021, 93(12), 5334–5342 CrossRef CAS PubMed.
- R. Holzer, W. Bloch and C. Brinkmann, Minimally invasive electrochemical patch-based sensor system for monitoring glucose and lactate in the human body—A survey-based analysis of the end-user’s perspective, Sensors, 2020, 20(20), 5761 CrossRef CAS PubMed.
- T. Arakawa, et al., Mouthguard biosensor with telemetry system for monitoring of saliva glucose: A novel cavitas sensor, Biosens. Bioelectron., 2016, 84, 106–111 CrossRef CAS PubMed.
- H. Yao, et al., A contact lens with integrated telecommunication circuit and sensors for wireless and continuous tear glucose monitoring, J. Micromech. Microeng., 2012, 22(7), 075007 CrossRef.
- J. Wei, et al., A multicomponent interconnected composite paper for triple-mode sensors and flexible micro-supercapacitors, J. Mater. Chem. A, 2020, 8(46), 24620–24634 RSC.
- E. Bihar, et al., A fully inkjet-printed disposable glucose sensor on paper, npj Flexible Electron., 2018, 2(1), 30 CrossRef.
- R. Karthick and F. Chen, Free-standing graphene paper for energy application: Progress and future scenarios, Carbon, 2019, 150, 292–310 CrossRef CAS.
- H. Zhou, et al., Amino acid protic ionic liquids: multifunctional carbon precursor for N/S codoped hierarchically porous carbon materials toward supercapacitive energy storage, ACS Sustainable Chem. Eng., 2019, 7(10), 9281–9290 CrossRef CAS.
- H. Zhou, et al., The preparation of porous carbon materials derived from bio-protic ionic liquid with application in flexible solid-state supercapacitors, J. Hazard. Mater., 2021, 402, 124023 CrossRef CAS PubMed.
- Y. Zhang, et al., Hierarchical porous carbon heterojunction flake arrays derived from metal organic frameworks and ionic liquid for H 2 O 2 electrochemical detection in cancer tissue, Nano Res., 2021, 14, 1335–1343 CrossRef CAS.
- Z. Ma, et al., A porous hollow fiber sensor for detection of cellular hydrogen peroxide release based on cell-in-lumen configuration, Sens. Actuators, B, 2020, 321, 128516 CrossRef CAS.
- T. Wang, et al., 3D nitrogen-doped carbon nanofoam arrays embedded with PdCu alloy nanoparticles: Assembling on flexible microelectrode for electrochemical detection in cancer cells, Anal. Chim. Acta, 2021, 1158, 338420 CrossRef CAS.
- D. Qi, et al., Stretchable electronics based on PDMS substrates, Adv. Mater., 2021, 33(6), 2003155 CrossRef CAS PubMed.
- Z. Zhang, et al., Carbon-sheathed MoS2 nanothorns epitaxially grown on CNTs: electrochemical application for highly stable and ultrafast lithium storage, Adv. Energy Mater., 2018, 8(7), 1700174 CrossRef.
- M. Zhou, et al., Single-atom Ni-N4 provides a robust cellular NO sensor, Nat. Commun., 2020, 11(1), 3188 CrossRef CAS.
- J. Li, et al., Stretchable and transparent electrochemical sensor based on nanostructured Au on carbon nanotube networks for real-time analysis of H2O2 release from cells, Anal. Chem., 2021, 93(17), 6723–6730 CrossRef CAS.
- V. Mani, et al., Real-time quantification of hydrogen peroxide production in living cells using NiCo2S4@ CoS2 heterostructure, Sens. Actuators, B, 2019, 287, 124–130 CrossRef CAS.
- B. Zhang, et al., Designing MOF nanoarchitectures for electrochemical water splitting, Adv. Mater., 2021, 33(17), 2006042 CrossRef CAS PubMed.
- L. Lu, et al., Nanochannel-confined graphene quantum dots for ultrasensitive electrochemical analysis of complex samples, ACS Nano, 2018, 12(12), 12673–12681 CrossRef CAS PubMed.
- Q. Xu, et al., Dual nanoenzyme modified microelectrode based on carbon fiber coated with AuPd alloy nanoparticles decorated graphene quantum dots assembly for electrochemical detection in clinic cancer samples, Biosens. Bioelectron., 2018, 107, 153–162 CrossRef CAS PubMed.
- Y. Zhang, et al., Flexible nanohybrid microelectrode based on carbon fiber wrapped by gold nanoparticles decorated nitrogen doped carbon nanotube arrays: In situ electrochemical detection in live cancer cells, Biosens. Bioelectron., 2018, 100, 453–461 CrossRef CAS.
- H. Yuan, et al., Hierarchical core–shell structure of 2D VS2@ VC@ N-doped carbon sheets decorated by ultrafine Pd nanoparticles: assembled in a 3D rosette-like array on carbon fiber microelectrode for electrochemical sensing, ACS Appl. Mater. Interfaces, 2020, 12(13), 15507–15516 CrossRef CAS PubMed.
- Y. Zhang, et al., Coral-like hierarchical structured carbon nanoscaffold with improved sensitivity for biomolecular detection in cancer tissue, Biosens. Bioelectron., 2020, 150, 111924 CrossRef CAS PubMed.
- A. Zhao, et al., Functionalized graphene fiber modified by dual nanoenzyme: Towards high-performance flexible nanohybrid microelectrode for electrochemical sensing in live cancer cells, Sens. Actuators, B, 2020, 310, 127861 CrossRef CAS.
- A. Zhao, et al., Green and controllable synthesis of multi-heteroatoms Co-doped graphene fiber as flexible and biocompatible microelectrode for in situ electrochemical detection of biological samples, Sens. Actuators, B, 2021, 335, 129683 CrossRef CAS.
- H.-J. Hsieh, et al., Shear-induced endothelial mechanotransduction: the interplay between reactive oxygen species (ROS) and nitric oxide (NO) and the pathophysiological implications, J. Biomed. Sci., 2014, 21, 1–15 CrossRef.
- W. Gao, et al., Promoting the electrocatalytic properties of nickel aerogel by gold decoration for efficient electrocatalytic oxygen evolution in alkali, Chem. Commun., 2020, 56(98), 15446–15449 RSC.
- R. Xing, et al., Rapid and sensitive electrochemical detection of myricetin based on polyoxometalates/SnO2/gold nanoparticles ternary nanocomposite film electrode, Sens. Actuators, B, 2019, 283, 35–41 CrossRef CAS.
- X. Zhao, et al., Fabrication of a flexible and stretchable nanostructured gold electrode using a facile ultraviolet-irradiation approach for the detection of nitric oxide released from cells, Anal. Chem., 2018, 90(12), 7158–7163 CrossRef CAS PubMed.
- F. Wu, et al., Single-atom Co–N4 electrocatalyst enabling four-electron oxygen reduction with enhanced hydrogen peroxide tolerance for selective sensing, J. Am. Chem. Soc., 2020, 142(39), 16861–16867 CrossRef CAS.
- J. Xi, et al., Synthesis strategies, catalytic applications, and performance regulation of single-atom catalysts, Adv. Funct. Mater., 2021, 31(12), 2008318 CrossRef CAS.
- T. Ozer, I. Agir and C. S. Henry, Low-cost Internet of Things (IoT)-enabled a wireless wearable device for detecting potassium ions at the point of care, Sens. Actuators, B, 2022, 365, 131961 CrossRef CAS.
- N. I. Hossain, A. Sharma and S. Sonkusale, A wearable and multiplexed electrochemical sensor suite for real-time sweat ionic content and pH monitoring with IoT integration, IEEE Sens. Lett., 2023 Search PubMed.
- P. Dwivedi and M. K. Singha, IoT based wearable healthcare system: post COVID-19. The Impact of the COVID-19 Pandemic on Green Societies, Environ. Sustainability, 2021, 305–321 Search PubMed.
- B. Li, et al., A wearable IoT aldehyde sensor for pediatric asthma research and management, Sens. Actuators, B, 2019, 287, 584–594 CrossRef CAS PubMed.
- X. Zhu, et al., Water splitting-assisted electrocatalytic oxidation of glucose with a metal–organic framework for wearable nonenzymatic perspiration sensing, Anal. Chem., 2019, 91(16), 10764–10771 CrossRef CAS PubMed.
- M. Fotouhi, A dual-mode assay kit using a portable potentiostat connected to a smartphone via Bluetooth communication and a potential-power angle-based paper device susceptible for low-cost point-of-care testing of iodide and dopamine, Anal. Chim. Acta, 2024, 1287, 342127 CrossRef CAS.
- Y. Bi, et al., Universal fully integrated wearable sensor arrays for the multiple electrolyte and metabolite monitoring in raw sweat, saliva, or urine, Anal. Chem., 2023, 95(16), 6690–6699 CrossRef CAS PubMed.
- S. K. Yadav, A. K. Yadav, A. Kaushik and P. Solanki, Functionalized Graphitic Carbon Nitride as an Efficient Electro-Analytical Platform for the Label-free Electrochemical Sensing of Interleukin-8 in Saliva Samples, Nanoscale, 2024 10.1039/D4NR02039A.
- Q. Zhao, et al., Printable nanophotonic devices via holographic laser ablation, ACS Nano, 2015, 9(9), 9062–9069 CrossRef CAS PubMed.
- S. Wang, et al., Vertically aligned conductive metal-organic framework nanowires array composite fiber as efficient solid-contact for wearable potentiometric sweat sensing, Sens. Actuators, B, 2022, 369, 132290 CrossRef CAS.
- J. Xiao, et al., An electrochemical wearable sensor for levodopa quantification in sweat based on a metal–Organic framework/graphene oxide composite with integrated enzymes, Sens. Actuators, B, 2022, 359, 131586 CrossRef CAS.
- H. Yao, J. S. Angela, C. Melissa, L. Ilkka and A. P. Babak, A contact lens with embedded sensor for monitoring tear glucose level, Biosens. Bioelectron., 2011, 26(7), 3290–3296 CrossRef CAS.
- Y. Zhao, et al., All-fiber structure covered with two-dimensional conductive MOF materials to construct a comfortable, breathable and high-quality self-powered wearable sensor system, J. Mater. Chem. A, 2022, 10(3), 1248–1256 RSC.
- L.-C. Tai, et al., Wearable sweat band for noninvasive levodopa monitoring, Nano lett., 2019, 19(9), 6346–6351 CrossRef CAS.
- Y. Yang, et al., A laser-engraved wearable sensor for sensitive detection of uric acid and tyrosine in sweat, Nat. Biotechnol., 2020, 38(2), 217–224 CrossRef CAS PubMed.
- B. Ciui, et al., Wearable wireless tyrosinase bandage and microneedle sensors: toward melanoma screening, Adv. Healthcare Mater., 2018, 7(7), 1701264 CrossRef PubMed.
- Y. Lei, et al., A MXene-based wearable biosensor system for high-performance in vitro perspiration analysis, Small, 2019, 15(19), 1901190 CrossRef.
- K.-C. Lin, S. Muthukumar and S. Prasad, Flex-GO (Flexible graphene oxide) sensor for electrochemical monitoring lactate in low-volume passive perspired human sweat, Talanta, 2020, 214, 120810 CrossRef CAS PubMed.
- N. Promphet, et al., Cotton thread-based wearable sensor for non-invasive simultaneous diagnosis of diabetes and kidney failure, Sens. Actuators, B, 2020, 321, 128549 CrossRef CAS.
- S. Y. Oh, et al., Skin-attachable, stretchable electrochemical sweat sensor for glucose and pH detection, ACS Appl. Mater. Interfaces, 2018, 10(16), 13729–13740 CrossRef CAS PubMed.
- A. Roda, et al., Smartphone-based biosensors: A critical review and perspectives, TrAC, Trends Anal. Chem., 2016, 79, 317–325 CrossRef CAS.
- E. V. Karpova, et al., Noninvasive diabetes monitoring through continuous analysis of sweat using flow-through glucose biosensor, Anal. Chem., 2019, 91(6), 3778–3783 CrossRef CAS.
- H. Wu, et al., Highly stable Ni-MOF comprising triphenylamine moieties as a high-performance redox indicator for sensitive aptasensor construction, Anal. Chim. Acta, 2019, 1049, 74–81 CrossRef CAS PubMed.
- B. Wang, et al., High-performance field-effect transistor glucose biosensors based on bimetallic Ni/Cu metal-organic frameworks, Biosens. Bioelectron., 2021, 171, 112736 CrossRef CAS.
- Y. Guo, et al., A wearable transient pressure sensor made with MXene nanosheets for sensitive broad-range human–machine interfacing, Nano lett., 2019, 19(2), 1143–1150 CrossRef CAS PubMed.
- M. Parrilla, et al., Wearable all-solid-state potentiometric microneedle patch for intradermal potassium detection, Anal. Chem., 2018, 91(2), 1578–1586 CrossRef PubMed.
- S. M. Mugo and J. Alberkant, Flexible molecularly imprinted electrochemical sensor for cortisol monitoring in sweat, Anal. Bioanal. Chem., 2020, 412, 1825–1833 CrossRef CAS.
- D. Maier, et al., Toward continuous monitoring of breath biochemistry: a paper-based wearable sensor for real-time hydrogen peroxide measurement in simulated breath, ACS sens., 2019, 4(11), 2945–2951 CrossRef CAS PubMed.
- Q. Jia, et al., Silver nanoparticle embedded polymer–zirconium-based metal–organic framework (polyUiO-66) for electrochemical biosensors of respiratory viruses, J. Mater. Chem. C, 2021, 9(40), 14190–14200 RSC.
- S. M. Sheta, et al., A novel HCV electrochemical biosensor based on a polyaniline@ Ni-MOF nanocomposite, Dalton Trans., 2020, 49(26), 8918–8926 RSC.
- N. Li, et al., Dual-aptamer-based voltammetric biosensor for the Mycobacterium tuberculosis antigen MPT64 by using a gold electrode modified with a peroxidase loaded composite consisting of gold nanoparticles and a Zr (IV)/terephthalate metal-organic framework, Microchim. Acta, 2018, 185, 1–7 CrossRef CAS PubMed.
- G. Dai, et al., Simultaneous electrochemical determination of nuc and mecA genes for identification of methicillin-resistant Staphylococcus aureus using N-doped porous carbon and DNA-modified MOF, Microchim. Acta, 2021, 188, 1–9 CrossRef PubMed.
- S. Panhwar, et al., Dual responsive disposable electrode for the enumeration of escherichia coli in whole blood, Electroanalysis, 2020, 32(10), 2244–2252 CrossRef CAS.
- A. Gupta, et al., Development of an advanced electrochemical biosensing platform for E. coli using hybrid metal-organic framework/polyaniline composite, Environ. Res., 2019, 171, 395–402 CrossRef CAS.
- W. Wang, et al., A universal signal-on electrochemical assay for rapid on-site quantitation of vibrio parahaemolyticus using aptamer modified magnetic metal–organic framework and phenylboronic acid-ferrocene co-immobilized nanolabel, Anal. Chim. Acta, 2020, 1133, 128–136 CrossRef CAS.
- X. Zhang, et al., A novel enzyme-free electrochemical biosensor for rapid detection of Pseudomonas aeruginosa based on high catalytic Cu-ZrMOF and conductive Super P, Biosens. Bioelectron., 2019, 142, 111486 CrossRef CAS PubMed.
- X. Zhou, et al., Glucose oxidase-initiated cascade catalysis for sensitive impedimetric aptasensor based on metal-organic frameworks functionalized with Pt nanoparticles and hemin/G-quadruplex as mimicking peroxidases, Biosens. Bioelectron., 2017, 98, 83–90 CrossRef CAS.
- L. Cui, et al., An electrochemical biosensor based on the enhanced quasi-reversible redox signal of prussian blue generated by self-sacrificial label of iron metal-organic framework, Biosens. Bioelectron., 2018, 122, 168–174 CrossRef CAS.
- Z. Hatami, et al., Application of metal-organic framework as redox probe in an electrochemical aptasensor for sensitive detection of MUC1, Biosens. Bioelectron., 2019, 141, 111433 CrossRef CAS PubMed.
- Y. Zhang, et al., Cascade amplification based on PEI-functionalized metal–organic framework supported gold nanoparticles/nitrogen–doped graphene quantum dots for amperometric biosensing applications, Electrochim. Acta, 2022, 405, 139803 CrossRef CAS.
- R. Qiu, et al., A novel enzyme-free biosensor based on porous core–shell metal organic frame nanocomposites modified electrode for highly sensitive detection of uric acid and dopamine, J. Biomed. Nanotechnol., 2019, 15(7), 1443–1453 CrossRef CAS.
- J. Li, et al., Innovative Integration of Phase-Change Microcapsules with Metal–Organic Frameworks into an Intelligent Biosensing System for Enhancing Dopamine Detection, ACS Appl. Mater. Interfaces, 2021, 13(35), 41753–41772 CrossRef CAS.
- M.-Q. Wang, et al., Carbon nanotubes implanted manganese-based MOFs for simultaneous detection of biomolecules in body fluids, Analyst, 2016, 141(4), 1279–1285 RSC.
- H. S. Choi, et al., Development of Co-hemin MOF/chitosan composite based biosensor for rapid detection of lactose, J. Taiwan Inst. Chem. Eng., 2020, 113, 1–7 CrossRef CAS.
- S. Biswas, et al., Label-free electrochemical immunosensor for ultrasensitive detection of carbohydrate antigen 125 based on antibody-immobilized biocompatible MOF-808/CNT, ACS Appl. Mater. Interfaces, 2021, 13(2), 3295–3302 CrossRef CAS.
- X. Fang, et al., Nanocomposites of Zr (IV)-based metal–organic frameworks and reduced graphene oxide for electrochemically sensing ciprofloxacin in water, ACS Appl. Nano Mater., 2019, 2(4), 2367–2376 CrossRef CAS.
- L.-C. Tai, et al., Wearable Sweat Band for Noninvasive Levodopa Monitoring, Nano Lett., 2019, 19(9), 6346–6351 CrossRef CAS PubMed.
- J. Xiao, et al., Hydrophilic metal-organic frameworks integrated uricase for wearable detection of sweat uric acid, Anal. Chim. Acta, 2022, 1208, 339843 CrossRef CAS PubMed.
- Y. Shu, et al., Highly stretchable wearable electrochemical sensor based on Ni-Co MOF nanosheet-decorated Ag/rGO/PU fiber for continuous sweat glucose detection, Anal. Chem., 2021, 93(48), 16222–16230 CrossRef CAS.
- L.-C. Tai, et al., Nicotine monitoring with a wearable sweat band, ACS sens., 2020, 5(6), 1831–1837 CrossRef CAS.
- R. Vinoth, et al., Fully printed wearable microfluidic devices for high-throughput sweat sampling and multiplexed electrochemical analysis, ACS sens., 2021, 6(3), 1174–1186 CrossRef CAS.
- Z. Zhou, et al., Luminescent wearable biosensors based on gold nanocluster networks for “turn-on” detection of Uric acid, glucose and alcohol in sweat, Biosens. Bioelectron., 2021, 192, 113530 CrossRef CAS PubMed.
- Z. Xu, et al., A conducting polymer PEDOT: PSS hydrogel based wearable sensor for accurate uric acid detection in human sweat, Sens. Actuators, B, 2021, 348, 130674 CrossRef CAS.
- M. Simsek, et al., Printable 3D carbon nanofiber networks with embedded metal nanocatalysts, ACS Appl. Mater. Interfaces, 2020, 12(35), 39533–39540 CrossRef CAS.
- L. Cao, et al., Nanoparticle-based 3D membrane for impedimetric biosensor applications, Bioelectrochemistry, 2020, 136, 107593 CrossRef CAS PubMed.
- C. Toyos-Rodríguez, F. J. García-Alonso and A. de la Escosura-Muñiz, Electrochemical biosensors based on nanomaterials for early detection of Alzheimer's disease, Sensors, 2020, 20(17), 4748 CrossRef.
- N. Wongkaew, et al., Functional nanomaterials and nanostructures enhancing electrochemical biosensors and lab-on-a-chip performances: recent progress, applications, and future perspective, Chem. Rev., 2018, 119(1), 120–194 CrossRef PubMed.
- N. Tripathy and D.-H. Kim, Metal oxide modified ZnO nanomaterials for biosensor applications, Nano convergence, 2018, 5(1), 27 CrossRef PubMed.
- G. Maduraiveeran, M. Sasidharan and V. Ganesan, Electrochemical sensor and biosensor platforms based on advanced nanomaterials for biological and biomedical applications, Biosens. Bioelectron., 2018, 103, 113–129 CrossRef CAS.
- I.-H. Cho, D. H. Kim and S. Park, Electrochemical biosensors: Perspective on functional nanomaterials for on-site analysis, Biomater. Res., 2020, 24(1), 6 CrossRef CAS.
- J. Li and N. Wu, Biosensors Based on Nanomaterials and Nanodevices, CRC Press, 2013 Search PubMed.
- J. M. George, A. Antony and B. Mathew, Metal oxide nanoparticles in electrochemical sensing and biosensing: a review, Microchim. Acta, 2018, 185, 1–26 CrossRef CAS.
- C. Ocaña and M. del Valle, Impedimetric aptasensors using nanomaterials, in Nanotechnology and biosensors, Elsevier, 2018, pp. 233–267 Search PubMed.
- Y. Dai, et al., Recent advances in nanomaterial-enhanced biosensing methods for hepatocellular carcinoma diagnosis, TrAC, Trends Anal. Chem., 2020, 130, 115965 CrossRef CAS.
- J. Xu, et al., Nanomaterials in electrochemical cytosensors, Analyst, 2020, 145(6), 2058–2069 RSC.
- J. Soleymani, et al., The role of nanomaterials on the cancer cells sensing based on folate receptor: analytical approach, TrAC, Trends Anal. Chem., 2020, 125, 115834 CrossRef CAS.
- C. A. Razzino, et al., An electrochemical immunosensor using gold nanoparticles-PAMAM-nanostructured screen-printed carbon electrodes for tau protein determination in plasma and brain tissues from Alzheimer patients, Biosens. Bioelectron., 2020, 163, 112238 CrossRef CAS PubMed.
- U. Badıllı, et al., Role of quantum dots in pharmaceutical and biomedical analysis, and its application in drug delivery, TrAC, Trends Anal. Chem., 2020, 131, 116013 CrossRef.
- M. K. Yazdi, et al., Nanotechnology-based biosensors in drug delivery, in Nanoengineered biomaterials for advanced drug delivery, Elsevier, 2020, pp. 767–779 Search PubMed.
- M. Ghalkhani, et al., Application of nanomaterials in development of electrochemical sensors and drug delivery systems for anticancer drugs and cancer biomarkers, Crit. Rev. Anal. Chem., 2022, 52(3), 481–503 CrossRef CAS PubMed.
- K. Nishimura, et al., Enhancement of the electron transfer rate in carbon nanotube flexible electrochemical sensors by surface functionalization, Electrochim. Acta, 2019, 295, 157–163 CrossRef CAS.
- T. Shu, et al., Stimuli-responsive polymer-based systems for diagnostic applications, J. Mater. Chem. B, 2020, 8(32), 7042–7061 RSC.
- R. Jain, N. Jadon and A. Pawaiya, Polypyrrole based next generation electrochemical sensors and biosensors: A review, TrAC, Trends Anal. Chem., 2017, 97, 363–373 CrossRef CAS.
- S. Shrivastava, N. Jadon and R. Jain, Next-generation polymer nanocomposite-based electrochemical sensors and biosensors: A review, TrAC, Trends Anal. Chem., 2016, 82, 55–67 CrossRef CAS.
- V. Svitkova and I. Palchetti, Functional polymers in photoelectrochemical biosensing, Bioelectrochemistry, 2020, 136, 107590 CrossRef CAS.
- M. Bilgi and E. Ayranci, Biosensor application of screen-printed carbon electrodes modified with nanomaterials and a conducting polymer: Ethanol biosensors based on alcohol dehydrogenase, Sens. Actuators, B, 2016, 237, 849–855 CrossRef CAS.
- I. C. Lopes, A. Zebda and P. Vadgama, New directions in membrane designs for biosensors, Curr. Opin. Electrochem., 2018, 12, 107–112 CrossRef CAS.
- V. Svitková, et al., Behaviour and detection of acridine-type DNA intercalators in urine using an electrochemical DNA-based biosensor with the protective polyvinyl alcohol membrane, J. Electroanal. Chem., 2018, 821, 87–91 CrossRef.
- F. Mazzara, et al., PANI-based wearable electrochemical sensor for pH sweat monitoring, Chemosensors, 2021, 9(7), 169 CrossRef CAS.
- V. Stepanova, et al., DNA-polylactide modified biosensor for electrochemical determination of the DNA-drugs and aptamer-aflatoxin M1 interactions, Sensors, 2019, 19(22), 4962 CrossRef CAS.
- G. Subashini and C. Nithya, Transition Metal Chalcogenide-Based Flexible and Wearable Sensors, in Flexible and Wearable Sensors, CRC Press, 2023, pp. 149–158 Search PubMed.
- H. Huang, Green Ink Formulation of Single-Crystalline Transition Metal Chalcogenides for Fully-Printed Strain-Insensitive Wearable Sensing Electronics, 2023 Search PubMed.
- R. Rakhi, et al., Novel amperometric glucose biosensor based on MXene nanocomposite, Sci. rep., 2016, 6(1), 36422 CrossRef CAS PubMed.
- A. J. Bandodkar, et al., Wearable sensors for biochemical sweat analysis, Annu. Rev. Anal. Chem., 2019, 12, 1–22 CrossRef.
- J. R. Sempionatto, et al., An epidermal patch for the simultaneous monitoring of haemodynamic and metabolic biomarkers, Nat. Biomed. Eng., 2021, 5(7), 737–748 CrossRef CAS PubMed.
- X. Xuan, H. S. Yoon and J. Y. Park, A wearable electrochemical glucose sensor based on simple and low-cost fabrication supported micro-patterned reduced graphene oxide nanocomposite electrode on flexible substrate, Biosens. Bioelectron., 2018, 109, 75–82 CrossRef CAS.
- S. Lin, et al., Natural perspiration sampling and in situ electrochemical analysis with hydrogel micropatches for user-identifiable and wireless chemo/biosensing, ACS sens., 2019, 5(1), 93–102 CrossRef PubMed.
- C. Cheng, et al., Battery-free, wireless, and flexible electrochemical patch for in situ analysis of sweat cortisol via near field communication, Biosens. Bioelectron., 2021, 172, 112782 CrossRef CAS.
- G. Li and D. Wen, Sensing nanomaterials of wearable glucose sensors, Chin. Chem. Lett., 2021, 32(1), 221–228 CrossRef CAS.
- X. Zhu, et al., Nonenzymatic wearable sensor for electrochemical analysis of perspiration glucose, ACS sens., 2018, 3(6), 1135–1141 CrossRef CAS PubMed.
- J. Yang, R. Wang, X. Guan, M. M. Hassan, A. Almogren and A. Alsanad, AI-enabled emotion-aware robot: The fusion of smart clothing, edge clouds and robotics, Future Gener. Comput. Syst., 2020, 102, 701–709 CrossRef.
- M. Chen, et al., Edge cognitive computing based smart healthcare system, Future Gener. Comput. Syst., 2018, 86, 403–411 CrossRef.
- O. Parlak, et al., Molecularly selective nanoporous membrane-based wearable organic electrochemical device for noninvasive cortisol sensing, Sci. Adv., 2018, 4(7), eaar2904 CrossRef CAS.
- H. Lee, et al., Wearable/disposable sweat-based glucose monitoring device with multistage transdermal drug delivery module, Sci. Adv., 2017, 3(3), e1601314 CrossRef PubMed.
- Y. J. Hong, et al., Multifunctional wearable system that integrates sweat-based sensing and vital-sign monitoring to estimate pre-/post-exercise glucose levels, Adv. Funct. Mater., 2018, 28(47), 1805754 CrossRef.
- K. Fan, et al., A lightweight authentication scheme for cloud-based RFID healthcare systems, IEEE Netw., 2019, 33(2), 44–49 Search PubMed.
- J. Lv, et al., Wearable biosupercapacitor: harvesting and storing energy from sweat, Adv. Funct. Mater., 2021, 31(38), 2102915 CrossRef CAS.
- Z. Yu, et al., Facile synthesis of NiCo2O4@Polyaniline core–shell nanocomposite for sensitive determination of glucose, Biosens. Bioelectron., 2016, 75, 161–165 CrossRef CAS PubMed.
- W. Chu, et al., Trifunctional of phosphorus-doped NiCo2O4 nanowire materials for asymmetric supercapacitor, oxygen evolution reaction, and hydrogen evolution reaction, ACS Appl. Mater. Interfaces, 2019, 12(2), 2763–2772 CrossRef.
- Y. Lu, et al., Wearable sweat monitoring system with integrated micro-supercapacitors, Nano Energy, 2019, 58, 624–632 CrossRef CAS.
- R. K. Mishra, et al., Simultaneous detection of salivary Δ9-tetrahydrocannabinol and alcohol using a Wearable Electrochemical Ring Sensor, Talanta, 2020, 211, 120757 CrossRef CAS.
- V. Katseli, A. Economou and C. Kokkinos, Smartphone-addressable 3D-printed electrochemical ring for nonenzymatic self-monitoring of glucose in human sweat, Anal. Chem., 2021, 93(7), 3331–3336 CrossRef CAS.
- L. Wang, et al., Weaving sensing fibers into electrochemical fabric for real-time health monitoring, Adv. Funct. Mater., 2018, 28(42), 1804456 CrossRef.
|
This journal is © The Royal Society of Chemistry 2024 |
Click here to see how this site uses Cookies. View our privacy policy here.