DOI:
10.1039/D4SC01650E
(Edge Article)
Chem. Sci., 2024,
15, 11500-11506
Crystalline organic monoliths with bicontinuous porosity†
Received
11th March 2024
, Accepted 18th June 2024
First published on 19th June 2024
Abstract
Organic crystals are a promising class of materials for various optical applications. However, it has been challenging to make macroscopic organic crystals with bicontinuous porosity that are applicable to flow chemistry. In this study, a new class of porous materials, cm-scale crystalline organic monoliths (COMs) with bicontinuous porosity, are synthesized by replicating the porous structure of silica monolith templates. The COMs composed of p-terphenyl can take up more than 30 wt% of an aqueous solution, and the photophysical properties of the p-terphenyl crystals are well maintained in the COMs. The relatively high surface area of the COMs can be exploited for efficient Dexter energy transfer from triplet sensitizers on the pore surface. The resulting triplet excitons in the COMs encounter and annihilate, generating upconverted UV emission. The COMs would open a new avenue toward applications of organic crystals in flow photoreaction systems.
Introduction
Organic crystals have attracted much attention for their intriguing luminescence functions such as solid-state fluorescence,1,2 photon upconversion,3–5 room-temperature phosphorescence,6–9 photochromism,10 and optical waveguides.11–14 While the size of organic crystals is typically in the range of μm to mm, macroscopic organic crystals on the cm-scale have also been exploited. Macroscopic crystals of polyaromatic hydrocarbons (PAHs) have been studied as organic scintillators.15–18 Acene-doped PAH single crystals have also been used for microwave amplification by stimulated emission of radiation (MASER)19–21 and dynamic nuclear polarization using photoexcited triplet electrons (triplet-DNP).22–25 These bulk crystals can be prepared by melting the components and subsequent cooling. On the other hand, these bulk crystals have only been used in “dry” systems, and their limited surface area made it difficult to interact with molecules in solution.
Here, we propose a new class of porous materials, crystalline organic monoliths (COMs). Monoliths with bicontinuous porous structures composed of silica and organic polymers have been used as adsorbents, supports, and chromatographic columns.26–34 While some photofunctional titania monoliths have been developed,35–37 examples of photofunctional organic monoliths have been limited.38 If bicontinuous porous monoliths can be created with organic crystals, the long exciton diffusion length in organic crystals would enable excitons to reach the pore surface to be utilized.39,40 This novel design of monoliths allows conventional bulk organic crystals to interact with molecules in solution effectively. We synthesized COMs using a template replication method. First, a melt of p-terphenyl (TP), a model compound, was introduced into the pores of template silica monoliths. A base treatment removed the template, leaving TP-based COMs (TP-COMs) with bicontinuous pore structures. The pore surface of the TP-COMs could be utilized for Dexter energy transfer. Upon visible light irradiation, surface-mediated triplet energy transfer from sensitizer molecules to the TP-COMs and subsequent triplet–triplet annihilation (TTA) resulted in upconverted UV emission from the TP-COMs. The visible-to-UV photon upconversion of the COM containing aqueous solutions shows their promising potential for applications in flow photochemistry.
Results and discussion
Synthesis of TP-based COMs (TP-COMs)
We used silica monoliths as templates because they can be easily removed by hydrolysis without dissolving organic crystals. Silica monolith is a monolithic gel with a hierarchical porous structure, and the pore structure can be tuned using synthetic conditions.27 Silica monoliths were synthesized following the previous report (Fig. 1a).27 Urea and poly(ethylene glycol) (MW: ∼10
000, PEG10
000) were dissolved in an aqueous acetic acid solution. After complete dissolution, tetramethoxysilane (TMOS) was added to the mixture and allowed to react in an ice bath. After stirring for 90 minutes, the resulting mixture was transferred to a water bath at 30 °C for gelation. The resulting gels were heated to 110 °C for 4 hours to modulate the mesoporous structure. After washing and drying, the resulting gels were calcined at 600 °C for 5 hours to yield white silica monoliths (Fig. 1c). Scanning electron microscopy (SEM) images after calcination showed the formation of bicontinuous macroporous structures with an average silica width of 0.73 ± 0.04 μm and pore sizes ranging from 1.0 to 3.3 μm (Fig. 1e). In addition, N2 adsorption analysis at 77 K showed that the Brunauer–Emmett–Teller (BET) surface area was 362 m2 g−1, and the mesopore size estimated from the Barrett–Joyner–Halenda (BJH) method was about 15.6 nm (Fig. S1†). The formation of mesopores is typical of silica monoliths synthesized by this method, confirming the successful synthesis of silica monoliths. When exposed to water, the silica monoliths exhibited 79.2 wt% of water uptake according to the thermogravimetric analysis (TGA) curves (Fig. 2a). Considering the density of silica gel (0.7 g cm−3) and water (1.0 g cm−3), the volume ratio of pores in the silica monoliths was calculated to be 72.7 vol%.
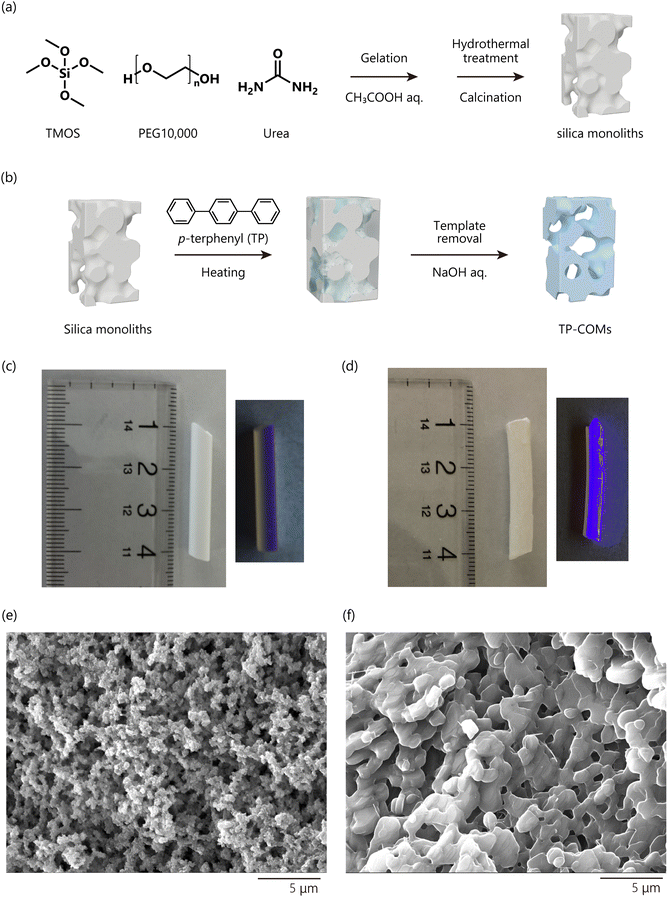 |
| Fig. 1 (a) Synthetic scheme of silica monoliths. TMOS: tetramethoxysilane and PEG10 000: poly(ethylene glycol) mw: 10 000. (b) Synthetic scheme of TP-COMs. Photographs without (left) and with (right) UV light irradiation of (c) silica monoliths and (d) TP-COMs. (e) SEM images of silica monoliths and (f) TP-COMs. | |
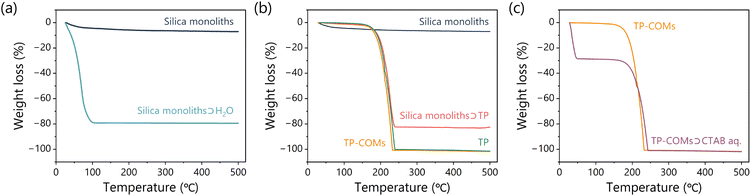 |
| Fig. 2 TGA curves of (a) silica monoliths (black) and silica monoliths exposed to water (blue), (b) silica monoliths (black), composite of silica monoliths and TP (red), TP powder (green), and TP-COMs (orange), (c) TP-COMs (orange) and TP-COMs immersed in the 10 mM aqueous CTAB solution (purple). The same color data represent the same sample. | |
The porous structure of silica monoliths has been transferred to organic crystals (Fig. 1b). In this work, p-terphenyl (TP) was chosen as a model compound to synthesize crystalline monoliths because TP has significant thermal stability and photoluminescence. A glass tube filled with the silica monoliths and TP powder was frame-sealed under reduced pressure. The sample was heated to 230 °C to introduce the molten TP into the pores of the silica monoliths. After cooling down to room temperature, excess TP was removed (see details in the ESI†). The resulting composite was immersed in a 1 M NaOH aqueous solution to dissolve the silica by hydrolysis. The resulting sample was washed with water and dried under reduced pressure to obtain TP-based crystalline organic monoliths (TP-COMs). While the original silica monoliths showed no luminescence under UV light irradiation (Fig. 1c), the obtained TP-COMs showed bright purple luminescence (Fig. 1d).
The introduction of TP into the silica pores and the removal of silica were tracked by TGA (Fig. 2b). The introduction of TP into the silica monoliths resulted in an 82.5% weight loss, which corresponds to 73.3 vol% of TP in silica monoliths (density of TP was estimated to be 1.2 g cm−3 based on the single crystal structure41). This value agrees well with the estimated porosity of water-filled silica monoliths (72.7 vol%), indicating that the pores of the silica monoliths were filled with TP. Furthermore, the TGA curve of TP-COMs reached 100% weight loss at around 230 °C, confirming the complete removal of the silica templates.
The porous structure of the TP-COMs was also investigated. SEM images of the TP-COMs showed a bicontinuous porous structure with an average size of pore walls and pore sizes of 3.0 ± 0.17 μm and 1.8 ± 0.14 μm, respectively, indicating the successful replication of the template silica monoliths (Fig. 1f). Since the pore surface of TP-COMs was too hydrophobic to take up water, an aqueous solution of 10 mM cetyltrimethylammonium bromide (CTAB) surfactant was introduced into the TP-COMs. The volume ratio of the aqueous solution in the TP-COM was estimated to be 32.8 vol% from the TGA curve (Fig. 2C). The volume ratio of TP to the silica monolith template (73.3 vol%) predicts the pore volume ratio of the TP-COMs to be 26.7 vol%. This predicted value is not far from the observed value (32.8 vol%), indicating the successful transfer of the porous structure from silica monoliths to TP-COMs. On the other hand, the N2 adsorption analysis showed less than 1.0 m2 g−1 of BET surface area, indicating that TP-COMs have a macroporous structure but are nonporous at the nanoscale (Fig. S1†). The current method successfully replicated the macropores but not the mesopores of the silica template, so further developments are needed to improve the surface area of the resulting COMs. The powder X-ray diffraction measurements supported the fact that the crystalline structure of TP is well preserved in the resulting TP-COMs (Fig. S3†). This simple melt crystallization and template removal method successfully synthesized the organic crystalline monoliths.
The morphology of the TP-COMs can be easily tuned by changing the porous structure of the silica monolith templates. By using different amounts of PEG10
000, the 3 types of silica monoliths were synthesized. Using these templates, corresponding porous structures of TP-COMs were successfully obtained (Fig. S4†). This result indicates that this method has excellent versatility in synthesizing COMs with different porous structures. The following demonstrations utilized TP-COMs synthesized with silica monolith using 575 mg of PEG10
000.
The optical properties of TP-COMs were evaluated. Although UV-vis absorption spectra could not be recorded due to the strong scattering, excitation and fluorescence spectra showed a similar spectral shape to TP powder (Fig. S5†). In addition, the TP powder shows a high fluorescence quantum yield (66.5%), which is mostly preserved in the TP-COMs (57.0%). This indicates that TP-COMs can be a promising UV-emitting bulk material with high porosity.
Dexter energy transfer at the pore surface of COMs
The triplet Dexter energy transfer at the pore surface of the TP-COMs has been investigated. The organic crystals of π-conjugated compounds with high porosity have the potential to harvest and convert light energy for various photochemical reactions. Here, we used the TP-COMs as triplet energy acceptors to demonstrate photon upconversion based on triplet–triplet annihilation (TTA-UC). As a triplet sensitizer, we chose Ir(ppy)3, which has a suitable T1 energy level (T1 = 2.48 eV)42 for efficient triplet energy transfer to TP crystals (T1 = 2.15 eV).43
Dexter energy transfer requires close contact between Ir(ppy)3 and TP molecules. Since TP is soluble in common organic solvents, the monolithic structure of TP-COMs is easily lost by organic solvents. On the other hand, hydrophobic Ir(ppy)3 is not soluble in water. To overcome these problems, we used an aqueous micelle solution to modify Ir(ppy)3 on the pore surface of TP-COMs (Fig. 3a). The incorporation of Ir(ppy)3 into the micelle allowed Ir(ppy)3 to dissolve in water. The use of surfactants also allowed the aqueous solution to be introduced into the highly hydrophobic pores by reducing the surface tension. A THF solution of Ir(ppy)3 was added to the aqueous solution of the surfactant CTAB, and stirred overnight, resulting in the Ir(ppy)3-dispersed micelle solution. After syringe filtration, the formation of Ir(ppy)3-dispersed micelles was confirmed by dynamic light scattering (DLS) and zeta potential measurements. An average micelle size of 3.21 ± 0.43 nm (Fig. 3b) and a zeta potential of +60.7 ± 1.5 mV were observed, indicating the formation of the CTAB micelles. Absorption and photoluminescence spectra of the micelle solution confirmed the inclusion of Ir(ppy)3 (Fig. 3c), and the concentration of Ir(ppy)3 was roughly estimated to be 9.3 μM from the absorbance of the aqueous micelle and the molar absorption coefficient in CH2Cl2 (ε377 = 12
000 M−1 cm−1) (Fig. S6†).44
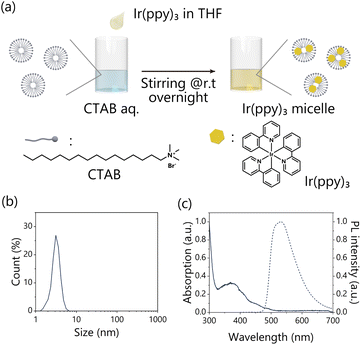 |
| Fig. 3 (a) Schematic representation of Ir(ppy)3 micelle preparation and chemical structures of CTAB and Ir(ppy)3. (b) DLS profile of Ir(ppy)3 micelles. (c) UV-vis absorption (solid line) and photoluminescence (dotted line) spectra of Ir(ppy)3 micelles in water. | |
To modify the macropore surface of the TP-COMs with Ir(ppy)3, the TP-COMs were immersed in the Ir(ppy)3 micellar solution. After 2 hours of immersion, the resulting sample was washed with water and dried under reduced pressure. The macropore structure of the TP-COMs was well preserved after the Ir(ppy)3 modification (Fig. S7†). The modification of Ir(ppy)3 was confirmed by photoluminescence spectra (Fig. S8†). Notably, the similar photoluminescence spectra of Ir(ppy)3 on the TP-COMs compared to those in the toluene solution indicated the good dispersibility of Ir(ppy)3 on the surface of TP-COMs (Fig. S8†). On the other hand, the amount of Ir(ppy)3 on the surface of the TP-COMs could not be determined due to its low concentration.
The TET process was evaluated by monitoring the change in the photoluminescence lifetime of Ir(ppy)3 (Fig. 4a). The photoluminescence lifetime of Ir(ppy)3 on the pore surface of the TP-COMs became significantly shorter than that in the toluene solution (Fig. 4b). The photoluminescence decay of Ir(ppy)3 on the TP-COMs could be fitted with a biexponential function. The mean photoluminescence lifetime was 0.38 μs (Fig. 4b, Table S1†). The TET efficiency was calculated using the following equation:
where
ΦET![[thin space (1/6-em)]](https://www.rsc.org/images/entities/char_2009.gif)
is the energy transfer efficiency,
τ is the average photoluminescence lifetime of Ir(ppy)
3 on the surface of the TP-COMs, and
τ0 is the lifetime of Ir(ppy)
3 in toluene (1.30 μs). The
ΦET was estimated to be 70.6%, demonstrating efficient Dexter energy transfer at the pore surface of the TP-COMs.
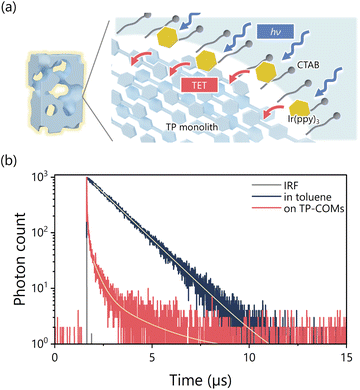 |
| Fig. 4 (a) Schematic representation of triplet Dexter energy transfer on the macropore surface of TP-COMs. (b) Photoluminescence decays and fitted lines (yellow) of Ir(ppy)3 in toluene (black) and on the surface of TP-COMs (red). The instrument response function (IRF) is shown in the gray line. | |
TTA-UC of Ir(ppy)3-modified TP-COMs
Finally, the TTA-UC properties of Ir(ppy)3 modified TP-COMs were evaluated. TTA-UC is one of the photon upconversion methods that can generate a higher energy photon from two lower energy photons.45–58 The photo-excited sensitizer undergoes intersystem crossing (ISC) to the triplet state. The triplet energy of the sensitizer is then transferred to the emitter. When two triplets of the emitter encounter and annihilate, the upconverted singlet state of the emitter is generated (Fig. 5a). TTA-UC is promising because it can work at low excitation light intensity and yield high quantum efficiency, which is expected for various applications in energy and biology disciplines.59–64
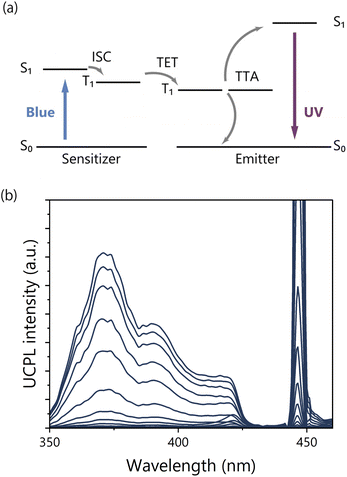 |
| Fig. 5 (a) Schematic representation of TTA-UC. ISC: intersystem crossing, TET: triplet energy transfer, and TTA: triplet–triplet annihilation. (b) Upconversion photoluminescence (UCPL) spectra of Ir(ppy)3-modified TP-COMs at different excitation intensities (λex = 445 nm, 425 nm short pass filter). | |
The TTA-UC measurements were performed under an Ar atmosphere for Ir(ppy)3 modified TP-COMs. Irradiation with a 445 nm continuous wave (CW) blue laser resulted in UC emission in the UV region with a peak top at 372 nm (Fig. 5b). The μs scale of the upconversion photoluminescence (UCPL) decay indicated that the triplet states were involved, and the triplet lifetime was estimated to be 133 μs (Fig. S9†). No UCPL was observed when the TP-COMs without Ir(ppy)3 were excited using the 445 nm laser, supporting the sensitized TTA-UC mechanism. The TTA-UC efficiency could not be determined due to the low absorption of Ir(ppy)3 on the surface of the TP-COMs and the intense light scattering of the TP-COMs. Interestingly, no UCPL was detected by photoexcitation of Ir(ppy)3-doped TP powder prepared by melting and cooling Ir(ppy)3-modified TP-COMs. This indicates that when Ir(ppy)3 is uniformly dispersed throughout the TP powder, almost no TTA is observed due to the low concentration of Ir(ppy)3 and, thus, the low concentration of triplet excitons. On the other hand, by selectively modifying Ir(ppy)3 on the pore surface of TP-COMs, the local triplet density increases and TTA is effectively induced. This means that excitons can accumulate near the pore surface of the COMs and are expected to be applied to chemical reactions involving energy transfer and electron transfer using upconverted energy.
At low excitation intensities, TTA-UC behaves as a two-photon process because triplet deactivation occurs faster than TTA, but as the excitation light intensity is increased, triplet consumption by TTA becomes dominant, and the process behaves as a pseudo-one-photon process.65–67 The threshold excitation intensity, Ith, is the point at which this quadratic to linear transition occurs and is estimated from the intersection of the fitting lines in the double logarithmic plot of UC emission intensity versus excitation light intensity (Fig. 6). Ith is a key parameter for evaluating TTA-UC because approximately half of the maximum UC efficiency of the system is obtained at Ith. The UC emission intensity at 372 nm of the Ir(ppy)3-modified TP-COMs showed the expected quadratic-to-linear transition and a relatively low Ith of 750 mW cm−2 (Fig. 6). Notably, a similar Ith value of 1040 mW cm−2 was observed for the Ir(ppy)3-modified TP-COMs immersed in degassed water for 2 h (Fig. 6). This indicates that the leakage of Ir(ppy)3 into water is suppressed and that the upconverted energy can be utilized under aqueous conditions. An important step toward the possible development of a flow photoreaction system based on upconverting monoliths with bicontinuous porous structures has been demonstrated.
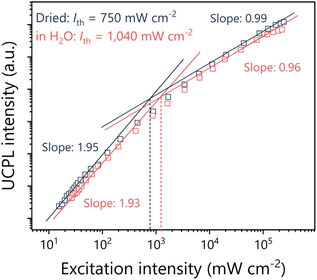 |
| Fig. 6 Excitation intensity dependence of UCPL intensity at 372 nm of Ir(ppy)3 modified TP-COMs under Ar (black) and in degassed water (red). | |
Conclusions
We have developed a method to impart bicontinuous porous structures to bulk organic crystals. Although examples of bicontinuous porous monoliths have been limited to amorphous materials, we have shown that the porous structure can be transferred to organic crystals using silica monoliths as templates. Furthermore, by modifying the monolith surface with a triplet sensitizer, we have succeeded in inducing highly efficient Dexter energy transfer and TTA-UC at relatively low excitation light intensities. The surface-mediated upconversion from visible light to UV light would lead to the flow of photochemical reaction systems. The method developed in this study is very simple and can be applied to various organic crystals. It will create a new class of materials called crystalline organic monoliths (COMs) with different optical and electronic functions.
Data availability
Data for this article are available at Zenodo at https://zenodo.org/doi/10.5281/zenodo.11512823.
Author contributions
N. Y. conceived the project. N. M. and S. N. carried out the experiments. K. M., K. K., and K. N. contributed to preparing the silica monolith templates. N. M. and N. Y. wrote the manuscript with the input of all co-authors.
Conflicts of interest
There are no conflicts to declare.
Acknowledgements
This work was partly supported by JST START (JPMJSF2303), JSPS KAKENHI (grant numbers JP23H00304, JP22K19051, and JP23KJ1715), the Murata Science Foundation, the Research Foundation for Opto-Science and Technology, the Kyushu University Platform of Inter-/Transdisciplinary Energy Research (Q-PIT) through its “Module-Research Program”, and the Kyushu University Integrated Initiative for Designing Future Society.
Notes and references
- H. Y. Zhang, Z. L. Zhang, K. Q. Ye, J. Y. Zhang and Y. Wang, Adv. Mater., 2006, 18, 2369–2372 CrossRef CAS.
- Q. Di, L. Li, X. Miao, L. Lan, X. Yu, B. Liu, Y. Yi, P. Naumov and H. Zhang, Nat. Commun., 2022, 13, 5280 CrossRef CAS PubMed.
- T. Ogawa, N. Yanai, S. Fujiwara, T.-Q. Nguyen and N. Kimizuka, J. Mater. Chem. C, 2018, 6, 5609–5615 RSC.
- C. D. Cruz, H. H. Choi, V. Podzorov, E. L. Chronister and C. J. Bardeen, J. Phys. Chem. C, 2018, 122, 17632–17642 CrossRef CAS.
- R. Enomoto, M. Hoshi, H. Oyama, H. Agata, S. Kurokawa, H. Kuma, H. Uekusa and Y. Murakami, Mater. Horiz., 2021, 8, 3449–3456 RSC.
- W. Z. Yuan, X. Y. Shen, H. Zhao, J. W. Y. Lam, L. Tang, P. Lu, C. Wang, Y. Liu, Z. Wang, Q. Zheng, J. Z. Sun, Y. Ma and B. Z. Tang, J. Phys. Chem. C, 2010, 114, 6090–6099 CrossRef CAS.
- O. Bolton, K. Lee, H.-J. Kim, K. Y. Lin and J. Kim, Nat. Chem., 2011, 3, 205–210 CrossRef CAS PubMed.
- W. Zhao, Z. He and B. Z. Tang, Nat. Rev. Mater., 2020, 5, 869–885 CrossRef CAS.
- M. Singh, K. Liu, S. Qu, H. Ma, H. Shi, Z. An and W. Huang, Adv. Opt. Mater., 2021, 9, 2002197 CrossRef CAS.
- K. Amimoto and T. Kawato, J. Photochem. Photobiol., C, 2005, 6, 207–226 CrossRef CAS.
- C. Zhang, Y. Yan, Y. S. Zhao and J. Yao, Acc. Chem. Res., 2014, 47, 3448–3458 CrossRef CAS PubMed.
- Y. Ma, Y. Zong, H. Yin, H. Lin, S. Chen and X.-D. Wang, Adv. Opt. Mater., 2021, 9, 2101481 CrossRef CAS.
- S. Wu, B. Zhou and D. Yan, Adv. Opt. Mater., 2021, 9, 2001768 CrossRef CAS.
- D. Tian and Y. Chen, Adv. Opt. Mater., 2021, 9, 2002264 CrossRef CAS.
- R. C. Sangster and J. W. Irvine Jr, J. Chem. Phys., 1956, 24, 670–715 CrossRef CAS.
- F. D. Brooks, Nucl. Instrum. Methods, 1979, 162, 477–505 CrossRef CAS.
- S. V. Budakovsky, N. Z. Galunov, N. L. Karavaeva, J. K. Kim, Y. K. Kim, O. A. Tarasenko and E. V. Martynenko, IEEE Trans. Nucl. Sci., 2007, 54, 2734–2740 CAS.
- N. Galunov, D. Gryn, N. Karavaeva, I. Khromiuk, I. Lazarev, O. Navozenko, A. Naumenko, O. Tarasenko and V. Yashchuk, J. Lumin., 2020, 226, 117477 CrossRef CAS.
- M. Oxborrow, J. D. Breeze and N. M. Alford, Nature, 2012, 488, 353–356 CrossRef CAS PubMed.
- Q. Ai, P. Chen, Y. Feng and Y. Xu, Cryst. Growth Des., 2017, 17, 2473–2477 CrossRef CAS.
- S. Cui, Y. Liu, G. Li, Q. Han, C. Ge, L. Zhang, Q. Guo, X. Ye and X. Tao, Cryst. Growth Des., 2020, 20, 783–792 CrossRef CAS.
- A. Henstra, T.-S. Lin, J. Schmidt and W. T. Wenckebach, Chem. Phys. Lett., 1990, 165, 6–10 CrossRef CAS.
- M. Iinuma, Y. Takahashi, I. Shaké, M. Oda, H. M. Shimizu, A. Masaike and T. Yabuzaki, J. Phys. Soc. Jpn., 2005, 74, 2622–2630 CrossRef CAS.
- K. Tateishi, M. Negoro, S. Nishida, A. Kagawa, Y. Morita and M. Kitagawa, Proc. Natl. Acad. Sci. U. S. A., 2014, 111, 7527–7530 CrossRef CAS PubMed.
- T. R. Eichhorn, A. J. Parker, F. Josten, C. Müller, J. Scheuer, J. M. Steiner, M. Gierse, J. Handwerker, M. Keim, S. Lucas, M. U. Qureshi, A. Marshall, A. Salhov, Y. Quan, J. Binder, K. D. Jahnke, P. Neumann, S. Knecht, J. W. Blanchard, M. B. Plenio, F. Jelezko, L. Emsley, C. C. Vassiliou, P. Hautle and I. Schwartz, J. Am. Chem. Soc., 2022, 144, 2511–2519 CrossRef CAS PubMed.
- E. C. Peters, F. Svec and J. M. J. Fréchet, Adv. Mater., 1999, 11, 1169–1181 CrossRef CAS.
- K. Nakanishi, H. Shikata, N. Ishizuka, N. Koheiya and N. Soga, J. High Resolut. Chromatogr., 2000, 23, 106–110 CrossRef CAS.
- J. O. Krause, S. Lubbad, O. Nuyken and M. R. Buchmeiser, Adv. Synth. Catal., 2003, 345, 996–1004 CrossRef CAS.
- K. Cabrera, J. Sep. Sci., 2004, 27, 843–852 CrossRef CAS PubMed.
- F. Svec, J. Sep. Sci., 2004, 27, 747–766 CrossRef CAS PubMed.
- K. Nakanishi and N. Tanaka, Acc. Chem. Res., 2007, 40, 863–873 CrossRef CAS PubMed.
- A. Sachse, A. Galarneau, F. Fajula, F. Di Renzo, P. Creux and B. Coq, Microporous Mesoporous Mater., 2011, 140, 58–68 CrossRef CAS.
- R. Porta, M. Benaglia and A. Puglisi, Org. Process Res. Dev., 2016, 20, 2–25 CrossRef CAS.
- C.-H. Pélisson, T. Nakanishi, Y. Zhu, K. Morisato, T. Kamei, A. Maeno, H. Kaji, S. Muroyama, M. Tafu, K. Kanamori, T. Shimada and K. Nakanishi, ACS Appl. Mater. Interfaces, 2017, 9, 406–412 CrossRef PubMed.
- X. Fan, H. Fei, D. H. Demaree, D. P. Brennan, J. M. St. John and S. R. J. Oliver, Langmuir, 2009, 25, 5835–5839 CrossRef CAS PubMed.
- O. Ruzimuradov, S. Nurmanov, M. Hojamberdiev, R. M. Prasad, A. Gurlo, J. Broetz, K. Nakanishi and R. Riedel, J. Eur. Ceram. Soc., 2014, 34, 809–816 CrossRef CAS.
- N. M. Nursam, X. Wang, J. Z. Y. Tan and R. A. Caruso, ACS Appl. Mater. Interfaces, 2016, 8, 17194–17204 CrossRef CAS PubMed.
- S. Ghasimi, S. Prescher, Z. J. Wang, K. Landfester, J. Yuan and K. A. I. Zhang, Angew. Chem., Int. Ed., 2015, 54, 14549–14553 CrossRef CAS PubMed.
- G. M. Akselrod, P. B. Deotare, N. J. Thompson, J. Lee, W. A. Tisdale, M. A. Baldo, V. M. Menon and V. Bulović, Nat. Commun., 2014, 5, 3646 CrossRef CAS PubMed.
- O. V. Mikhnenko, P. W. M. Blom and T.-Q. Nguyen, Energy Environ. Sci., 2015, 8, 1867–1888 RSC.
- H. M. Rietveld, E. N. Maslen and C. J. B. Clews, Acta Crystallogr., Sect. B: Struct. Sci., Cryst. Eng. Mater., 1970, 26, 693–706 CrossRef CAS.
- W. Zhao and F. N. Castellano, J. Phys. Chem. A, 2006, 110, 11440–11445 CrossRef CAS PubMed.
- D. M. Niedzwiedzki, D. Mulrow and L. G. Sobotka, J. Phys. Chem. A, 2022, 126, 5273–5282 CrossRef CAS PubMed.
- A. B. Tamayo, B. D. Alleyne, P. I. Djurovich, S. Lamansky, I. Tsyba, N. N. Ho, R. Bau and M. E. Thompson, J. Am. Chem. Soc., 2003, 125, 7377–7387 CrossRef CAS PubMed.
- S. Baluschev, T. Miteva, V. Yakutkin, G. Nelles, A. Yasuda and G. Wegner, Phys. Rev. Lett., 2006, 97, 143903 CrossRef CAS PubMed.
- T. N. Singh-Rachford and F. N. Castellano, Coord. Chem. Rev., 2010, 254, 2560–2573 CrossRef CAS.
- J. Zhao, S. Ji and H. Guo, RSC Adv., 2011, 1, 937–950 RSC.
- J.-H. Kim and J.-H. Kim, J. Am. Chem. Soc., 2012, 134, 17478–17481 CrossRef CAS PubMed.
- Y. C. Simon and C. Weder, J. Mater. Chem., 2012, 22, 20817–20830 RSC.
- V. Gray, D. Dzebo, M. Abrahamsson, B. Albinsson and K. Moth-Poulsen, Phys. Chem. Chem. Phys., 2014, 16, 10345–10352 RSC.
- M. Wu, D. N. Congreve, M. W. B. Wilson, J. Jean, N. Geva, M. Welborn, T. Van Voorhis, V. Bulović, M. G. Bawendi and M. A. Baldo, Nat. Photonics, 2016, 10, 31–34 CrossRef CAS.
- S. Wieghold, A. S. Bieber, Z. A. VanOrman, L. Daley, M. Leger, J.-P. Correa-Baena and L. Nienhaus, Matter, 2019, 1, 705–719 CrossRef CAS.
- P. Bharmoria, H. Bildirir and K. Moth-Poulsen, Chem. Soc. Rev., 2020, 49, 6529–6554 RSC.
- J. Alves, J. Feng, L. Nienhaus and T. W. Schmidt, J. Mater. Chem. C, 2022, 10, 7783–7798 RSC.
- A. Ronchi and A. Monguzzi, Chem. Phys. Rev., 2022, 3, 041301 CrossRef CAS.
- M. Uji, T. J. B. Zähringer, C. Kerzig and N. Yanai, Angew. Chem., Int. Ed., 2023, 62, e202301506 CrossRef CAS PubMed.
- K. Wang, R. P. Cline, J. Schwan, J. M. Strain, S. T. Roberts, L. Mangolini, J. D. Eaves and M. L. Tang, Nat. Chem., 2023, 15, 1172–1178 CrossRef CAS PubMed.
- T. Mizokuro, A. Abulikemu, K. Suzuki, Y. Sakagami, R. Nishii, T. Jin and K. Kamada, Phys. Chem. Chem. Phys., 2020, 22, 17807–17813 RSC.
- B. D. Ravetz, A. B. Pun, E. M. Churchill, D. N. Congreve, T. Rovis and L. M. Campos, Nature, 2019, 565, 343–346 CrossRef CAS PubMed.
- Y. Sasaki, M. Oshikawa, P. Bharmoria, H. Kouno, A. Hayashi-Takagi, M. Sato, I. Ajioka, N. Yanai and N. Kimizuka, Angew. Chem., Int. Ed., 2019, 58, 17827–17833 CrossRef CAS PubMed.
- B. Pfund, D. M. Steffen, M. R. Schreier, M.-S. Bertrams, C. Ye, K. Börjesson, O. S. Wenger and C. Kerzig, J. Am. Chem. Soc., 2020, 142, 10468–10476 CrossRef CAS PubMed.
- L. Huang, T. Le, K. Huang and G. Han, Nat. Commun., 2021, 12, 1898 CrossRef PubMed.
- S. N. Sanders, T. H. Schloemer, M. K. Gangishetty, D. Anderson, M. Seitz, A. O. Gallegos, R. C. Stokes and D. N. Congreve, Nature, 2022, 604, 474–478 CrossRef CAS PubMed.
- D. K. Limberg, J.-H. Kang and R. C. Hayward, J. Am. Chem. Soc., 2022, 144, 5226–5232 CrossRef CAS PubMed.
- A. Monguzzi, J. Mezyk, F. Scotognella, R. Tubino and F. Meinardi, Phys. Rev. B: Condens. Matter Mater. Phys., 2008, 78, 195112 CrossRef.
- Y. Y. Cheng, T. Khoury, R. G. C. R. Clady, M. J. Y. Tayebjee, N. J. Ekins-Daukes, M. J. Crossley and T. W. Schmidt, Phys. Chem. Chem. Phys., 2010, 12, 66–71 RSC.
- A. Haefele, J. Blumhoff, R. S. Khnayzer and F. N. Castellano, J. Phys. Chem. Lett., 2012, 3, 299–303 CrossRef CAS.
|
This journal is © The Royal Society of Chemistry 2024 |
Click here to see how this site uses Cookies. View our privacy policy here.