DOI:
10.1039/D4SC03029J
(Edge Article)
Chem. Sci., 2024,
15, 17937-17943
Crystal engineering of a new platform of hybrid ultramicroporous materials and their C2H2/CO2 separation properties†
Received
8th May 2024
, Accepted 26th September 2024
First published on 27th September 2024
Abstract
Hybrid ultramicroporous materials (HUMs) comprised of combinations of organic and inorganic linker ligands are a leading class of physisorbents for trace separations involving C1, C2 and C3 gases. First generation HUMs are modular in nature since they can be self-assembled from transition metal cations, ditopic linkers and inorganic “pillars”, as exemplified by the prototypal variant, SIFSIX-3-Zn (3 = pyrazine, SIFSIX = SiF62−). Conversely, HUMs that utilise chelating ligands such as ethylenediamine derivatives are yet to be explored as sorbents. Herein, we report the structures and sorption properties of two HUMs based upon the chelating ligand N1,N2-bis(pyridin-4-ylmethyl)ethane-1,2-diamine (enmepy), [Zn(enmepy)(SiF6)]n (SIFSIX-24-Zn) and [Zn(enmepy)(SO4)]n (SOFOUR-2-Zn). These HUMs are isostructural and exhibit high C2H2 uptakes of 85 cm3 g−1 (3.79 mmol g−1) and 79 cm3 g−1 (3.52 mmol g−1), and C2H2/CO2 IAST selectivities of 7.4 and 8.1 (1 bar, 1
:
1 mixture, 298 K), respectively. Dynamic column breakthrough experiments resulted in separation factors of 5.26 and 2.05, and CO2 effluent purities of 99.991 and 99.989%, respectively. Temperature programmed desorption experiments at 60 °C resulted in rapid desorption of CO2, followed by fuel grade C2H2 (>98%), affording productivities of 9.45 and 7.96 L kg−1 and maximum C2H2 outlet purities of 99.92% and 99.66%, respectively. This study introduces the use of diamine chelating ligands in HUMs for gas separations through two parent sorbents that are prototypal for families of related materials, one of which, SOFOUR-2-Zn, uses the earth-friendly sulfate anion as a pillar.
Introduction
The first studies that confirmed permanent porosity in porous coordination networks (PCNs)1–3 spawned intense interest in study of PCNs4,5 for their potential utility for gas separations including purification of light hydrocarbons.6–9 One class of PCN, hybrid ultramicroporous materials (HUMs), is comprised of inorganic and organic linker ligands and can exhibit highly selective physisorption.10 Their performance can be attributed to their ultramicroporous (≤7.0 Å) nature, which results in tight sorbate binding, and the strong electrostatics of the inorganic anions that line the pores,11 in effect creating a high density of highly selective binding sites.5 The prototypal HUM, [Zn(SiF6)(pyz)2]n (SIFSIX-3-Zn),12 is comprised of an N-donor linker ligand, pyrazine (3), that links Zn2+ cations to form a cationic 2D square lattice topology, sql, network and SiF62− (SIFSIX) anions that pillar the sql nets to form a 3D primitive cubic, pcu, topology network. SIFSIX-3-Zn was found to offer exceptional trace CO2 capture properties over N2 and CH4,10 and its inherent modularity enabled systematic fine-tuning of composition through substitution of the metal cation, organic linker ligand and/or the SIFSIX pillar, affording improvements in stability and performance.10,13,14 Second generation HUMs pillared by SIFSIX and other MF62− anions (M = Si, Ge, Sn, Ti, Zr) belong to the MFSIX platform.15 Other HUM platforms have been based on different inorganic pillars, including DICRO (Cr2O72−),16,17FOXY (NbOF52−),18,19MFFIVE (AlF52−),20mmo topology nets (CrO42−,MoO42−, WO42−),21 and, most recently, SOFOUR (SO42−).22
A potential application for HUMs is acetylene (C2H2) purification. C2H2 is typically produced via oxidative coupling of CH4 with CO2 as a by-product.9 Since C2H2 and CO2 have the same kinetic diameter of 3.3 Å and similar boiling/sublimation points of 188.4 and 194.7 K, respectively,5,6 sorptive separation is challenging. Industrially, purification is typically achieved using organic solvents, which is inefficient and requires multiple steps to reach the required levels of purity. HUMs such as SIFSIX-dps-Cu,23UTSA-300a24 and DICRO-4-Ni-i25 selectively adsorb C2H2via strong C2H2 binding sites in which F atoms serve as hydrogen bond acceptors, particularly when spaced optimally (ca. 7 Å) to interact with both ends of a C2H2 molecule.6 Recently, the linker ligand bpe, 1,2-bis(4-pyridyl)ethane, afforded a layered flexible HUM, sql-SIFSIX-bpe-Zn, the activated phase of which, sql-SIFSIX-bpe-Zn-β, set a new benchmark for C2H2 adsorption enthalpy (Qst) of 67.5 kJ mol−1.26 This strong binding was attributed to induced fit of C2H2 molecules by enzyme-like adaptable sorbent binding sites and sql-SIFSIX-bpe-Zn-β was found to be highly C2H2-selective over both ethylene and CO2.
Although families of pillared HUMs composed of linear or angular ditopic linker ligands are the most commonly studied HUM variants, other ligand types are possible. HUMs with 4,6-connected fsc topology are composed of tetratopic ligands where the ligand serves as a 4-c node and the metal ion serves as a 6-c node. Examples of ligands and their respective fsc topology HUMs are as follows: metalloligand [Cu2(3-(pyridin-4-yl)acrylate)4] in fsc-2-SIFSIX;27 tetra-(pyridin-4-yl)porphyrin in CPM-131;28 “FTPFs” Cu-Nb-M (M = Zn, Fe, Ni);29 1,2,4,5-tetra(pyridin-4-yl)benzene (tepb) in ZJU-280 (SIFSIX-22-Cu),30SIFSIX-22-Zn,22,31SOFOUR-1-Zn,22 and TIFSIX-Cu-TPB (TIFSIX-6-Cu).32 These materials have been studied for CO2 capture,27 electrocatalysis,29 C2H2/C2H4 separation,30 and C2H2/CO2 separation.22,26 Since the ligand serves as a 4-c node, such PCNs are also amenable to pillar substitution as exemplified by replacement of the SIFSIX pillar in SIFSIX-22-Zn by SO42− (SOFOUR) in SOFOUR-1-Zn22 and SOFOUR-DPDS-Ni (4-DPDS = 4,4′-dipyridyldisulfide),33 and the use of other fluorinated pillars, e.g. TiF62−, SnF62−, GeF62−, ZrF62− and TaF72−, while retaining fsc topology.34 The SO42− pillar has also been used in HUMs such as CuSO4(1,4-bin)1.5 (1,4-bin = 1,4-bisimidazole naphthalene),35 and SOFOUR-TEPE-Zn (TEPE = 1,1,2,2-tetra(pyridin-4-yl) ethene).36
Nevertheless, the availability of linker ligands suitable for HUMs remains somewhat limited in scope and derivatives of chelating ligands such as ethylenediamine (en), which represent a potentially inexpensive and versatile class of ligand, are to our knowledge unexplored. This is despite en and its derivatives representing 7.27% (55
046 entries) of coordination compounds archived in the Cambridge Structural Database (CSD version 5.45, September 2024).37 In this contribution, we report a successful crystal engineering approach to preparing the prototypal members of two HUM platforms comprising a pyridyl functionalised chelating en ligand, enmepy, and their sorption properties in the context of C2H2/CO2 gas separations.
Results and discussion
CSD analysis
In order to design and characterise new HUM platforms based on en derivatives, we first looked for reports of en chelates pillared by MF6 anions (M = Si, Ge, Sn, Ti, Zr, Hf). Database mining of the CSD for coordination compounds containing both an en (or derivative) chelate and a coordinated MFSIX anion afforded just five hits (Table S1 and Fig. S1a†): two ZrF62− complex anions ([(ZrF6)2(ZrF5(OH2))2]6− and [(ZrF6)2]4−); three 1D chains involving SIFSIX or TiF62− (TIFSIX) linkers ([Cu(en)2(SiF6)]n and [Cu(en)2(TiF6)]n). No apparent porosity was present or studied in these compounds.38,39 This lack of sorption candidates prompted us to look at the earth-friendly inorganic pillar SOFOUR and our search (Fig. S1b†) resulted in 25 hits (20 distinct compounds, Table S2;† corresponding to chelating ligands listed in Fig. S2†). [Cu(en)(OH2)2(SO4)]n and [Zn(en)2(SO4)]n are 1D chains with no apparent or permanent porosity.40–45 However, [Zn(enmepy)(SO4)]n (CSD refcode ZOMNOG, hereinafter referred to as SOFOUR-2-Zn) is composed of the chelating en derivative N1,N2-bis(pyridin-4-ylmethyl)ethane-1,2-diamine (enmepy) and Zn2+ cations that form a sql network pillared by SOFOUR to afford a 3D pcu network. Even though SOFOUR-2-Zn contains 1D ultramicropores, no gas sorption data was reported.46 We then searched for other structures containing enmepy chelates that form sql nets similar to SOFOUR-2-Zn. This search, which used the query in Fig. S3,† afforded 62 hits, manual inspection of which revealed 31 sql nets (see Table S3†). The majority (22) are not pillared, with axial positions metal cations coordinated by terminal ligands. The remaining entries were found to be pillared by either 1,4-benzenedicarboxylate derivatives,47,48 MO42− (M = Cr, Mo) or, in the case of SOFOUR-2-Zn, SOFOUR.46 The earth friendly characteristics of SOFOUR over CrO42− and MoO42−, motivated us to characterise the sorption properties of SOFOUR-2-Zn along with its new SIFSIX variant, [Zn(enmepy)(SiF6)]n (SIFSIX-24-Zn).
Crystal structures
SOFOUR-2-Zn was previously reported in 2014, crystallising in the monoclinic space group C2.46 Each octahedral Zn2+ cation is chelated by amino groups of one enmepy and two pyridyl groups from two different enmepy ligands to fill its equatorial positions (Fig. 1a), thereby generating a 2D cationic network with sql topology (Fig. 1b). In each axial position, a SOFOUR anion serves as a pillar to adjacent sql layers so as to form a pcu topology network with an intermetallic distance between sql layers of 6.74 Å (Fig. 1c). The sql layers stack such that triangular 1D channels containing hydrate molecules run parallel to the crystallographic c-axis (Fig. 1b). The calculated void space is 40.9% of unit cell volume after removal of hydrate molecules (mercury contact surface, probe radius 1.2 Å).49
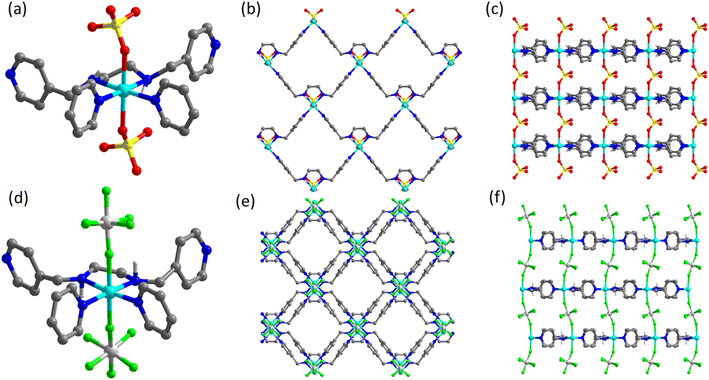 |
| Fig. 1 Crystal structures of SIFSIX-24-Zn and SOFOUR-2-Zn (C–H atoms omitted for clarity, C = grey, H = white, N = blue, O = red, F = green, S = yellow, Si = light beige, Zn = cyan). (a) The octahedral coordination environment around Zn2+ in SOFOUR-2-Zn, (b) SOFOUR-2-Zn packing viewed along a-axis, (c) SOFOUR-2-Zn crystal packing viewed along c-axis, (d) the octahedral coordination environment around Zn2+ in SIFSIX-24-Zn. (e) SIFSIX-24-Zn crystal packing viewed along b-axis, (f) SIFSIX-24-Zn crystal packing viewed along c-axis. | |
SIFSIX-24-Zn was prepared by reacting enmepy with ZnSiF6. Crystals suitable for single crystal X-ray diffraction, SCXRD, were obtained by diffusion of a methanolic solution of enmepy layered onto an ethylene glycol solution of ZnSiF6 (see ESI† for details). SCXRD data revealed that SIFSIX-24-Zn had crystallised in the chiral orthorhombic space group C2221. The equatorial environment of the Zn2+ cations is similar to that of SOFOUR-2-Zn but the SIFSIX pillars form shorter bonds with the Zn cation at the axial positions (Zn–F = 2.148(5) Å vs. Zn–O = 2.223 Å, Fig. 1d). SIFSIX-24-Zn formed the same sql layers found in SOFOUR-2-Zn, resulting in the pcu network illustrated in Fig. 1e and f. The larger SIFSIX anion resulted in intermetallic distance between layers of 7.5759(16) Å (0.833 Å longer than SOFOUR-2-Zn, Fig. 1f). SIFSIX-24-Zn possesses similar 1D pores to SOFOUR-2-Zn but with diffuse electron density which was accounted for by the PLATON SQUEEZE50 procedure in the final structure refinement. The void space (Mercury contact surface, probe radius 1.2 Å) was calculated to be 37.9% of unit cell volume. Further crystallographic details are presented in Table S4.†
In both SOFOUR-2-Zn and SIFSIX-24-Zn, all enmepy ligands within a sql layer orient in the same direction, precluding a crystallographic inversion centre. The stacking of sql layers differs between the two compounds. In SOFOUR-2-Zn, each layer is identical and orients in the same direction, whereas in SIFSIX-24-Zn, the layers alternate in direction and chirality and a pseudo centre of symmetry is present. Though this detail may seem inconsequential for gas adsorption, it impacts pore shape as becomes apparent when examining crystal packing (Fig. 1). The chirality of the sql layers formed by enmepy has been explored by Wen et al., where homochiral batches of crystals could be obtained when using a homochiral template during crystallisation.51–53 The SIFSIX-24-Zn crystal used for SCXRD study was found to be a racemic twin with a Flack parameter of 0.5 (Table S4†).46,47
Gas sorption
Pure gas sorption studies.
In order to perform sorption studies, bulk samples of SOFOUR-2-Zn and SIFSIX-24-Zn were prepared by mixing methanolic solutions of enmepy with aqueous solutions of ZnSO4 and ZnSiF6, respectively, at room temperature, to obtain microcrystalline powders (see ESI† for synthetic details) with Powder X-ray diffraction (PXRD) patterns matching those calculated from SCXRD data (Fig. S4†). Thermogravimetric analysis (TGA) of SOFOUR-2-Zn and SIFSIX-24-Zn indicated loss of solvent molecules at 90 and 75 °C, respectively, and thermal stability up to approximately 300 °C and 200 °C, respectively (Fig. S5†). Following activation at 60 °C under dynamic vacuum, both materials exhibited type-I isotherms for CO2 at 195 K, with saturation uptakes of 135 cm3 g−1 and 178 cm3 g−1, respectively (Fig. 2a), and apparent BET surface areas of 452 m2 g−1 and 590 m2 g−1, respectively (Fig. S6 and S7†). The Horvath–Kawazoe differential pore volume plots from 195 K CO2 isotherms for both compounds were centred around 5.0 Å, classifying them as ultramicroporous (Fig. S8†). SIFSIX-24-Zn exhibited a minor inflection in the 195 K CO2 isotherm in the absolute pressure range 100–200 mmHg. Similarly, SOFOUR-2-Zn revealed inflections at 350–400 mmHg in the CO2 at 195 K isotherm (Fig. 2a), and at 5–10 mm Hg in the N2 at 77 K isotherm (Fig. S9†). Such an inflection was not observed in the 273 K and 298 K CO2 and C2H2 isotherms (Fig. S10 and S11†). At 298 K, the CO2 uptakes of SOFOUR-2-Zn and SIFSIX-24-Zn at 1 bar were observed to be 41 cm3 g−1 (1.78 mmol g−1) and 40 cm3 g−1 (1.79 mmol g−1), respectively (Fig. 2b). The Qst values for CO2, 22.1 kJ mol−1 and 16.8 kJ mol−1, respectively, at low loading (Fig. 2c and S12†) are exceptionally low. In contrast, the uptakes for C2H2 at 298 K were relatively high, 79 and 84 cm3 g−1, or 3.52 and 3.75 mmol g−1, respectively (Fig. 2b), with Qst values of 33.3 kJ mol−1 and 31.8 kJ mol−1, respectively, at low loading.54 Evidently both these sorbents exhibit a relatively high pure component uptake for C2H2 over CO2 at 298 K, which is in the range that could break the usual trade-off between uptake and selectivity.55,56 PXRD patterns measured after activation and adsorption experiments showed retention of the crystal structures of both materials (Fig. S13†).
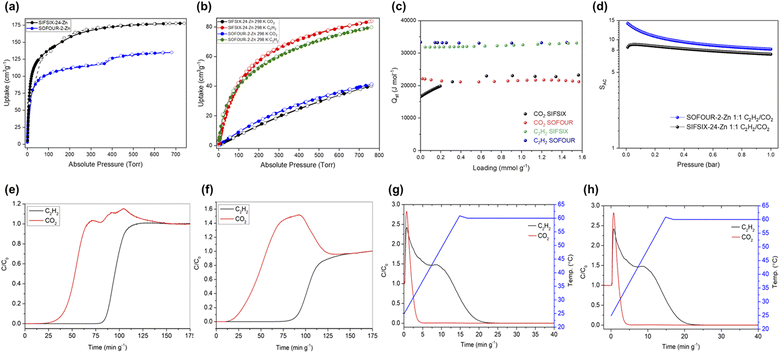 |
| Fig. 2 Gas sorption properties of SIFSIX-24-Zn and SOFOUR-2-Zn: (a) 195 K CO2; (b) 298 K C2H2 and CO2, (c) Qst for C2H2 and CO2. (d) IAST selectivity for C2H2 over CO2 at 298 K and 1 : 1(v/v) mixture; (e) breakthrough curve at 298 K for 1 : 1 C2H2/CO2 mixture for SOFOUR-22-Zn; (f) breakthrough curve at 298 K for 1 : 1 C2H2/CO2 mixture for SIFSIX-24-Zn; (g) temperature programmed desorption after breakthrough experiment for SOFOUR-2-Zn; (h) temperature programmed desorption after breakthrough experiment for SIFSIX-24-Zn. | |
Since single-component gas sorption isotherms revealed significantly higher C2H2 uptakes relative to CO2, ideal adsorbed solution theory (IAST) was applied using the pyIAST Python package57 to determine the selectivity for C2H2 in a 1
:
1 C2H2/CO2 mixture, SAC. We found moderate selectivities for SOFOUR-2-Zn and SIFSIX-24-Zn of 8.2 and 7.3 at 1 bar and 298 K, respectively (Fig. 2d and S14†). Although these selectivity numbers are lower than benchmark physisorbents such as ZNU-1 (56.6),58Zn2(bpy)(btec) (33.3),59sql-16-Cu-NO3-α (27.8),60 and Ni3(HCOO)6 (22),61 they are higher than HUMs such as TIFSIX-2-Ni-i (6.1),62 and NbOFFIVE-3-Ni (6.0)56 (see Table S5† for a list of leading C2H2/CO2 selective PCNs). Combining both IAST selectivity and adsorption capacity recorded at 298 K, the separation potentials for both sorbents were found to be similar at 1 bar and 298 K: 0.78 mmol g−1 for SOFOUR-2-Zn, and 0.76 mmol g−1 for SIFSIX-24-Zn (Fig. S15†).
The water vapour sorption isotherm of SOFOUR-2-Zn reveals a sigmoidal adsorption profile with a total uptake approaching 30 wt% at 95% R.H. (relative humidity), followed by desorption with low hysteresis. In contrast, SIFSIX-24-Zn shows a sudden decrease in uptake at ca. 90% R.H., followed by high desorption hysteresis, typical of moisture-induced phase degradation (Fig. S16†). Accelerated stability tests conducted by incubating activated samples at 40 °C and 75% R.H. show retention of the porous phase of SOFOUR-2-Zn over 5 days, whereas significant new peaks are observed in SIFSIX-24-Zn within 90 hours of exposure (Fig. S17†), indicating that the SO42− pillar enhances framework stability relative to SiF62−.
Dynamic column breakthrough testing.
Encouraged by the single-component gas sorption data, we performed dynamic column breakthrough (DCB) experiments on microcrystalline samples of SOFOUR-2-Zn and SIFSIX-24-Zn. A 1
:
1 C2H2/CO2 mixture was passed through a column packed with activated sample under a total flow rate of 1.0 sccm and the effluent composition was monitored using mass spectrometry. For SOFOUR-2-Zn (Fig. 2e), CO2 breakthrough occurred at 25.4 min g−1, followed by C2H2 at 81.0 min g−1 (55.6 min g−1 later). During this interval, the minimum effluent purity of CO2 was measured to be 99.989% and the saturation uptakes were 47.1 cm3 g−1 and 23.2 cm3 g−1 for C2H2 and CO2, respectively, resulting in a separation factor (αAC) of 2.05. For SIFSIX-24-Zn (Fig. 2f), CO2 breakthrough occurred at 14.2 min g−1 with a minimum effluent purity of 99.991% and C2H2 breakthrough occurred at 78.6 min g−1 (64.4 min g−1 later). Minor fluctuations were observed at the onset of gas breakthrough in all experiments due to a pressure drop across the powdered sorbent bed. The saturation uptakes for C2H2 and CO2 were determined to be 53.0 cm3 g−1 and 10.1 cm3 g−1, respectively (Table S6†), resulting in higher αAC of 5.26, putting SIFSIX-24-Zn in the same performance range as TIFSIX-4-Cu in terms of separation factor and pure component C2H2 uptake (5.4 and 3.85 mmol g−1, respectively).56
Temperature Programmed Desorption (TPD) experiments were conducted after completion of the adsorption branches in DCB experiments for both SOFOUR-2-Zn and SIFSIX-24-Zn (Fig. 2g and h) by replacing the inlet gas mixture flow with 20 sccm of He and applying a temperature ramp of 5 °C min−1 from 25 °C to 60 °C. Desorption was continued until no further adsorbate was detected. We found that 60 °C was sufficient to regenerate both HUMs. Similar TPD curves were obtained for both SOFOUR-2-Zn and SIFSIX-24-Zn, characterised by rapid decreases in effluent CO2 concentration, while C2H2 continued to be released with increasing temperature for a period prior to desorption. Fuel grade C2H2 (>98% purity) was eluted in the interval between 5 and 27 min g−1 for SIFSIX-24-Zn, and between 4 and 20 min g−1 for SOFOUR-2-Zn, corresponding to productivities of 9.45 L kg−1 and 7.69 L kg−1 of fuel grade C2H2 respectively, and peak C2H2 purities of 99.66 and 99.92%, respectively (Table S7†). These results are similar to literature values in terms of peak C2H2 purity for TIFSIX-2-Cu-i,63SIFSIX-22-Zn and SOFOUR-1-Zn,22 which are all 99.9% or higher. The productivities for fuel grade C2H2 in a 1
:
1 C2H2/CO2 mixture are 9.45 L kg−1 and 7.69 L kg−1 for SIFSIX-24-Zn and SOFOUR-2-Zn, respectively. This is much higher than previously reported values of 3.3 L kg−1 and 3.1 L kg−1, for SIFSIX-22-Zn and SOFOUR-1-Zn, respectively.22SOFOUR-2-Zn and SIFSIX-24-Zn also produced significant amounts of high purity C2H2 (99.5%). For SIFSIX-24-Zn, >99.5% C2H2 eluted over the interval 5.8 to 23.9 min g−1, resulting in a productivity of 8.75 L kg−1, only 0.7 L kg−1 less than the production of fuel-grade >98% C2H2, but for SOFOUR-2-Zn, >99.5% C2H2 was eluted only between 5.3 and 9.4 min g−1 resulting in a significantly lower productivity of 3.01 L kg−1. These values are lower than the benchmark of 53.8 L kg−1 for >99.5% C2H2 set by ZNU-1.58 Additional DCB experiments conducted using a humid C2H2/CO2 mixture showed that separation performance was largely unaffected by moisture in both SOFOUR-2-Zn and SIFSIX-24-Zn over a complete adsorption cycle, despite the poor stability of SIFSIX-24-Zn towards moisture (Fig. S18†).
Based on pure component isotherms and IAST calculations, both SOFOUR-2-Zn and SIFSIX-24-Zn should have roughly the same performance. However, DCB experiments revealed that despite showing comparable C2H2 uptakes in mixed-gas conditions, SIFSIX-24-Zn is better in terms of separation factor (2.05 vs. 5.26 for SOFOUR-2-Zn and SIFSIX-24-Zn, respectively). Production of high purity (>99.5%) C2H2 was also higher for SIFSIX-24-Zn than for SOFOUR-2-Zn, although, fuel-grade (>98%) productivity was similar for both. The differences might be attributed to the weaker Qst for CO2 of SIFSIX-24-Zn, 16.8 kJ mol−1 (ΔQst for C2H2/CO2 = 15.0 kJ mol−1), vs. 22.1 kJ mol−1 (ΔQst for C2H2/CO2 = 11.2 kJ mol−1) for SOFOUR-2-Zn.
Sorbate binding sites
To better understand the nature of the C2H2 and CO2 binding sites, density functional theory (DFT) calculations were performed for SOFOUR-2-Zn and SIFSIX-24-Zn (see ESI† for computational methodology). In SOFOUR-2-Zn, the C2H2 molecule orients along one of the channel walls, forming simultaneous hydrogen bonds from both C–H hydrogen atoms to SO42− oxygen atoms, with O⋯H distances of 2.57 Å and 2.62 Å (Fig. 3). In SIFSIX-24-Zn, hydrogen bonds are bifurcated, with the H-atoms being shared between two F-atoms of the same SIFSIX pillar, with distances of 2.38 and 2.66 Å on one end, and 2.93 Å with a contact of 3.66 Å on the other end (Fig. 3). These multi-site short contacts provide a clear basis for the selective C2H2 binding observed in both materials. In contrast, CO2 binding sites are primarily governed by electrostatic interactions between the CO2 carbon and electronegative pillar F and O atoms respectively (Fig. S19†). DFT-derived enthalpy values associated with gas adsorption are presented in Table S8.†
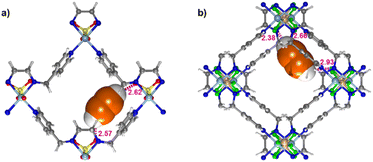 |
| Fig. 3 Ball and stick models of (a) the C2H2 binding site for SOFOUR-2-Zn; (b) the C2H2 binding site for SIFSIX-24-Zn. Short contacts are highlighted as dashed magenta bonds and distances are given in Å. | |
Conclusions
The previously reported material [Zn(enmepy)(SO4)]n (SOFOUR-2-Zn),46 illustrates the potential utility of en based ligands for design of new HUMs with potential utility in gas separations. Pillar substitution using a crystal engineering approach enabled us to isolate [Zn(enmepy)(SiF6)]n (SIFSIX-24-Zn), the prototypal member of a new platform of MFSIX HUMs. Pure component gas sorption studies and dynamic column breakthrough experiments revealed strong performance for the separation of C2H2 from CO2 for both HUMs with appreciable amounts of fuel grade C2H2 (>98%) as shown by temperature programmed desorption of the sorbed gases from a 1
:
1 mixture of C2H2/CO2. Productivities of 9.45 L kg−1 and 7.69 L kg−1 were measured for SIFSIX-24-Zn and SOFOUR-2-Zn, respectively, and SIFSIX-24-Zn produced high purity C2H2 (>99.5%). This study demonstrates for the first time that chelating ethylenediamine based ligands can sustain facile to synthesise HUMs with strong gas separation properties. Both HUM platforms are inherently modular and their scope in terms of composition and properties are under further investigation in our laboratory. We anticipate that SIFSIX-24-Zn and SOFOUR-2-Zn will serve as prototypes for families of second-generation sorbents through metal and/or pillar substitution, as well as ligand modification.
Data availability
The data supporting the findings of this study are available in the ESI† or from the authors upon request.
Author contributions
Project conceptualisation: D. J. O., D. S., S. M. and M. J. Z.; synthesis and characterisation: D. J. O., A. R. and A. A. B.; single component gas sorption: D. S., A. R. and D. J. O.; dynamic column breakthrough and temperature programmed desorption: D. S. and S. M.; molecular modelling: M. V.; single crystal X-ray crystallography: D. J. O.; Cambridge Structural Database analysis: D. J. O. and A. R.; writing – original draft: D. J. O., D. S., S. M., A. A. B., and M. J. Z.; writing – review & editing: all authors; funding acquisition: M. J. Z. and M. V.; supervision: S. M., M. J. Z. and M. V. D. J. O., D. S. and A. R. contributed equally to this work.
Conflicts of interest
There are no conflicts to declare.
Acknowledgements
We gratefully acknowledge Science Foundation Ireland (16/IA/4624, and 12/RC/2278_P2) and the European Research Council (ADG885695). M. V. acknowledges the Irish Centre for High-End Computing (ICHEC) for the provision of computational facilities and support. Amin Koochaki is acknowledged for useful discussions and preliminary modelling studies. S. M. acknowledges an SFI-IRC Pathway award (21/PATH-S/9454) from the Science Foundation Ireland.
Notes and references
- W. Mori, F. Inoue, K. Yoshida, H. Nakayama, S. Takamizawa and M. Kishita, Chem. Lett., 1997, 26, 1219–1220 CrossRef.
- M. Kondo, T. Yoshitomi, H. Matsuzaka, S. Kitagawa and K. Seki, Angew. Chem., Int. Ed., 1997, 36, 1725–1727 CrossRef CAS.
- H. Li, M. Eddaoudi, T. L. Groy and O. M. Yaghi, J. Am. Chem. Soc., 1998, 120, 8571–8572 CrossRef CAS.
- X. Zhao, Y. Wang, D.-S. Li, X. Bu and P. Feng, Adv. Mater., 2018, 30, 1705189 CrossRef PubMed.
- J.-R. Li, R. J. Kuppler and H.-C. Zhou, Chem. Soc. Rev., 2009, 38, 1477–1504 RSC.
- S. Mukherjee, D. Sensharma, K.-J. Chen and M. J. Zaworotko, Chem. Commun., 2020, 56, 10419–10441 RSC.
- L. Yang, S. Qian, X. Wang, X. Cui, B. Chen and H. Xing, Chem. Soc. Rev., 2020, 49, 5359–5406 RSC.
- G. Verma, J. Ren, S. Kumar and S. Ma, Eur. J. Inorg. Chem., 2021, 2021, 4498–4507 CrossRef CAS.
- D. Zhao, K. Yu, X. Han, Y. He and B. Chen, Chem. Commun., 2022, 58, 747–770 RSC.
- P. Nugent, Y. Belmabkhout, S. D. Burd, A. J. Cairns, R. Luebke, K. Forrest, T. Pham, S. Ma, B. Space, L. Wojtas, M. Eddaoudi and M. J. Zaworotko, Nature, 2013, 495, 80–84 CrossRef CAS PubMed.
- S. Mukherjee and M. J. Zaworotko, Trends Chem., 2020, 2, 506–518 CrossRef CAS.
- K. Uemura, A. Maeda, T. K. Maji, P. Kanoo and H. Kita, Eur. J. Inorg. Chem., 2009, 2009, 2329–2337 CrossRef.
- A. Kumar, C. Hua, D. G. Madden, D. O'Nolan, K.-J. Chen, L.-A. J. Keane, J. J. Perry and M. J. Zaworotko, Chem. Commun., 2017, 53, 5946–5949 RSC.
- S. Mukherjee, N. Kumar, A. A. Bezrukov, K. Tan, T. Pham, K. A. Forrest, K. A. Oyekan, O. T. Qazvini, D. G. Madden, B. Space and M. J. Zaworotko, Angew. Chem., Int. Ed., 2021, 60, 10902–10909 CrossRef CAS PubMed.
- N. C. Harvey-Reid, D. Sensharma, S. Mukherjee, K. M. Patil, N. Kumar, S. J. Nikkhah, M. Vandichel, M. J. Zaworotko and P. E. Kruger, ACS Appl. Mater. Interfaces, 2024, 16, 4803–4810 CrossRef CAS PubMed.
- H. S. Scott, A. Bajpai, K.-J. Chen, T. Pham, B. Space, J. J. Perry and M. J. Zaworotko, Chem. Commun., 2015, 51, 14832–14835 RSC.
- H. S. Scott, N. Ogiwara, K.-J. Chen, D. G. Madden, T. Pham, K. Forrest, B. Space, S. Horike, J. J. Perry Iv, S. Kitagawa and M. J. Zaworotko, Chem. Sci., 2016, 7, 5470–5476 RSC.
- P. M. Bhatt, Y. Belmabkhout, A. Cadiau, K. Adil, O. Shekhah, A. Shkurenko, L. J. Barbour and M. Eddaoudi, J. Am. Chem. Soc., 2016, 138, 9301–9307 CrossRef CAS PubMed.
- A. Cadiau, K. Adil, P. M. Bhatt, Y. Belmabkhout and M. Eddaoudi, Science, 2016, 353, 137–140 CrossRef CAS PubMed.
- A. Cadiau, Y. Belmabkhout, K. Adil, P. M. Bhatt, R. S. Pillai, A. Shkurenko, C. Martineau-Corcos, G. Maurin and M. Eddaoudi, Science, 2017, 356, 731–735 CrossRef CAS PubMed.
- M. H. Mohamed, S. K. Elsaidi, T. Pham, K. A. Forrest, B. Tudor, L. Wojtas, B. Space and M. J. Zaworotko, Chem. Commun., 2013, 49, 9809–9811 RSC.
- D. Sensharma, D. J. O'Hearn, A. Koochaki, A. A. Bezrukov, N. Kumar, B. H. Wilson, M. Vandichel and M. J. Zaworotko, Angew. Chem., Int. Ed., 2022, 61, e202116145 CrossRef CAS PubMed.
- J. Wang, Y. Zhang, Y. Su, X. Liu, P. Zhang, R.-B. Lin, S. Chen, Q. Deng, Z. Zeng, S. Deng and B. Chen, Nat. Commun., 2022, 13, 200 CrossRef CAS PubMed.
- R.-B. Lin, L. Li, H. Wu, H. Arman, B. Li, R.-G. Lin, W. Zhou and B. Chen, J. Am. Chem. Soc., 2017, 139, 8022–8028 CrossRef CAS PubMed.
- H. S. Scott, M. Shivanna, A. Bajpai, D. G. Madden, K.-J. Chen, T. Pham, K. A. Forrest, A. Hogan, B. Space, J. J. Perry Iv and M. J. Zaworotko, ACS Appl. Mater. Interfaces, 2017, 9, 33395–33400 CrossRef CAS PubMed.
- M. Shivanna, K.-i. Otake, B.-Q. Song, L. M. van Wyk, Q.-Y. Yang, N. Kumar, W. K. Feldmann, T. Pham, S. Suepaul, B. Space, L. J. Barbour, S. Kitagawa and M. J. Zaworotko, Angew. Chem., Int. Ed., 2021, 60, 20383–20390 CrossRef CAS PubMed.
- S. K. Elsaidi, M. H. Mohamed, T. Pham, T. Hussein, L. Wojtas, M. J. Zaworotko and B. Space, Cryst. Growth Des., 2016, 16, 1071–1080 CrossRef CAS.
- Q. Lin, C. Mao, A. Kong, X. Bu, X. Zhao and P. Feng, J. Mater. Chem. A, 2017, 5, 21189–21195 RSC.
- L. He, J. K. Nath and Q. Lin, Chem. Commun., 2019, 55, 412–415 RSC.
- Q.-L. Qian, X.-W. Gu, J. Pei, H.-M. Wen, H. Wu, W. Zhou, B. Li and G. Qian, J. Mater. Chem. A, 2021, 9, 9248–9255 RSC.
- S. Zou, Z. Di, Y. Liu, Z. Ji, H. Li, C. Chen and M. Wu, Inorg. Chem. Commun., 2022, 137, 109198 CrossRef CAS.
- Y. Liu, Y. Zhang, P. Zhang, Y. Peng, X. Liu, J. Chen, S. Chen, Z. Zeng, J. Wang and S. Deng, Chem. Eng. Process., 2022, 172, 108768 CrossRef CAS.
- J. Liu, H. Shuai, J. Chen, S. Chen, Z. Zhou, J. Wang and S. Deng, Chem & Bio Eng., 2024, 1, 83–90 Search PubMed.
- D. Sensharma, B. H. Wilson, N. Kumar, D. J. O'Hearn and M. J. Zaworotko, Cryst. Growth Des., 2022, 22, 5472–5480 CrossRef CAS PubMed.
- Y. H. Andaloussi, D. Sensharma, A. A. Bezrukov, D. C. Castell, T. He, S. Darwish and M. J. Zaworotko, Cryst. Growth Des., 2024, 24, 2573–2579 CrossRef CAS PubMed.
- X. Liu, P. Zhang, H. Xiong, Y. Zhang, K. Wu, J. Liu, R. Krishna, J. Chen, S. Chen, Z. Zeng, S. Deng and J. Wang, Adv. Mater., 2023, 35, 2210415 CrossRef CAS.
- C. R. Groom, I. J. Bruno, M. P. Lightfoot and S. C. Ward, Acta Crystallogr., Sect. B: Struct. Sci., Cryst. Eng. Mater., 2016, 72, 171–179 CrossRef CAS.
- J. Haníková, J. Černák, J. Kuchár and E. Čižmár, Inorg. Chim. Acta, 2012, 385, 178–184 CrossRef.
- J. Lhoste, K. Adil, A. Le Bail, M. Leblanc, A. Hémon-Ribaud and V. Maisonneuve, J. Fluor. Chem., 2012, 134, 29–34 CrossRef CAS.
- P. C. Healy, C. H. L. Kennard, G. Smith and A. H. White, Cryst. Struct. Commun., 1978, 7, 565–570 CAS.
- V. Manríquez, M. Campos-Vallette, N. Lara, N. González-Tejeda, O. Wittke, G. Díaz, S. Diez, R. Muñoz and L. Kriskovic, J. Chem. Crystallogr., 1996, 26, 15–22 CrossRef.
- M. K. Taylor, D. E. Stevenson, L. E. A. Berlouis, A. R. Kennedy and J. Reglinski, J. Inorg. Biochem., 2006, 100, 250–259 CrossRef CAS PubMed.
- O. V. Kravchina, A. I. Kaplienko, E. P. Nikolova, A. G. Anders, D. V. Ziolkovskii, A. Orendachova and M. Kajnakova, Russ. J. Phys. Chem., 2011, 5, 209–214 CrossRef CAS.
- M. Lutz, S. Smeets and P. Parois, Acta Crystallogr., Sect. E: Crystallogr. Commun., 2010, 66, m671–m672 CrossRef CAS PubMed.
- M. Kajňaková, A. Orendáčová, M. Orendáč, A. Feher, M. Mal'arová and Z. Trávníček, Acta Phys. Pol., 2008, 113, 507–510 CrossRef.
- Y. Wen, T. Sheng, Z. Sun, Z. Xue, Y. Wang, Y. Wang, S. Hu, X. Ma and X. Wu, Chem. Commun., 2014, 50, 8320–8323 RSC.
- Y. Wen, T. Sheng, X. Zhu, C. Zhuo, S. Su, H. Li, S. Hu, Q.-L. Zhu and X. Wu, Adv. Mater., 2017, 29, 1700778 CrossRef PubMed.
- S. Khatua, A. Santra, S. Padmakumar, K. Tomar and S. Konar, ChemistrySelect, 2018, 3, 785–793 CrossRef CAS.
- C. F. Macrae, I. Sovago, S. J. Cottrell, P. T. A. Galek, P. McCabe, E. Pidcock, M. Platings, G. P. Shields, J. S. Stevens, M. Towler and P. A. Wood, J. Appl. Crystallogr., 2020, 53, 226–235 CrossRef CAS PubMed.
- A. Spek, Acta Crystallogr., Sect. C: Struct. Chem., 2015, 71, 9–18 CrossRef CAS PubMed.
- Y. Wen, T. Sheng, S. Hu, X. Ma, C. Tan, Y. Wang, Z. Sun, Z. Xue and X. Wu, Chem. Commun., 2013, 49, 10644–10646 RSC.
- Y. Wen, T. Sheng, S. Hu, Y. Wang, C. Tan, X. Ma, Z. Xue, Y. Wang and X. Wu, CrystEngComm, 2013, 15, 2714–2721 RSC.
- Y. Wen, T. Sheng, C. Zhuo, X. Zhu, S. Hu, W. Cao, H. Li, H. Zhang and X. Wu, Inorg. Chem., 2016, 55, 4199–4205 CrossRef CAS.
- A. Nuhnen and C. Janiak, Dalton Trans., 2020, 49, 10295–10307 RSC.
- N. Kumar, S. Mukherjee, N. C. Harvey-Reid, A. A. Bezrukov, K. Tan, V. Martins, M. Vandichel, T. Pham, L. M. van Wyk, K. Oyekan, A. Kumar, K. A. Forrest, K. M. Patil, L. J. Barbour, B. Space, Y. Huang, P. E. Kruger and M. J. Zaworotko, Chem, 2021, 7, 3085–3098 CAS.
- D. M. Venturi, M. S. Notari, R. Bondi, E. Mosconi, W. Kaiser, G. Mercuri, G. Giambastiani, A. Rossin, M. Taddei and F. Costantino, ACS Appl. Mater. Interfaces, 2022, 14, 40801–40811 CrossRef CAS.
- C. M. Simon, B. Smit and M. Haranczyk, Comput. Phys. Commun., 2016, 200, 364–380 CrossRef CAS.
- L. Wang, W. Sun, Y. Zhang, N. Xu, R. Krishna, J. Hu, Y. Jiang, Y. He and H. Xing, Angew. Chem., Int. Ed., 2021, 60, 22865–22870 CrossRef CAS PubMed.
- Y. Chen, Y. Du, Y. Wang, R. Krishna, L. Li, J. Yang, J. Li and B. Mu, AIChE J., 2021, 67, e17152 CrossRef CAS.
- N. Kumar, S. Mukherjee, A. A. Bezrukov, M. Vandichel, M. Shivanna, D. Sensharma, A. Bajpai, V. Gascón, K.-i. Otake, S. Kitagawa and M. J. Zaworotko, SmartMat, 2020, 1, e1008 CrossRef.
- L. Zhang, K. Jiang, J. Zhang, J. Pei, K. Shao, Y. Cui, Y. Yang, B. Li, B. Chen and G. Qian, ACS Sustain. Chem. Eng., 2019, 7, 1667–1672 CrossRef CAS.
- M. Jiang, X. Cui, L. Yang, Q. Yang, Z. Zhang, Y. Yang and H. Xing, Chem. Eng. J., 2018, 352, 803–810 CrossRef CAS.
- K.-J. Chen, H. S. Scott, D. G. Madden, T. Pham, A. Kumar, A. Bajpai, M. Lusi, K. A. Forrest, B. Space, J. J. I. V. Perry and M. J. Zaworotko, Chem, 2016, 1, 753–765 CAS.
Footnotes |
† Electronic supplementary information (ESI) available: Synthesis, gas sorption, modelling details, crystallographic information. CCDC 2206134. For ESI and crystallographic data in CIF or other electronic format see DOI: https://doi.org/10.1039/d4sc03029j |
‡ These authors contributed equally to this work. |
|
This journal is © The Royal Society of Chemistry 2024 |
Click here to see how this site uses Cookies. View our privacy policy here.