DOI:
10.1039/D4SC04318A
(Edge Article)
Chem. Sci., 2024,
15, 18161-18169
Substituent effect in determining the total structure of an all-alkynyl-protected Ag98 nanocluster for methanol tolerant oxygen reduction reaction†
Received
1st July 2024
, Accepted 8th October 2024
First published on 9th October 2024
Abstract
Metal nanoclusters (NCs) with atomically precise structures are desirable models for truly understanding their structure–property relationship. This study reports the synthesis and structural anatomy of a Ag98 NC protected solely by an alkynyl ligand, 2-(trifluoromethyl)phenylacetylene (2-CF3PhC
CH), which features a –CF3 substituent at the ortho position (ortho-CF3). 2-CF3PhC
CH ligands are so exquisitely arranged on the surface of Ag98 that the steric hindrance caused by ortho-CF3 is minimized but its function as a hydrogen-bond (H-bond) acceptor (H⋯F) is maximized. Such a rule also applies to inter-cluster interactions which define the stacking sequence of Ag98 NCs. When supported on carbon black, Ag98 NCs demonstrate desirable oxygen reduction activity with robust long-term durability and excellent methanol tolerance, outperforming the commercial Pt/C catalyst.
Introduction
Atomically precise metal nanoclusters (NCs) featuring a small metal core and peripheral ligands are desirable models for understanding their physicochemical properties.1–5 To access this type of NC, the choice of ligands is vital. Different ligands have distinct bonding modes, resulting in diverse structures of NCs.6–9 The ligands confine the metal core via metal–ligand bonding, guarantee the NCs' solubility in solvents, and direct their crystallization behavior through ligand–ligand interactions, not to mention their synergistic effects in optical and catalytic applications.10–12 Therefore, understanding the role of ligands has aroused keen interest in recent years.13–15 Up to now, a variety of organic ligands such as thiols,16–20 phosphines,21,22 alkynes23–26 and carbenes27–29 have been successfully applied in the synthesis of coinage metal NCs (Au, Ag, Cu).30
Alternatively, a metal NC can also be regarded as a certain number of ligands being anchored densely on an extremely small metal nanoparticle (usually less than 2 nm). In such narrow space, steric hindrance is pronounced, especially considering the ligands often have a bulky benzene ring as an integral part. The ligands are also restricted by their specific bonding mode. For example, PPh3 usually coordinates with one metal atom, while thiolate tends to form a staple bonding motif.31 Besides, as single crystals, ligands must also strictly comply with crystallographic rules. Constrained by these conditions, ligands tend to exhibit highly regular and symmetric patterns, which in turn shape the outermost layer of metal atoms.32,33 Thus, studying the distribution and coordination patterns of ligands will provide key information for understanding how the ligands shape the NC.34
Previously, the complete structure of alkynyl-protected Ag NCs (e.g., Ag32,35 Ag42,36 Ag48,37 Ag51,38 Ag74,39,40 Ag112 (ref. 41) and Ag148 (ref. 42)) has been determined.43 They mostly employed alkynyl ligands either without any substituent or with substituents at symmetric positions, such as phenylacetylene,36,39,40 3,5-bis(trifluoromethyl) phenylacetylene,23,35,41tert-butylacetylene25,37,44etc. The potential role of an asymmetrically arranged substituent group in determining the crystal structure of metal NCs has yet to be truly unraveled. In this work, a Ag98(2-CF3PhC
C)48Cl4 (denoted as Ag98) NC protected by 2-(trifluoromethyl)phenylacetylene (o-TPA) is synthesized and characterized. O-TPA has one sterically demanding –CF3 substituent at the ortho position (ortho-CF3). It causes the o-TPA ligand to lose its C2 symmetry in comparison with phenylacetylene. This can be used as an indicator of ligand orientation.
Hence, Ag98 where 48 alkynyl ligands are pinned on a 1.5 nm Ag nanoparticle is a desirable model for studying the substituent effect in the following aspects. Firstly, how does the bulky o-TPA adapt to the narrow surface when a sterically demanding ortho-CF3 is present? Secondly, how does the arrangement of surface ligands shape the Ag–ligand interface? Thirdly, will ortho-CF3 influence the inter-cluster interaction and accordingly the whole crystal structure of Ag98? Aiming to resolve these puzzles, a comprehensive structural analysis of Ag98 is implemented. The decisive role of ortho-CF3 in shaping the crystal structure is revealed. When supported on activated carbon, Ag98 exhibits excellent oxygen reduction reaction (ORR) activity with superior durability and methanol tolerance compared with a commercial Pt/C catalyst.
Experimental
Materials and methods
All the chemicals were of analytical grade, obtained from commercial sources and used as received. Bis(diphenylphosphino)propane (Dppp) and 2-(trifluoromethyl)phenylacetylene (2-CF3PhC
CH) were purchased from Adamas Reagent Co., Ltd. Silver nitrate (AgNO3, 99.8%) and sodium borohydride (NaBH4, 98%) were from Energy Chemical (Shanghai, China). Dichloromethane and the other reagents employed were purchased from Sinopharm Chemical Reagent Co., Ltd (Shanghai, China).
Synthesis of Ag98(2-CF3PhC
C)48Cl4 nanoclusters
AgNO3 (17 mg, 0.1 mmol) was dissolved in 1 mL ethanol, then a 2 mL CH2Cl2 solution containing Dppp (1,3-bis(diphenylphosphino)propane) (21 mg, 0.05 mmol) and 2-CF3PhC
CH (14 μL, 0.1 mmol) was added under vigorous stirring. A freshly prepared solution of NaBH4 (0.1 mmol in 1 mL ethanol) was added dropwise after that. The reaction was aged for 30 h under ambient conditions, during which the color further changed from light yellow to dark brown red. The isolated precipitate was dissolved in CH2Cl2 (2 mL) and centrifuged for 10 min at 12
000 rpm. The supernatant solution was layered with n-hexane and diethyl ether (volume ratio, 1
:
1) for diffusion. Black block crystals were obtained after one week with a yield of 24% (based on Ag).
Electrochemical test
The ORR performance of the catalysts was tested by cyclic voltammetry (CV) and linear sweep voltammetry (LSV) on a CHI 760E electrochemical workstation with a typical three-electrode system, in which a rotating disk electrode (RDE, d = 3.0 mm) loaded with catalysts was used as the working electrode, Ag/AgCl (saturated KCl) as the reference electrode and Pt wire as the auxiliary electrode. The CV and LSV curves were recorded in O2-saturated 0.1 M KOH electrolyte. All potentials were converted to the reversible hydrogen electrode (RHE) scale according to the formula:
ERHE = EAg/AgCl + 0.0592 pH + 0.198 V |
Physical measurements
UV-vis absorption spectra were recorded on a TU-1950 UV-vis spectrophotometer with samples being dispersed in CH2Cl2. FT-IR spectra were collected on a Nicolet iS5 with samples prepared as KBr pellets. X-ray Photoelectron Spectroscopy (XPS) for Ag, C, F, and Cl was carried out using a monochromatic Al Kα (1486.69 eV) X-ray source operated on a Thermo Fisher Scientific K-Alpha, and the spectra were calibrated using the C 1s peak at 284.8 eV. Thermogravimetric analysis (TGA) was performed on a Setaram Labsys Evo TG-DSC/DTA analyzer in a N2 atmosphere with a heating rate of 10 °C min−1 from 50 °C to 800 °C. The powder X-ray diffraction (XRD) analysis of the obtained crystal samples was carried out using a Bruker D2 PHASER diffractometer with Cu Kα radiation ranging from 5° to 90°.
Crystallography
The crystallographic data of Ag98 was obtained on an Agilent Technologies SuperNova Single Crystal Diffractometer using Cu Kα radiation (λ = 1.54184 Å) at 150 K. Absorption corrections were applied by using the program CrysAlis45 (multi-scan). The structure was solved and refined using full-matrix least-squares based on F2 with program SHELXT and SHELXL within OLEX2.46 All the non-hydrogen atoms were refined anisotropically and H atoms isotropically; all hydrogen atoms were generated geometrically and constrained to ride on their parent atoms. For the –CF3 group, RIGU, SIMU, DFIX and DANG constraints are often applied due to disorder. The bond lengths of C–F were set to ∼1.35 ± 0.01 Å. The bond lengths of C–C were set to ∼1.54 ± 0.01 Å. The bond lengths of C
C were set to ∼1.20 ± 0.01 Å. Meanwhile the distances between the 1,3-atoms of C⋯F and F⋯F were set to ∼2.5 ± 0.01 Å and 2.2 ± 0.01 Å. Some aromatic rings were treated by using rigid constraints (AFIX 66).
Results and discussion
Crystal structure from single crystal X-ray diffraction
As shown in Fig. 1, Ag98 is a 1.5 nm Ag metal core protected by 48 o-TPAs and 4 Cl ligands (Fig. S2†). It crystallizes in a trigonal lattice, R
space group (Fig. S3†). All 48 o-TPAs employ the μ3-bridging mode (Fig. 1c and S4†) via σ-type or π-type bonds. Notably, ortho-CF3 causes the benzene rings to tilt in the opposite direction from the CF3 group. This is true for almost every o-TPA ligand, whose tilt angles range from 14° to 43° (Table S1†). Apparently, ortho-CF3 introduces steric hindrance into the local coordination environment. The metal core consists of 98 silver atoms that are arranged into a Ag10@Ag16@Ag72 three-shell architecture. The innermost core is a Ag10 tetrahedron. It can be viewed as a unit cut from a face-centered cubic (FCC) silver (Fig. 1d). The average Ag–Ag bond distance of the Ag10 shell is 2.884 Å (Fig. S5†), close to that in bulk Ag (2.889 Å).47 This shows the metallic nature of Ag98. Each of the four tetrahedron facets is extended by another Ag10 tetrahedron. In this fashion, Ag10@Ag16 appears as a star-shaped polyhedron (Fig. 1e). The outermost Ag72 shell is composed of four bowl-like Ag18 units. Each one caps a convex tetrahedron of the star polyhedron (Fig. S6†). Four μ3-Cl moieties are tetrahedrally arranged, through one of them passes the 3-fold rotation axis (Fig. 1f). Considering there is no Cl element in the metal precursor or ligands, it likely results from the solvent, CH2Cl2.
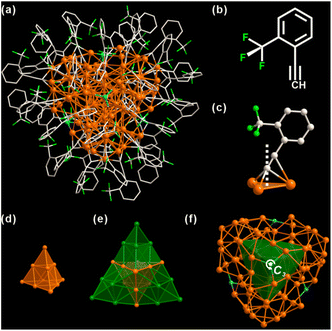 |
| Fig. 1 (a) Overall structure of Ag98(2-CF3PhC C)48Cl4 (Ag98). (b) Structure of 2-CF3PhC CH (o-TPA). (c) Bonding motif of o-TPAs with Ag atoms. The white dotted line is the normal line from the alkynyl C atom to the Ag3 plane. (d) Inner Ag10 tetrahedron. (e) Ag10@Ag16 shells. (f) Ag10@Ag16@Ag72 core viewed along the C3-axis. Ag16 is represented by a green polyhedron. Color labels: orange and green, Ag; grey, C; blue, Cl; fluorescent green, F; white, H. | |
There is a total of 48 alkynyl ligands, o-TPAs, on Ag98. For ease of discussion, the metal core is divided into two areas, colored in green and gray respectively (Fig. 2a). O-TPAs are accordingly divided into four groups: 24 white, 15 yellow, 6 purple, and 3 orange o-TPAs. 24 white o-TPAs form a “three-leaf clover” structure (Fig. 2b). Each “leaflet” includes eight o-TPAs that can be further divided into two equal parallel subgroups. The bottom view in Fig. 2c includes 21 o-TPAs. They form a “shield-like” shape.
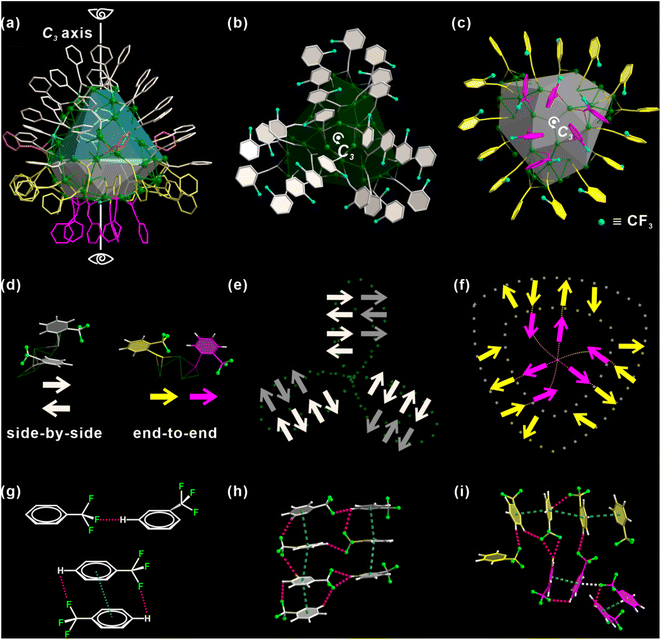 |
| Fig. 2 (a) Distribution of the ligands on Ag98. They are separated into groups by different colors. “Three-leaf clover” (b) and “shield-like” (c) ligand ensembles of Ag98. (d) “Side-by-side” and “end-to-end” position relations between two neighboring o-TPA ligands. Simplified schematic illustration of the o-TPA ligand of “three-leaf clover” (e) and “shield-like” (f) structures. An arrow represents an o-TPA ligand. It approximately points from the center of the benzene ring to the carbon atom of ortho-CF3. (g–i) The intra-cluster C–F⋯H (red dashed lines), π–π (green dashed lines) and C–F⋯π (white dashed line) interactions. Color labels: green, Ag; gray, orange, yellow and purple, C; fluorescent green, F; blue, Cl; white, H. | |
Six purple o-TPAs are arranged into a triangle that is surrounded by the outer 15 yellow o-TPAs. For convenience, each o-TPA is represented by an arrow, which approximately points from the center of the benzene ring to the carbon atom of ortho-CF3, as explained in Fig. 2d. As such, “three-leaf clover” and “shield” ligand ensembles are simplified as the patterns in Fig. 2e and f. It is shown that for two adjacent o-TPA ligands, they have two positional relationships: “side-by-side” and “end-to-end” (Fig. 2d). For any two neighboring “side-by-side” o-TPAs of either “three-leaf clover” or “shield” ligand ensembles, their arrows point in opposite directions so their ortho-CF3 moieties are away from each other. Their benzene rings are arranged nearly parallelly. This not only minimizes the steric hindrance between o-TPAs but also lets them fully interact via C–F⋯H and π–π interactions, as illustrated in Fig. 2g. For the “end-to-end” o-TPA ligand pair, their arrows point in the same direction. This avoids direct contact between the two ortho-CF3 and guarantees the formation of C–F⋯H hydrogen bonds (H-bond) (Fig. 2g). It is conceivable that if one of the two adjacent o-TPAs points to the opposite direction, two ortho-CF3 would contact directly. Fig. 2h and i provide the details of multiple C–F⋯H H-bonds (red dashed lines). Basically, each ortho-CF3 group functions as a hydrogen bond acceptor. The distances of H-bonds are in the range of 2.6 to 3.0 Å. In other words, o-TPAs are arranged in such an exquisite manner that the capability of ortho-CF3 as an H-bond acceptor is maximized but the steric effect caused by it is minimized. As shown in Fig. S7,† the steric effect is analyzed using the web tool SambVca 2.47 The closely arranged dark red strips indicate prominent steric hindrance caused by the rigid benzene rings. The areas enclosed by green dashed boxes result from ortho-CF3. Their orange color indicates a significant steric effect caused by ortho-CF3, although it is slightly less than that of the benzene ring.
The distribution of o-TPAs and the surface Ag atoms are interdependent. Each “leaflet” of the “three-leaf clover” structure includes 19 edge-sharing Ag3 triangles (Fig. S8a†) capped by eight o-TPAs. Each o-TPA bonds with a Ag3 triangle around the periphery of the “leaflet”. In the purple part, six Ag3 triangles are connected by corner-sharing to form a large Ag6 triangle (Fig. S8b†). A Cl atom is at the center through which passes the C3 rotation axis. Thirty-six Ag atoms in the yellow part form six linked pentagonal pyramids. Two apexes of neighboring pyramids are located either above or below the pentagon base (Fig. S8c and S8f†). All o-TPA are in the μ3-bridging mode (Fig. S8†).
Not only the individual Ag98, but also the inter-cluster structure is significantly affected by ortho-CF3. For ease of discussion, Ag98 is also divided into two parts: the “three-leaf clover” top part (red, Fig. 3a) and a “shield-like” bottom part (pink). Each such Ag98 has six nearest neighbor Ag98 NCs, and each side has three. As shown in Fig. 3b, the top part of the center Ag98 is in close proximity to the same parts of another three Ag98 NCs (red dashed box area in Fig. 3b). The situation is similar for the bottom part. Such an arrangement necessitates the opposite orientations of Ag98 NCs of adjacent layers. Such a situation is illustrated in the view along the b-axis (Fig. 3c). Correspondingly, the inter-cluster ligand interactions are either “leaf-to-leaf” or “shield-to-shield” (Fig. 3d and g). A perpendicular view of the involved top area as framed by a red dashed rectangle (Fig. 3b) is shown in Fig. 3d. One “leaflet” overlaps with a “leaflet” of another Ag98. In this way, the “three-leaf clover” pattern forms interactions with three neighboring Ag98 NCs.
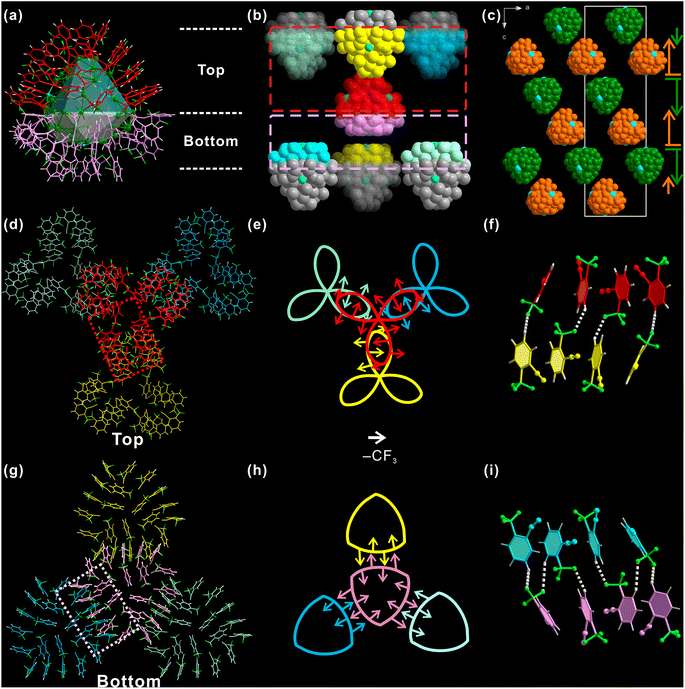 |
| Fig. 3 (a) Forty-eight 2-CF3PhC CH (o-TPA) ligands colored in red and pink on Ag98. (b) One centered Ag98 (red and pink) with its six neighboring Ag98 NCs. (c) Orientations of Ag98 NCs. Different orientations are shown in different colors (orange or green). Cross-section views of “three-leaf clover” ligands (d) and “shield-like” ligands (g) of one Ag98 interacting with the same parts of another three Ag98. (e) and (h) are simplified schematic representations of the patterns (d) and (g), respectively. An arrow is an o-TPA, it approximately points from the center of the benzene ring to the carbon of the ortho-CF3 substituent. (f) and (i) are the details of bonding of (e) and (h). The color code is the same as that in (e) and (h). | |
To better understand such a pattern, “three-leaf clover” is represented by a Kiepert curve that also has a C3 rotation symmetry (Fig. 3e). Each arrow of it stands for one o-TPA ligand and it points from the center of the benzene ring to ortho-CF3, the same as discussed above. It is found that although these o-TPAs are from different Ag98 NCs, they still follow the rule observed on an individual NC. That is “side-by-side” o-TPAs have their ortho-CF3 facing the opposite directions and “end-to-end” o-TPAs have their ortho-CF3 pointing in the same direction. As shown in Fig. 3f, a red and a yellow o-TPA from respective Ag98 NCs are arranged end-to-end, forming a C–F⋯H H-bond. The neighboring pairs of o-TPA have similar interactions but face the opposite direction. Similarly, the ligands in the “shield-like” part are simplified to the Reuleaux triangle. In Fig. 3h, the rule observed on the Kiepert curve also applies to the Reuleaux triangle (Fig. 3i). Thus, the orientation and distribution of ortho-CF3 is not only critical for determining the ligand arrangement on an individual Ag98, but is also decisive in shaping the inter-cluster structure.
The Hirshfeld surface, which defines an outer surface of electron density, encodes information about intermolecular interactions.48,49 The analytical results for the “shield-like” part are shown in Fig. 4. The external distances from the Hirshfeld surfaces to the nearest nucleus, de, are shown in Fig. 4b. Three red areas which indicate short-distance interactions are trigonally distributed around the center. This results from the proximity of another three trigonally distributed Ag98 NCs, as described in the crystallographic results (Fig. 3 and S9†). The normalized distance (dnorm) highlights the contacts shorter than the van der Waals radius as red spots (Fig. 4c).
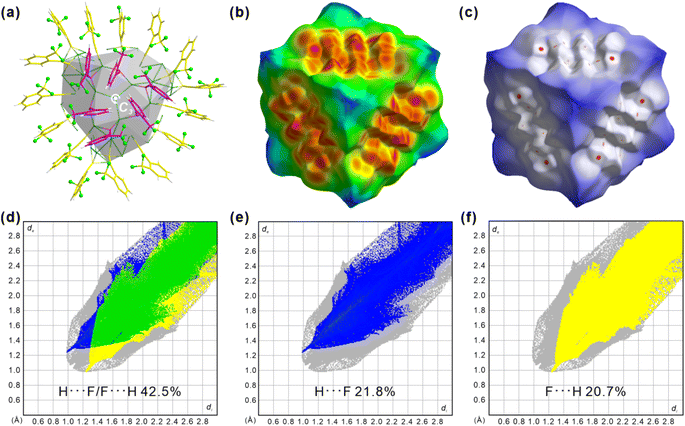 |
| Fig. 4 (a) “Shield-like” part of Ag98 viewed along the c-axis. Hirshfeld surface of the structure in (a) as mapped by de (b) and dnorm (c) surfaces. Fingerprint plots (d) H⋯F/F⋯H, (e) H⋯ and (f) F⋯H contacts. The full fingerprint appears beneath each decomposed plot as a grey shadow. | |
Three groups of red spots have nearly the same numbers and shapes, corresponding to the same bond numbers and distances. A decomposition analysis in Fig. S10† proves that the H⋯F/F⋯H H-bond dominates the short interactions, although F⋯F and H⋯H are also present. Furthermore, the fingerprint plot in Fig. 4f shows two spikes which have the characteristic shape for H-bonding (upper one for a H-bond donor and lower one for an acceptor). Ag98 has its one o-TPA functioning as an acceptor (F atom) and its neighboring o-TPA as a donor (H of benzene) (Fig. S11†). In all, H⋯F/F⋯H H-bonding contributes 42.5% to the Hirshfeld surface (Fig. S12 and S13†), much higher than other non-H⋯H contacts. In other words, C–F⋯H H-bonds dominate the inter-cluster interactions and direct the packing sequence of Ag98. This further highlights the importance of the ortho-CF3 substituent as an H-bond acceptor.
Ag98 was characterized by different techniques. The disappearance of the stretching mode of ν(
C–H) at 3300 cm−1 in the FT-IR spectrum of Ag98 indicates the coordination of o-TPA with Ag (Fig. S14†). Upon addition of NaBH4, a new absorption peak in the UV-vis spectrum appeared, which gradually red-shifted from 398 to 432 nm, and finally to 522 nm during aging (Fig. S15 and S16†). It is characteristic of Ag98. X-ray photoelectron spectroscopy (XPS) of Ag98 reveals all expected elements of Ag98 (Fig. S18 and S19†).50 The Ag 3d5/2 signals can be deconvoluted into signals at 369.1 eV (3d5/2, Ag0) and 366.9 eV (3d5/2, Ag+). Also, the thermogravimetric analysis (TGA) curve proves that Ag98 remains stable up to 180 °C (Fig. S21†).
To gain further insights, the electronic structure of Ag98 was examined using density functional theory (DFT) calculations. To simplify the computations, benzene rings were replaced with methyl groups. The neutral singlet state of the Ag cluster displays a unique and stable electronic configuration. Fig. 5 accurately illustrates the spatial distribution of the highest occupied molecular orbital (HOMO) and the lowest unoccupied molecular orbital (LUMO). The HOMO orbitals, being the least stable electron residence, indicate areas on the Ag cluster where electrons are most likely to detach, particularly around the –CH3 groups and the –Ag–C
C motifs. Conversely, the LUMO orbitals highlight the potential sites for the cluster to accept additional electrons, which are centered around the Ag core at their lowest energy state, thus predicting the cluster's potential electron affinity. Specifically, in the neutral singlet state, the HOMO has an energy of −2.709 eV, while for the LUMO it is −2.365 eV, yielding a HOMO–LUMO gap of 0.344 eV. Analysis of the total density of states (TDOS) in Fig. 5c indicates that the Ag cluster exhibits metallic characteristics, with an even distribution of state density near the HOMO orbital energy level. This corresponds to an sp-like band, suggesting that the Ag cluster possesses electron delocalization properties. Additionally, there are prominent peaks at −6.2 eV and 4.9 eV, indicating the presence of pseudo energy gaps (1.5 eV). This further suggests that the Ag cluster exhibits covalent bonding character. The primary contributions to the DOS near the Fermi level come from Ag 5s orbital electrons. This indicates that the potential sites for accepting electrons are around the Ag atoms, which should facilitate its electrocatalytic applications.
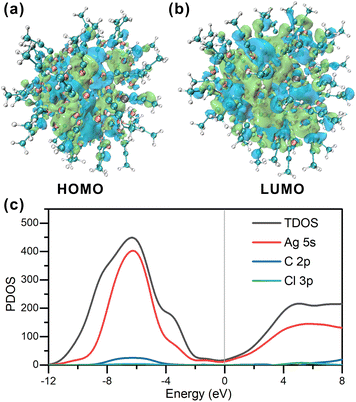 |
| Fig. 5 (a and b) Frontier orbitals of Ag98. (c) PDOS of Ag98. The HOMO levels are positioned at the zero-energy level. | |
Electrocatalytic ORR with Ag98
Thus, the ORR catalytic performance of the Ag98/C material was evaluated on a glassy carbon electrode in a three-electrode system with a 0.1 mol L−1 KOH electrolyte. A carbon rod and an Ag/AgCl electrode served as the counter electrode and reference electrode, respectively. The tunable d-orbital electronic structure of the transition metal silver allows for an optimal adsorption energy for oxygen molecules, which could effectively reduce the activation energy barrier of the ORR. Compared to the bulk materials, Ag NCs possess a high specific surface area and more active sites. These effects enhance the electron transfer efficiency between Ag NCs and reactants, thereby modulating both the reaction pathway and activity of the ORR.51 From the linear sweep voltammetry (LSV) curves in Fig. 6a, it can be observed that the Ag98/C exhibits desirable ORR performance with a high half-wave potential (E1/2) of 0.76 V and a large limiting current density (JL) of −5.2 mA cm−2, close to that of the Pt/C catalyst (E1/2: 0.84 V, JL: −4.5 mA cm−2). Notably, the oxygen reduction potential and E1/2 of Ag98/C significantly exceed those of pure carbon black (Fig. S23†), revealing the desirable ORR electrocatalytic activity of Ag98. Moreover, the E1/2 and jL values of Ag98/C are even superior to those of most recently reported similar electrocatalysts (Fig. 6b and Table S2†).30,52–55 On the other hand, Ag98/C samples were also treated by a fast thermal treatment method to remove the ligands without sintering them.56 They displayed a slightly reduced E1/2 value (0.66 V), suggesting the surface ligands had a minor promoting effect on the ORR performance, which could be ascribed to the electronic effect caused by the bonding with alkynyl ligands (Fig. S24†). Also, the Tafel slope of the Ag98/C is calculated to be 56.9 mV dec−1, lower than that of Pt/C (97.7 mV dec−1, Fig. 6c), demonstrating its facilitated kinetics and higher efficiency in the catalytic process of ORR. As shown in Fig. 6d, the H2O2 yield and electron transfer number (n) calculated from the LSV curves measured on a rotating ring-disk electrode (RRDE) are 14.5% and 3.7, respectively, evaluating the selectivity of Ag98/C for O2 reduction through near 4e− transfer steps. Additionally, Ag98/C achieves prominent durability with high current retention of 85.3% after continuous operation for 23 h, whereas Pt/C retains only 60.1% after 10 h of cycling (Fig. 6e). This may be attributed to the high dispersion of silver nanoclusters over the conductive carbon substrate and strong interactions, which could prevent nanoclusters from easy abscission during continuous stability testing. Also, the chronoamperometric curve of Ag98/C in Fig. 6f remains nearly unchanged after methanol injection, while the current for Pt/C significantly declines, revealing the superior methanol tolerance of the Ag98 NCs compared to the Pt/C catalyst. This is due to silver having a relatively low catalytic activity compared to methanol, which reduces its corrosion by methanol.57 Additionally, the electronic structure of silver allows it to preferentially adsorb oxygen molecules in the ORR, further decreasing the accessibility to methanol. In contrast, platinum is more prone to reacting with methanol, leading to poor methanol tolerance performance.58 The steric hindrance of the ortho-CF3 ligand may also make it difficult for methanol molecules to approach the active sites, thereby further enhancing its methanol tolerance performance.
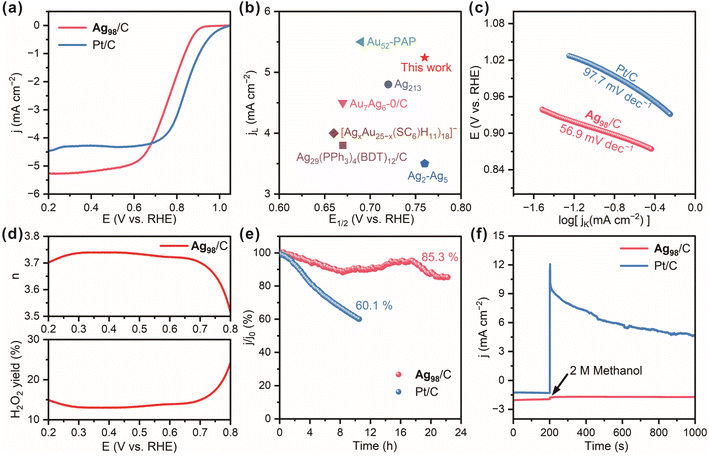 |
| Fig. 6 (a) ORR LSV curves of Ag98/C and Pt/C in an O2-saturated 0.1 M KOH solution at a scan rate of 10 mV s−1 and a rotation speed of 1600 rpm. (b) Comparison of the E1/2 and jL values between Ag98/C and the reported cluster catalysts. (c) The Tafel slopes of Ag98/C and Pt/C catalysts. (d) Electron transfer numbers (n) and H2O2 yield of Ag98/C. (e) Chronoamperometric curves for the ORR of Ag98/C and Pt/C catalysts. (f) The methanol-tolerance performances of Ag98/C and Pt/C catalysts. | |
Conclusions
This work highlights the unanticipated significance of an ortho-CF3 substituent of an alkynyl ligand in determining not only the distribution of ligands on an individual Ag nanocluster but also the inter-cluster interactions. The steric hindrance caused by ortho-CF3 is minimized but its function as an H-bond acceptor is maximized. When supported on carbon black, Ag98 nanoclusters exhibit excellent methanol tolerance and robust long-term durability in the oxygen reduction reaction.
Data availability
Data are available on request from the authors.
Author contributions
Ting Li and Xiaoqin Cui: synthesis, structural analysis. Huan Li and Xiaoqin Cui: writing of the paper. Ting Li and Xiaoqin Cui: data collection on an X-ray diffractometer and crystal structure refinement. Xuehuan Zhang and Xiaoqin Cui: data testing and analysis of electrocatalytic ORR. Huan Li, Sheng Zhu and Gaoyi Han: conceptualization, funding, and revision of the paper. All authors have discussed the results and contributed to the final manuscript.
Conflicts of interest
There are no conflicts to declare.
Acknowledgements
This work was supported by the National Natural Science Foundation of China (21972080 and 21503123), Natural Science Research Foundation of Shanxi Province (202303021221060) and Shanxi “1331 Project”.
Notes and references
- G. Yang, Z. Wang, F. Du, F. Jiang, X. Yuan and J. Y. Ying, J. Am. Chem. Soc., 2023, 145, 11879–11898 CrossRef CAS PubMed.
- M. Wang, Y. Chen and C. Tang, Chem.–Asian J., 2023, 18, e202300463 CrossRef CAS PubMed.
- D. Cheng, R. Liu and K. Hu, Front. Chem., 2022, 10, 958626 CrossRef CAS PubMed.
- X. Liu, J. Yuan, C. Yao, J. Chen, L. Li, X. Bao, J. Yang and Z. Wu, J. Phys. Chem. C, 2017, 121, 13848–13853 CrossRef CAS.
- I. Chakraborty and T. Pradeep, Chem. Rev., 2017, 117, 8208–8271 CrossRef CAS PubMed.
- Y. Negishi, Phys. Chem. Chem. Phys., 2022, 24, 7569–7594 RSC.
- T.-H. Chiu, J.-H. Liao, R. P. B. Silalahi, M. N. Pillay and C. W. Liu, Nanoscale Horiz., 2024, 9, 675–692 RSC.
- C. M. Aikens, Acc. Chem. Res., 2018, 51, 3065–3073 CrossRef CAS PubMed.
- F. Hu, R.-L. He, Z.-J. Guan, C.-Y. Liu and Q.-M. Wang, Angew. Chem., Int. Ed., 2023, 62, e202304134 CrossRef CAS PubMed.
- H. Xu and K. S. Suslick, Adv. Mater., 2010, 22, 1078–1082 CrossRef CAS PubMed.
- Y. Jin, C. Zhang, X.-Y. Dong, S.-Q. Zang and T. C. W. Mak, Chem. Soc. Rev., 2021, 50, 2297–2319 RSC.
- X. Li, S. Takano and T. Tsukuda, J. Phys. Chem. C, 2021, 125, 23226–23230 CrossRef CAS.
- J. Yan, B. K. Teo and N. Zheng, Acc. Chem. Res., 2018, 51, 3084–3093 CrossRef CAS PubMed.
- X. Li, F. Zhang, X. Han, J.-H. Wang, X. Cui, P. Xing, H. Li and X.-M. Zhang, Nano Res., 2023, 16, 8003–8011 CrossRef CAS.
- A. Aparna, H. Sreehari, A. Chandran, K. P. Anjali, A. M. Alex, P. Anuvinda, G. B. Gouthami, N. P. Pillai, N. Parvathy, S. Sadanandan and A. Saritha, Talanta, 2022, 239, 123134 CrossRef CAS PubMed.
- N. Yan, N. Xia, L. Liao, M. Zhu, F. Jin, R. Jin and Z. Wu, Sci. Adv., 2018, 4, eaat7259 CrossRef CAS PubMed.
- T. Higaki, C. Liu, M. Zhou, T.-Y. Luo, N. L. Rosi and R. Jin, J. Am. Chem. Soc., 2017, 139, 9994–10001 CrossRef CAS PubMed.
- M.-X. Ma, X.-L. Ma, G.-M. Liang, X.-T. Shen, Q.-L. Ni, L.-C. Gui, X.-J. Wang, S.-Y. Huang and S.-M. Li, J. Am. Chem. Soc., 2021, 143, 13731–13737 CrossRef CAS PubMed.
- L. Sumner, N. A. Sakthivel, H. Schrock, K. Artyushkova, A. Dass and S. Chakraborty, J. Phys. Chem. C, 2018, 122, 24809–24817 CrossRef CAS.
- C. P. Joshi, M. S. Bootharaju, M. J. Alhilaly and O. M. Bakr, J. Am. Chem. Soc., 2015, 137, 11578–11581 CrossRef CAS PubMed.
- R. H. Adnan, J. M. L. Madridejos, A. S. Alotabi, G. F. Metha and G. G. Andersson, Adv. Sci., 2022, 9, 2105692 CrossRef CAS PubMed.
- Q.-F. Zhang, P. G. Williard and L.-S. Wang, Small, 2016, 12, 2518–2525 CrossRef CAS PubMed.
- M.-M. Zhang, X.-Y. Dong, Y.-J. Wang, S.-Q. Zang and T. C. W. Mak, Coord. Chem. Rev., 2022, 453, 214315 CrossRef CAS.
- Y.-X. Wang, J. Zhang, H.-F. Su, X. Cui, C.-Y. Wei, H. Li and X.-M. Zhang, ACS Nano, 2023, 17, 11607–11615 CrossRef CAS PubMed.
- L. Qin, F. Sun, X. Ma, G. Ma, Y. Tang, L. Wang, Q. Tang, R. Jin and Z. Tang, Angew. Chem., Int. Ed., 2021, 60, 26136–26141 CrossRef CAS PubMed.
- Z. Lei, X.-K. Wan, S.-F. Yuan, Z.-J. Guan and Q.-M. Wang, Acc. Chem. Res., 2018, 51, 2465–2474 CrossRef CAS PubMed.
- H. Shen, Z. Xu, M. S. A. Hazer, Q. Wu, J. Peng, R. Qin, S. Malola, B. K. Teo, H. Häkkinen and N. Zheng, Angew. Chem., Int. Ed., 2021, 60, 3752–3758 CrossRef CAS PubMed.
- E. C. Hurst, K. Wilson, I. J. S. Fairlamb and V. Chechik, New J. Chem., 2009, 33, 1837–1840 RSC.
- J. Vignolle and T. D. Tilley, Chem. Commun., 2009, 7230–7232 RSC.
- S. Zhuang, D. Chen, W.-P. Ng, D. Liu, L.-J. Liu, M.-Y. Sun, T. Nawaz, X. Wu, Y. Zhang, Z. Li, Y.-L. Huang, J. Yang, J. Yang and J. He, JACS Au, 2022, 2, 2617–2626 CrossRef CAS PubMed.
- R. Anumula, A. C. Reber, P. An, C. Cui, M. Guo, H. Wu, Z. Luo and S. N. Khanna, Nanoscale, 2020, 12, 14801–14807 RSC.
- C. Zeng, Y. Chen, K. Kirschbaum, K. J. Lambright and R. Jin, Science, 2016, 354, 1580–1584 CrossRef CAS PubMed.
- R.-W. Huang, J. Yin, C. Dong, A. Ghosh, M. J. Alhilaly, X. Dong, M. N. Hedhili, E. Abou-Hamad, B. Alamer, S. Nematulloev, Y. Han, O. F. Mohammed and O. M. Bakr, J. Am. Chem. Soc., 2020, 142, 8696–8705 CrossRef PubMed.
- B. Zhang, J. Chen, Y. Cao, O. J. H. Chai and J. Xie, Small, 2021, 17, 2004381 CrossRef CAS PubMed.
- L. Chen, F. Sun, Q. Shen, L. Qin, Y. Liu, L. Qiao, Q. Tang, L. Wang and Z. Tang, Nano Res., 2022, 15, 8908–8913 CrossRef CAS.
- S.-S. Zhang, H.-F. Su, Z. Wang, L. Wang, Q.-Q. Zhao, C.-H. Tung, D. Sun and L.-S. Zheng, Chem.–Eur. J., 2017, 23, 3432–3437 CrossRef CAS PubMed.
- S.-S. Zhang, F. Alkan, H.-F. Su, C. M. Aikens, C.-H. Tung and D. Sun, J. Am. Chem. Soc., 2019, 141, 4460–4467 CrossRef CAS PubMed.
- G.-X. Duan, L. Tian, J.-B. Wen, L.-Y. Li, Y.-P. Xie and X. Lu, Nanoscale, 2018, 10, 18915–18919 RSC.
- M. Qu, H. Li, L.-H. Xie, S.-T. Yan, J.-R. Li, J.-H. Wang, C.-Y. Wei, Y.-W. Wu and X.-M. Zhang, J. Am. Chem. Soc., 2017, 139, 12346–12349 CrossRef CAS PubMed.
- Y. Yang, T. Jia, Y.-Z. Han, Z.-A. Nan, S.-F. Yuan, F.-L. Yang and D. Sun, Angew. Chem., Int. Ed., 2019, 58, 12280–12285 CrossRef CAS PubMed.
- F. Hu, J.-J. Li, Z.-J. Guan, S.-F. Yuan and Q.-M. Wang, Angew. Chem., Int. Ed., 2020, 59, 5312–5315 CrossRef CAS PubMed.
- Z.-G. Jiang, W.-H. Wu, B.-X. Jin, H.-M. Zeng, Z.-G. Jin and C.-H. Zhan, Nanoscale, 2022, 14, 1971–1977 RSC.
- H. Guo, X. He, C.-Q. Wan and L. Zhao, Chem. Commun., 2016, 52, 7723–7726 RSC.
- Z.-G. Jiang, K. Shi, Y.-M. Lin and Q.-M. Wang, Chem. Commun., 2014, 50, 2353–2355 RSC.
-
CrysAlisPro Version 1.171.35.19, Agilent Technologies Inc, Santa Clara, CA, USA, 2011 Search PubMed.
- O. V. Dolomanov, L. J. Bourhis, R. J. Gildea, J. A. K. Howard and H. Puschmann, J. Appl. Crystallogr., 2009, 42, 339–341 CrossRef CAS.
- L. Falivene, R. Credendino, A. Poater, A. Petta, L. Serra, R. Oliva, V. Scarano and L. Cavallo, Organometallics, 2016, 35, 2286–2293 CrossRef CAS.
- L. Falivene, Z. Cao, A. Petta, L. Serra, A. Poater, R. Oliva, V. Scarano and L. Cavallo, Nat. Chem., 2019, 11, 872–879 CrossRef CAS PubMed.
- P. R. Spackman, M. J. Turner, J. J. McKinnon, S. K. Wolff, D. J. Grimwood, D. Jayatilaka and M. A. Spackman, J. Appl. Crystallogr., 2021, 54, 1006–1011 CrossRef CAS PubMed.
-
J. F. Moulder, W. F. Stickle, W. M. Sobol and K. D. Bomben, in Handbook of X-Ray Photoelectron Spectroscopy, ed. J. Chastain, Perkin-Elmer Corporation, 1992 Search PubMed.
- R. Sui, X.-H. Zhang, X.-D. Wang, X.-Y. Wang, J.-J. Pei, Y.-F. Zhang, X.-R. Liu, W.-X. Chen, W. Zhu and Z.-B. Zhuang, Nano Res., 2022, 15, 7968–7975 CrossRef CAS.
- C.-G. Shi, J.-H. Jia, Y. Jia, G. Li and M.-L. Tong, CCS Chem., 2023, 5, 1154–1162 CrossRef CAS.
- X. Yuan and M. Zhu, Inorg. Chem. Front., 2023, 10, 3995–4007 RSC.
- R. Jin, S. Zhao, C. Liu, M. Zhou, G. Panapitiya, Y. Xing, N. L. Rosi, J. P. Lewis and R. Jin, Nanoscale, 2017, 9, 19183–19190 RSC.
- X. Zou, S. He, X. Kang, S. Chen, H. Yu, S. Jin, D. Astruc and M. Zhu, Chem. Sci., 2021, 12, 3660–3667 RSC.
- M. Cargnello, C. Chen, B. T. Diroll, V. V. T. Doan-Nguyen, R. J. Gorte and C. B. Murray, J. Am. Chem. Soc., 2015, 137, 6906–6911 CrossRef CAS PubMed.
- B. R. Camach, R. Morales-Rodriguez and A. Medina Ramírez, Int. J. Hydrogen Energy, 2016, 41, 23336–23344 CrossRef.
- Y.-S. Zhang, L.-K. Yin, Z.-H. Luo, X.-Q. Zhuge, P. Wei, Z. Song and K. Luo, Sustainable Energy Fuels, 2023, 7, 3276–3283 RSC.
Footnotes |
† Electronic supplementary information (ESI) available: Experimental and characterization details and additional figures and tables (Fig. S1–S24 and Tables S1–S3) offer more details on the nanoclusters. CCDC 2362662. For ESI and crystallographic data in CIF or other electronic format see DOI: https://doi.org/10.1039/d4sc04318a |
‡ These authors contributed equally to this work. |
|
This journal is © The Royal Society of Chemistry 2024 |
Click here to see how this site uses Cookies. View our privacy policy here.