Ti3C2Tx MXene coupled Co(OH)2: a stable electrocatalyst for the hydrogen evolution reaction in alkaline media
Received
23rd July 2024
, Accepted 18th September 2024
First published on 24th September 2024
Abstract
Green hydrogen (H2) production via water electrolysis is a promising technique. Within this domain, two dimensional (2D) materials are gaining more attention throughout the world particularly in energy conversion/storage devices due to their unique features. Herein, this study focuses on the development of sustainable, durable, and economical electrocatalysts based on titanium carbide (Ti3C2Tx) MXene and cobalt hydroxide (Co(OH)2) as a composite. Ti3C2Tx has been doped into Co(OH)2 (CT nanostructure) with varying concentrations by the aqueous chemical growth method. The as-prepared electrocatalysts (CT-15 and CT-30) have been investigated through different physicochemical characterization studies including X-ray diffraction (XRD), scanning electron microscopy (SEM), Fourier transform infrared spectroscopy (FTIR), and electrochemical analysis in order to access their morphology, crystalline phase homogeneity, surface functionalization, and electrochemical behaviour for the HER. It is observed that the as-prepared material (CT-30) exhibits superior hydrogen evolution reaction (HER) activity in 1.0 M potassium hydroxide (KOH). The optimised electrocatalyst CT-30 demonstrates an overpotential of 380 mV at a current density of 10 mA cm−2 with a 99 mV dec−1 Tafel slope value, showing fast reaction kinetics. Moreover, it offers a low charge transfer resistance (Rct) accompanied by good stability, high electrochemical active surface area (ECSA), and durability for 30 h, as evident for efficient HER activity. This novel electrocatalyst can contribute to the replacement of noble metal-based electrocatalysts for practical usage in energy conversion/storage systems.
Sustainability spotlight
Combining Ti3C2Tx (MXene) with Co(OH)2 as an electrocatalyst for the hydrogen evolution reaction (HER) in an alkaline medium improves both the effectiveness and long-term stability of the catalytic process, while also making a substantial contribution to sustainability. This sophisticated electrocatalyst contributes to the achievement of Sustainable Development Goal (SDG) 7 by facilitating the generation of economical and environmentally friendly energy via enhanced hydrogen (H2) production, which is crucial for the advancement of renewable energy technology. Additionally, by promoting cleaner energy alternatives, it also contributes to achieving Sustainable Development Goal 13 by lessening the negative effects of climate change via decreased dependence on fossil fuels and decreased emissions of greenhouse gases. Hence, the incorporation of Co(OH)2 into Ti3C2Tx as an electrocatalyst is a crucial method for attaining a long-lasting and robust energy future, directly contributing to worldwide sustainability goals.
|
1. Introduction
Demand for energy is increasing with the world population, so the annual consumption per capita is expected to increase with advancement in the human lifestyle.1 Currently, fossil fuels account for 80% of the world's total energy production. However, the combustion of these fuels releases greenhouse gases into the atmosphere, leading to adverse effects on the climate. This, in turn, causes a rise in global temperatures and poses a threat to freshwater reserves.2 It is the need of the world to invent more efficient and economical renewable energy sources.3 Hydrogen (H2) production technologies are gaining more attention as a renewable energy source in which the H2 is produced by steam reforming, thermal decomposition, biomass fermentation, and electrolysis.4–6 Among all these methods, the water electrolysis approach is more attractive due to producing H2 from water molecules by splitting it into H2 and oxygen (O2). There are two kinds of reactions that occur during the water splitting process such as the hydrogen evolution reaction (HER) and oxygen evolution reaction (OER) in the process of electrolysis.7,8 The HER and OER take place at the cathode and anode side in acidic and alkaline electrolytes, respectively. However, the HER offers more hindrances in an alkaline environment, so more electrical potential is required to carry out this reaction effectively.9,10 In order to rectify this issue, noble metal-based electrocatalysts such as platinum (Pt), ruthenium (Ru) and iridium (Ir) and their alloys, are used to facilitate this reaction at low overpotential.11,12 However, these noble catalysts are costly due to limited reserves leading to less economical output for mass scale production. Therefore, the world is focusing on alternative materials that are reliable for industrial scale H2 production.13
For this reason, transition metal (TM)-based catalysts have been developed such as sulfides, carbides,14,15 phosphides,16,17 nitrides,18 oxides,19,20 and hydroxides.21,22 From these reported catalysts, TM hydroxide-based catalysts offer good stability and efficient electrochemical performance. In addition, various metal hydride catalysts have been reported to enhance the HER activity, but cobalt-based hydroxides are most favourable due to their lowest possible reaction rate, tuneable electronic structure and morphological characteristics.23 For example, X.-Y. Zhang et al.24 reported CoFeOH encapsulated CoFeP on iron foam (CoFeOH/CoFeP/IF) for reducing phosphorus loss and enhancing its performance in alkaline media. Furthermore, X. Feng et al.25 synthesized hollow nanotubes of Ni/CoFe hydroxide and Ni/CoSe2 on nickel foam through the electrodeposition process to optimize the HER electrochemical reaction kinetics. Moreover, Zhan et al.26 effectively generated a precursor material by electrodeposition of two doping elements to create a co-doped cobalt hydroxide electrocatalyst (Ru-Co(OH)2-Se). This strategy has been shown to be an efficacious method for enhancing the performance of the HER. The test findings indicate that the material had a reduced impedance value and an increased electrochemically active surface area.
Previously, two-dimensional (2D) materials are developed and extensively used for energy storage and conversion applications.27 Among them, MXenes are a novel material, which exhibit higher electrical conductivity and tuneable surface functionality.28 MXenes can be prepared from the MAX phase having the general formula Mn+1AXn (n = 1, 2, 3), where M represents TM, A denotes the IIIA or IVA elements and X shows nitrogen or carbon.29,30 To produce MXenes, the A layer (Al, Ga, and so on) is selectively removed and terminated by Tx functional groups (–O, –OH and –F). Mostly Ti3C2Tx MXene is widely used in H2 generation applications because of superior metallic conductivity and hydrophilicity.31,32 Various thin layered MXene-based electrocatalysts have been recently reported in the literature for HER activity such as 1T/2H MoS2 (25D)/Ti3C2Tx −1,33 Fe/Ni doped GO@Ti3C2Tx,34 MoS2/Ti3C2 (ref. 35), etc. Notwithstanding MXene's outstanding performance, a few issues remain, including low capacitance, weaker surface functional groups, unreacted microstructure, and restacking. Different approaches, including doping, surface modification, morphological control, and their hybrid composites with other TM materials, can be used to overcome these issues and improve the efficiency of energy conversion and storage devices.35 In general, the doped MXene shows an increase in interlayer space and electrical conductivity. Selecting highly conductive carbon materials to couple with cobalt oxide, such as graphene, carbon nanotubes, and MXenes, has already been shown to be an effective way to increase catalytic behaviour by improving electrical conductivity.36 Typically, carbon acts as a supporting matrix in various nanocomposites, boosting conductivity, preventing composite material aggregation, and increasing the material's specific surface area.37
In this study, a promising composite of Ti3C2Tx and Co(OH)2 has been prepared through the aqueous chemical growth method, wherein Ti3C2Tx is doped into Co(OH)2 nanostructures with varying concentrations such as 15 and 30 mg (CT-15 and CT-30). The structural, compositional, and morphological characteristics were also examined using various physicochemical techniques. The as-prepared composites along with pristine materials were analysed for HER activity in 1.0 M KOH. The optimised composite CT-30 exhibits superior electrochemical performance with an overpotential of 380 mV at 10 mA cm−2 current density, a Tafel slope of 99 mV dec−1, and stability for 30 h. This is an entirely new approach and has not been reported before (Fig. 1).
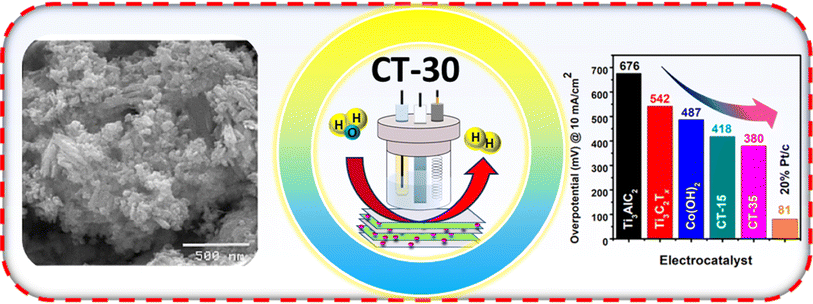 |
| Fig. 1 The schematic illustration of this research study. | |
2. Materials and methods
2.1 Materials
Cobalt chloride hexahydrate (CoCl2·6H2O), urea (CH4N2O), deionized (DI) water, MAX phase (Ti3AlC2), 5 wt% Nafion, potassium hydroxide (KOH), hydrochloric acid (HCl) and hydrofluoric acid (HF) were bought from Sigma Aldrich in Karachi, Pakistan. The tests used high-grade analytical substances without purification.
2.2 Synthesis method
The composite of Ti3C2Tx and Co(OH)2 was synthesized in two stages which include preparation of the MXene and its composite with Co(OH)2. Initially, the preparation of Ti3C2Tx (Tx = –F) MXene was performed by the HF + HCl etching method, wherein the layer of Al was removed from the Ti3AlC2 MAX phase. Here, 0.5 g of bulk MAX was progressively introduced in a Teflon lined beaker having 60 mL of 20% aqueous HF + 5% HCl. The resultant Ti3AlC2 powder was agitated continuously at room temperature for 40 h using a Teflon-lined magnetic bar. The etching/removal of Al layers from the MAX precursor was then conducted. For removal of the excess acidity of solution, HF acidic solutions were centrifuged multiple times with D.I. water until their pH reaches ∼7. Ti3C2Tx was collected and dried in a vacuum oven (to avoid oxidation).
Secondly, the formation of the Ti3C2Tx and Co(OH)2 composite was conducted by the aqueous chemical growth method. Typically, 15 mg and 30 mg of prepared Ti3C2Tx MXene were added individually into precursors of Co(OH)2 and respectively labelled as CT-15 and CT-30. The precursors of Co(OH)2 contained 0.1 M of CoCl2·6H2O and 0.1 M of CH4N2O with the addition of Ti3C2Tx. The precursor solution was continually stirred on a magnetic stirrer until a homogenous solution was obtained and then placed in an electric oven at 95 °C for 5 h. After completing the growth time interval, the prepared composite was washed with DI water multiple times to remove other impurities. The samples were dried at room temperature and collected in sample vials. In addition, pristine Co(OH)2 was also synthesized similarly by following the above procedure (Fig. 2). Hence, as-synthesized Ti3C2Tx, Co(OH)2, and Ti3C2Tx doped Co(OH)2 composites were ready to be characterized and optimized for electrochemical analysis.
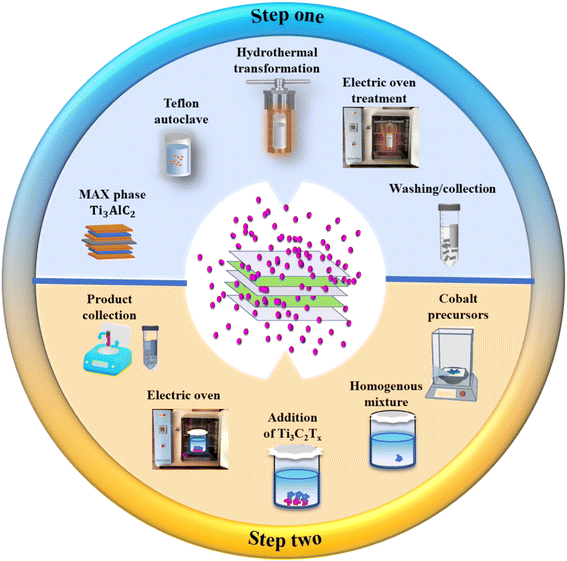 |
| Fig. 2 Schematic illustration of the preparation of the Ti3C2Tx MXene coupled Co(OH)2 electrocatalyst. | |
2.3 Characterization
The X-ray diffraction (XRD) technique was conducted using a Philips PAN analytical X-ray diffractometer to evaluate the crystal orientation and phase purity of the prepared nanostructures. The operating parameters were kept as 45 kV voltage, 45 mA current and a CuKa radiation source with 1.5418 Å wavelength. The obtained X-ray spectra were quantified via High Score Plus software. Scanning electron microscopy (SEM) was performed using a JEOL JSM-6480A for morphological analysis of synthesized materials at an operating voltage of 20 kV. In addition, the surface functionalization of the samples was investigated by Fourier transform infrared (FTIR) spectroscopy using a PerkinElmer's Spectrum 2 instrument. A concentration of 0.5 wt% of the samples was mixed with dried KBr for each sample measurement and the operating range was kept within 400 to 4000 cm−1.
2.4 Electrochemical measurement
The electrochemical measurement for the HER was performed via a VERSASTAT4-500 containing a three electrode cell assembly. The cell assembly consists of a reference electrode (silver/silver chloride), counter electrode (Pt wire), working electrode (modified glassy carbon electrode (GCE)) and electrolyte (1.0 M KOH). The catalyst ink was used to modify the GCE. It consists of 5 mg as-synthesized catalyst, 30 μL of 5% Nafion solution and 5 mL DI water. Each catalyst ink was properly dispersed through an ultrasonic bath for 20 min. The obtained homogeneous ink was drop cast onto the cleaned surface of the GCE, and air was blown for drying. The dried GCE was placed into the cell assembly for electrochemical measurements.
HER polarization curves were obtained by linear sweep voltammetry (LSV) in 1.0 M KOH at a scan rate of 5 mV s−1. The chrono-potentiometric test was used to determine the durability of the best sample at 10 mA cm−2 and 20 mA cm−2 current densities for 30 h. The electrochemical impedance spectroscopy (EIS) technique was performed for examination of the charge transfer resistance (Rct) of various synthesized catalysts. It was operated at −0.4 V vs. RHE with 5 mV sinusoidal potential in the range of 100
000 Hz to 1 Hz frequency. Electrochemical active surface area (ECSA) of the samples was determined via the cyclic voltammetry (CV) technique, performed at various scan rates (30, 50, 70, 90, 110 and 130 mV s−1) in the non-faradaic region. At last, the obtained potential data were converted into the reversible hydrogen electrode (RHE) through the Nernst equation:
The EAg/AgCl value is 0.197, while the overpotential (η) is calculated by subtracting the water splitting system's onset thermodynamic potential of 0 V for the HER.
Overpotential (η) = onset potential (ERHE) V − 0 V |
The Tafel formula has been employed to determine the Tafel slope.
η = b log j + a |
η represents the overpotential,
b stands for the Tafel slope,
j denotes the current density, and
a represents the point of the intersection parameter or exchange current density.
38
3. Results and discussion
3.1 Composition and structural analysis
Fig. 3 displays the distinct peak pattern of MAX powder (Ti3AlC2). It can be seen that the XRD patterns of pristine MAX powder has well-matched patterns with reference JCPDS no. 520-875 along with sharp and broader peaks at around 9.28°, 19.15°, 33.71°, 34.04°, 38.81°, 39.04°, 41.81°, 44.92°, 48.54°, 56.57° and 60.25° which correspond to the (002), (004), (100), (101), (008), (104), (105), (106), (107), (109) and (110) crystallographic planes.39,40 The XRD diffraction pattern of MXenes shows a major peak at 7.22° corresponding to the (002) plane and minor peaks at 14.4° and 29° which correspond to the (004) and (006) planes, also in good correlation with the literature.41
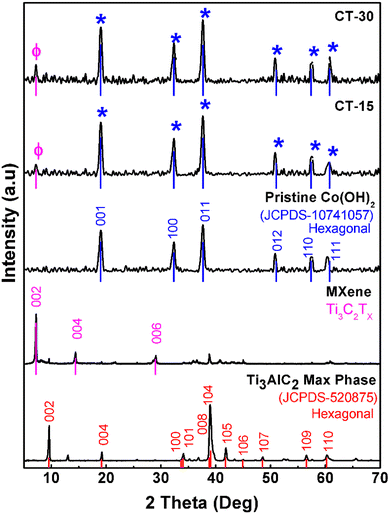 |
| Fig. 3 XRD diffraction of Ti3AlC2(MAX), Ti3C2Tx (MXene), Co(OH)2, CT-15 and CT-30. | |
After HF + HCl exfoliation treatment, there was an impressive shift in the (002) peak from 2θ 9.26° to 7.22° suggesting the increase in the c lattice parameter. On the other hand, pristine Co(OH)2 has broader peaks at 19.02°, 32.35°, 37.69°, 51.03°, 57.42° and 60.81° which correspond to the (001), (100), (011), (012), (11 0) and (111) crystallographic planes, which is well-matched with reference JCPDS no. 10741057 and with some reported research studies as well.42,43 The MXene/Co(OH)2 composite possessed nearly all the specific peaks of MXenes and Co(OH)2, which confirmed the successful synthesis of the desired composite material. Besides, a little shift has been observed after composite formation, and this change may be attributed to the introduction of MXenes into Co(OH)2 nanostructures as well as the variation of lattice parameters in the final crystal structure.44
The morphology and structural features of Ti3AlC2, exfoliated MXene, Co(OH)2 and the composite CTs were studied by SEM analysis. The uneven surface and the closely packed structure of the MAX phase are shown in Fig. 4(a).45 The exfoliated flake like morphology of MXenes was obtained after the HF + HCl treatment of the MAX phase (Fig. 4(b)).46 Pristine Co(OH)2 demonstrated an agglomerated spherical particle like morphology as shown in Fig. 4(c). However, composites (CT-15 and CT-30) show the growth of a spherical particle layer on MXene flakes as a combination of both structural features. Through SEM analysis (Fig. 4(d and e)), we have confirmed our successful synthesis of MXene integrated Co(OH)2 materials based on the provided literature.47
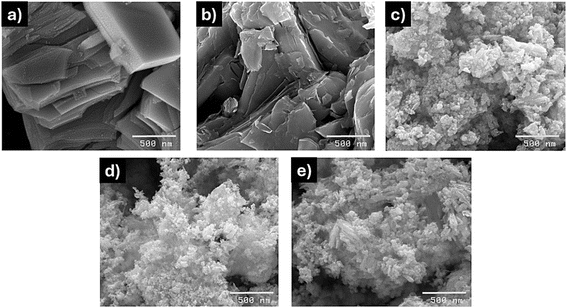 |
| Fig. 4 SEM images: (a) Ti3AlC2 (MAX phase), (b) Ti3C2Tx (MXene), (c) Co(OH)2 and (d and e) CT-15 and CT-30. | |
The surface functionalization of synthesized materials has been conducted through FTIR spectroscopy. The FTIR spectra of Ti3AlC2 (MAX), Ti3C2Tx (MXene), Co(OH)2, CT-15 and CT-30 are shown in Fig. 5. It is observed that all materials revealed some common bands such as at 3438, 2930, 2854, 1601 and 1382 cm−1 assigned to (O–H), (C–H), (C–H), (O–H) and (C–H), respectively. The O–H groups are present due the absorption of external water H2O molecules on the surface of materials because of their hydrophilic nature. The presence of the C–H band in materials is attributed to symmetrical/asymmetrical methylene (CH2) and methyl (CH3) stretching.46,48 Furthermore, the FTIR spectra of the Ti3AlC2 phase probably represent Ti–O–Ti and Ti–O deformation vibration linkage bonding at 879 and 605 cm−1. Moreover, the peak at 481 cm−1 represents the rocking or twisting vibration of Ti–C. However, the Ti3C2Tx generated fluorine-terminated band (C–F) at 1108 cm−1 and sharpness of the Ti–C band confirm the etching of the Ti3AlC2 MAX phase. The FTIR spectra of Co(OH)2 showed the O–H and Co–O bands at 3540 and 425 cm−1 which validate the formation of the cobalt hydroxide phase.49,50 Despite of composite CT-15 and CT-30 exhibit the stretching and vibration band related to Ti3C2Tx and Co(OH)2 such as C–F, Ti–O–Ti, Ti–O–TiC, and Co–O bands.51,52 It is also observed that the vibration band of Co–O is reduced with the addition of a higher amount of Ti3C2Tx in CT-30. Hence, this confirms the presence of Ti3C2Tx in Co(OH2) nanostructures.
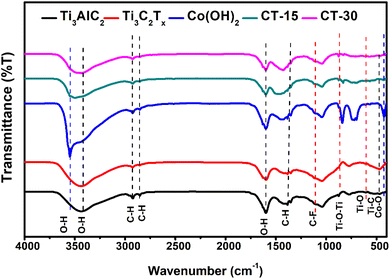 |
| Fig. 5 FTIR spectra of Ti3AlC2 (MAX phase), Ti3C2Tx (MXene), Co(OH)2, CT-15 and CT-30. | |
3.2 Electrochemical investigation
The electrochemical analysis was performed on Ti3AlC2, Ti3C2Tx, Co (OH)2, CT-15 and CT-30 as shown in Fig. 6. These electrocatalysts were analysed for HER study through LSV in 1.0 KOH electrolyte, as presented in Fig. 6(a). The polarization curves reveal that Ti3AlC2 has an overpotential value of 676 mV whereas Ti3C2Tx and Co (OH)2 have shown lower overpotential values of 542 mV and 487 mV at a current density of 10 mA cm−2. In contrast, CT-15 and CT-30 have shown much better HER activity compared to their counterparts with the overpotential values of 418 mV and 380 mV at a current density of 10 mA cm−2, which is nearer to that of 20% Pt/C as a reference. The overpotential values corresponding to each catalyst can be observed via a histogram as shown in Fig. 6(b). According to comparative analysis with reported work mentioned in Table 1 this presented work is superior as compared to to previous reports. The relationship between the reaction rate and overpotential applied provides information about the electrochemical performance of electrocatalysts in terms of the Tafel slope which can be seen in Fig. 6(c). It is evident that CT-30 exhibits a Tafel slope value of 99 mV dec−1, which is lower than the values observed for the other analysed samples, namely Ti3AlC2, Ti3C2Tx, Co(OH)2, and CT-15, which have Tafel slope values of 214, 170, 147, and 112 mV dec−1, respectively. In addition, 20% Pt/C has the lowest Tafel slope value of 37 mV dec−1, which is nearer to that of CT-30 as compared to other as-prepared electrocatalysts. In an alkaline environment, the kinetics of the HER can be described by three sequential steps, as outlined in the equations. | H2O + e− + M → MHads + OH− (Volmer step) | (1) |
| MHads + MHads → H2 + 2M (Tafel step) | (2) |
| H2O + MHads + e− → H2 + M + OH− (Heyrovsky step) | (3) |
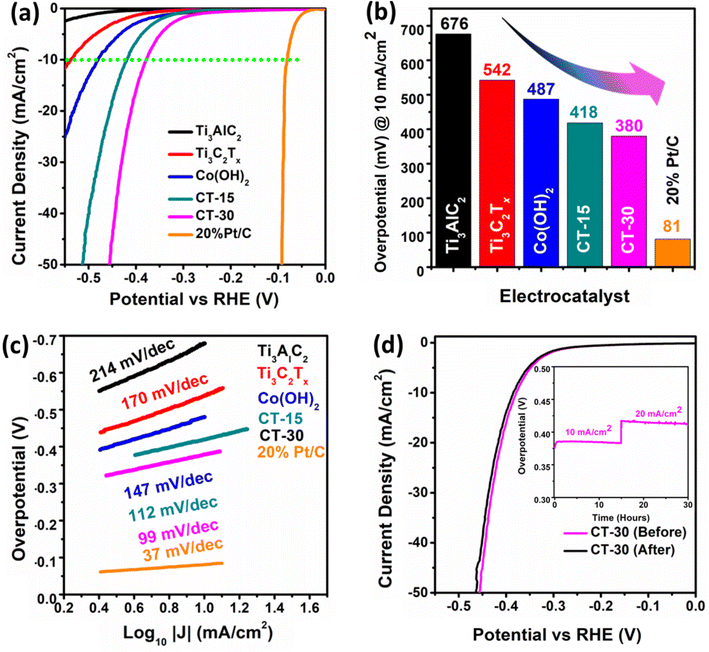 |
| Fig. 6 (a) Linear Scan Voltammetry (LSV) of different catalysts with iR corrected data, (b) histogram representing overpotential of the prepared electrocatalysts, (c) corresponding Tafel slope values generated from LSV and (d) LSV measurement before and after the durability test; (inset) chronopotentiometric durability. | |
Table 1 Comparative analysis of the presented work with previous reported work
Electrocatalyst |
Electrolyte |
Current density |
Overpotential |
Tafel slope |
Ref. |
CT-30
|
1 M KOH
|
10 mA cm−
2
|
380 mV
|
99 mV dec
−1
|
This work
|
Fe50Ni50 |
1 M NaOH |
10 mA cm−2 |
390 mV |
96 mV dec−1 |
53
|
MCO3 |
1 M KOH |
10 mA cm−2 |
400 mV |
174 mV dec−1 |
54
|
MoS2/Ti3C2 |
0.5 M H2SO4 |
10 mA cm−2 |
400 mV |
74 mV dec−1 |
55
|
HC-MoS2/MoC2 |
1 M KOH |
1000 mA cm−2 |
412 mV |
60 mV dec−1 |
56
|
BP Ir Sur |
1 M KOH |
10 mA cm−2 |
420 mV |
50.4 mV dec−1 |
57
|
HF-MXene |
1 M KOH |
10 mA cm−2 |
444 mV |
311 mV dec−1 |
58
|
N,P,O doped GC |
1 M KOH |
10 mA cm−2 |
446 mV |
154 mV dec−1 |
59
|
Pt 3D Lig |
1 M KOH |
10 mA cm−2 |
455 mV |
73.2 mV dec−1 |
60
|
Fe/Ni doped GO@MXene |
0.5 M H2SO |
10 mA cm−2 |
470 mV |
110 mV dec−1 |
34
|
Co3+-Ti2CTx |
1 M KOH |
10 |
460 |
103 |
61
|
Nb4C3T |
1 M KOH |
10 |
398 |
122 |
62
|
According to eqn (1), the initial phase is associated with the Volmer step, which involves the adsorption of protons onto the surface of the electrode. Subsequently, the attached intermediate components merge, as illustrated in eqn (2) or the Heyrovsky step, which involves the absorption of the adsorbed substances as stated in eqn (3). MHads is produced on the catalytically active site in the Volmer step as a result of reduction of the proton or H2O.63 There are two potential primary pathways to enter the second phase. The inclusion of two adsorbed hydrogen atoms (MHads) in the Tafel process could lead to the formation of H2.64 The Heyrovsky step represents another mechanism where MHads could combine with a molecule of H2O and an extra electron to generate H2. The most common approach to express the difficulty of initiating a reaction over a catalyst is by referring to the Gibbs free energy of hydrogen adsorption, typically abbreviated as ΔGH*, which quantifies the strength of the binding of H* to the surface of the electrocatalyst.65 The Gibbs free energy of H2 adsorption (ΔGH*) is commonly employed to assess the energy barrier associated with initiating a reaction on a catalyst. This parameter quantifies the extent of binding strength between H2 species (H*) and the surface of the electrocatalyst. The suitability for the subject of interest is evident in the case of the HER, as the expected value of ΔGH* approaches zero, suggesting a state of equilibrium among the adsorption as well as desorption phenomena. The Heyrovsky step subsequently depicts the HER mechanism on the CT-30 composite.
The durability of the electrocatalyst CT-30 has also been conducted before and after the chronopotentiometry test Fig. 6(d). The stability of an electrocatalyst plays a pivotal role in real-time applications.66 Hence, the CT-30 composite material has been examined for stability using chronopotentiometry at current densities of 10 and 20 mA cm−2 over a duration of 30 h, thereby validating its long-term electrochemical performance. It is noteworthy that the proposed electrocatalyst has demonstrated stability, except for minor potential losses or fluctuations observed within the graph as shown in Fig. 6d (inset). The LSV polarization of CT-30 demonstrates that the electrocatalyst is well-stable in nature with some minor fluctuations in its curve.
The Rct of various electrocatalysts has been evaluated using EIS in a 1 M KOH medium. The Nyquist and Bode (I and II) plots are demonstrated in Fig. 7. Compared to Ti3AlC2, Ti3C2Tx, Co (OH)2, and CT-15, the material CT-30 has exhibited an improved phase angle. Additionally, the Rct of Ti3AlC2, Ti3C2Tx, Co(OH)2, CT-15, and CT-30 has been calculated to be 2825, 1705, 1167, 878, and 563.1 Ω. According to the data presented in Table 2 CT-30 has exhibited low Rct which indicates a higher electrical conductivity that provides better electrochemical performance as compared to its counterparts.
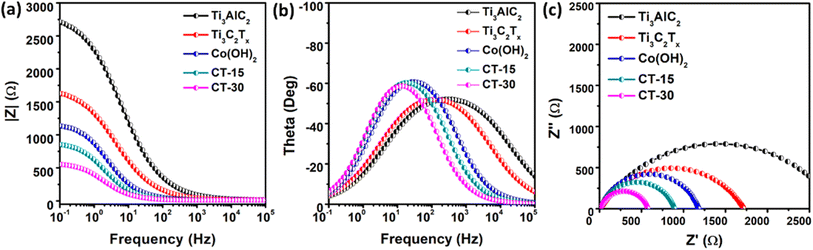 |
| Fig. 7 Electrochemical impedance spectroscopy data of Ti3AlC2, Ti3C2Tx, Co(OH)2, CT-15 and CT-30; (a & b) Bode plots (I and II); (c) Nyquist plot. | |
Table 2 Summarized HER based electrochemical features of the prepared catalysts
Catalyst |
Calculated from LSV |
Calculated from EIS |
Calculated from CV |
Overpotential |
Tafel slope |
Charge transfer resistance |
Double layer capacitance |
Double layer capacitance |
Electrochemically active surface area |
η
10
|
B
|
R
ct
|
CPEdl |
C
dl
|
ECSA |
mV |
mV dec−1 |
Ω |
mF |
μF cm−2 |
cm2 |
Ti3AlC2 |
676 |
214 |
2825 |
0.01 |
0.9 |
22.5 |
Ti3C2Tx |
542 |
170 |
1705 |
0.03 |
1.4 |
35 |
Co(OH)2 |
487 |
147 |
1167 |
0.08 |
4.8 |
120 |
CT-15 |
418 |
112 |
878 |
0.13 |
5.4 |
135 |
CT-30 |
380 |
99 |
563.1 |
0.22 |
9.2 |
230 |
Furthermore, CV has also been utilized to investigate the double-layer capacitance (Cdl) within the non-faradaic region of the as-prepared materials as seen in Fig. 8(a–e). Various scan rates, including 30, 50, 70, 90, 110, and 130 mV s−1, were employed for this analysis. Corresponding Cdl values are calculated to be 0.9, 1.4, 4.8, 5.4, and 9.2 μF cm−2 for Ti3AlC2, Ti3C2Tx, Co (OH)2, CT-15, and CT-30, respectively (Fig. 8(f)). Moreover, the electrochemical active surface area (ECSA) has been calculated through Cdl values through the given formula:
|  | (4) |
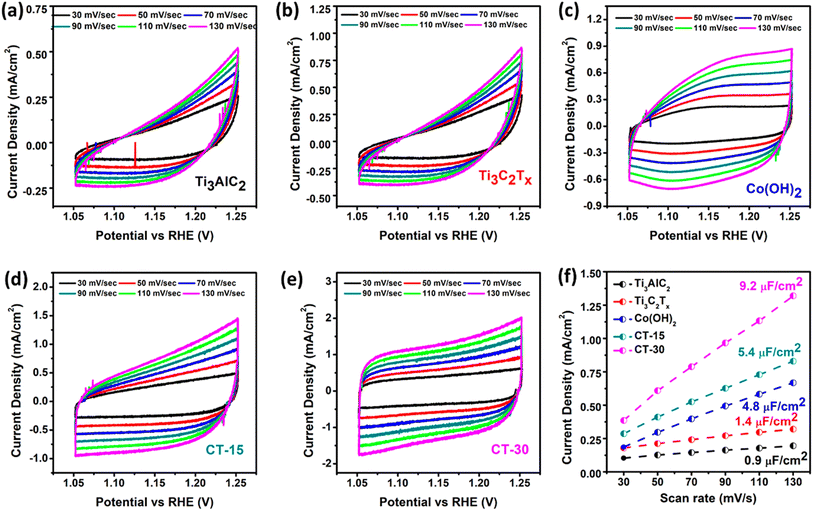 |
| Fig. 8 Cyclic voltammetry data (a–e) of Ti3AlC2, Ti3C2Tx, Co(OH)2, CT-15 and CT-30 and (f) double layer capacitance data of the prepared catalyst extracted from CV data. | |
In the given expression (4), Cdl represents the double-layer capacitance, while Cs is regarded as the specific capacitance at the electrolyte interface. Notably, the value of Cs is 0.04 mF cm−2 for KOH. The corresponding values of ECSA for Ti3AlC2, Ti3C2Tx, Co (OH)2, CT-15, and CT-30 have been calculated to be 22.5, 35, 120, 135, and 230 cm2. The higher ECSA value of CT-30 may be attributed to the synergistic effect of two distinct materials with more active sites.67
The LSV polarization data are normalized via ECSA to minimize the effect of electrochemical surface area on the HER activity as shown in Fig. 9(a). The normalized curve of CT-30 shows superior HER performance as compared to CT-15, Co (OH)2, Ti3C2Tx, and Ti3AlC2. It is revealed that CT-30 has improved intrinsic activity. Furthermore, the turnover frequency (TOF) is also determined for assessing the actual performance of the prepared catalyst using the following formula.
| 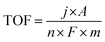 | (5) |
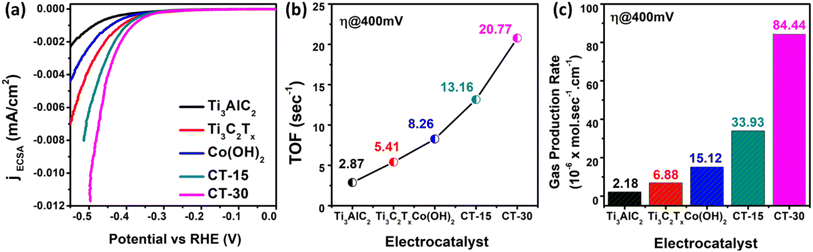 |
| Fig. 9 (a) ECSA normalized LSV curve, (b) TOFs at 400 mV overpotential and (c) theoretical gas production rate at a fixed overpotential of 400 mV of various catalysts. | |
In the above eqn (5), TOF is the turnover frequency (s−1), j represents the current density obtained at a specific overpotential, A is the electrode area, n is the number of electrons in the reaction, F represents the Faraday constant, and m denotes the number of moles of the active deposited material. The TOF of various electrocatalysts is calculated at a fixed overpotential of 400 mV as given in Fig. 9(b). The current densities and corresponding TOFs of Ti3AlC2, Ti3C2Tx, Co (OH)2, CT-15, and CT-30 are (0.42 mA cm−2 and 2.87 s−1), (1.32 mA cm−2 and 5.41 s−1), (2.91 mA cm−2 and 8.26 s−1), (6.54 mA cm−2 and 13.16 s−1) and (16.30 mA cm−2 and 20.77 s−1) respectively. It can be suggested that CT-30 can significantly outperform in industrial applications. Due to this fact, theoretical hydrogen production is also determined to validate the gas production rate of the electrocatalyst. The theoretical gas production is calculated using the given equation.
|  | (6) |
Here, in
eqn (6),
r is the gas production rate (mol s
−1 cm
−1),
i is the recorded current density at a specific overpotential,
n is the electron transfer number (
n = 2 in the HER), and
F is the Faraday constant (96
![[thin space (1/6-em)]](https://www.rsc.org/images/entities/char_2009.gif)
485.3 s A mol
−1). The hydrogen gas production rate of various catalysts can be seen in
Fig. 9(c). The result revealed that CT-30 has a higher hydrogen production rate
i.e., 84.44 × 10
−6 mol s
−1 cm
−1 as compared to others. Such an improvement could have enhanced the performance of this composition, making it promising for HER activity in KOH media. Various electrochemical characteristic features of as-prepared materials are illustrated in
Table 2. Conclusively, the material CT-30 exhibited robust electrochemical performance and has been compared with recent studies.
4. Conclusion
In summary, the presented electrocatalysts were effectively prepared by the aqueous chemical growth method. The 2D layered MXene T3C2Tx was introduced into the matrix of cobalt hydroxide Co(OH)2 nanostructures for the preparation of their composites. The physicochemical characteristics of the as-synthesized composites along with their pristine samples were analysed via various analytical techniques. X-ray diffraction (XRD) and Fourier transform infrared spectroscopy (FTIR) analyses were carried out to confirm the successful preparation of the as-prepared samples including pristine Ti3AlC2, Ti3C2Tx, Co (OH)2 samples, and CT-15, and CT-30 composites. Scanning electron microscopy (SEM) was also carried out for all as-prepared samples to confirm their morphology. Composite CT-30 shows the lowest overpotential of 380 mV at 10 mA cm−2 current density, among pristine samples and other composites in alkaline electrolyte. In addition, the low Tafel value of CT-30 of about 99 mV dec−1 further optimized HER activity. It is perceived that the CT-30 catalyst is durable and stable at 10 mA cm−2 and 20 mA cm−2 current densities for 30 h without any major drop in the potential value. A low charge transfer resistance (Rct) of 563.1 Ω, higher double layer capacitance (Cdl) value of 9.2 μF cm−2 and greater electrochemical active surface area (ECSA) of 230 cm2 are achieved because the thin layered structure of Ti3C2Tx facilitates easy transport of charges into Co(OH)2 nanostructures. Hence, it enhances the robustness of the HER that is beneficial for energy production and storage applications for sustainable society.
Data availability
The data will be available on request.
Conflicts of interest
The authors of this paper have acknowledged no conflicts of interest.
Acknowledgements
The authors would like to acknowledge the Researcher's Supporting Project Number (RSP2024R269) King Saud University, Riyadh, Saudi Arabia.
References
- R. Badrnezhad and et al,
., Effect of iron on Ni–Mo–Fe composite as a low-cost bifunctional electrocatalyst for overall water splitting, Int. J. Hydrogen Energy, 2021, 46(5), 3821–3832 CrossRef CAS.
- Y. Xiao, Nanoclusters for photoelectrochemical water splitting: bridging the photosensitizer and carrier transporter, EcoEnergy, 2023, 1(1), 60–84 CrossRef.
- J. Ren and et al,
., Modulating amorphous/crystalline heterogeneous interface in RuCoMoyOx grown on nickel foam to achieve efficient overall water splitting, Chem. Eng. J., 2023, 469, 143993 CrossRef CAS.
- U. Aftab,
et al., Mixed CoS2@Co3O4 composite material: an efficient nonprecious electrocatalyst for hydrogen evolution reaction, Int. J. Hydrogen Energy, 2020, 45(27), 13805–13813 CrossRef CAS.
- A. Hanan,
et al., Co2FeO4@rGO composite: towards trifunctional water splitting in alkaline media, Int. J. Hydrogen Energy, 2022, 47(80), 33919–33937 CrossRef CAS.
- A. Pareek,
et al., Insights into renewable hydrogen energy: recent advances and prospects, Mater. Sci. Energy Technol., 2020, 3, 319–327 CAS.
- A. J. Laghari and et al,
., MgO as promoter for electrocatalytic activities of Co3O4–MgO composite via abundant oxygen vacancies and Co2+ ions towards oxygen evolution reaction, Int. J. Hydrogen Energy, 2023, 48(34), 12672–12682 CrossRef CAS.
-
P. Banoth, C. Kandula and P. Kollu, Introduction to Electrocatalysts, in Noble Metal-free Electrocatalysts: New Trends in Electrocatalysts for Energy Applications, American Chemical Society, 2022, vol. 2, p. 1–37 Search PubMed.
- Z. H. Ibupoto, NiCo2O4 nanostructures loaded onto pencil graphite rod: an advanced composite material for oxygen evolution reaction, Int. J. Hydrogen Energy, 2022, 47(10), 6650–6665 CrossRef CAS.
- J. Liu, V-Doping Strategy Induces the Construction of the Functionally Complementary Ru2P/V-RuP4 Heterostructures to Achieve Amperometric Current Density for HER, Adv. Funct. Mater., 2024, 34(28), 2315773 CrossRef CAS.
- W. Li and et al,
., Defective RuO2/TiO2 nano-heterostructure advances hydrogen production by electrochemical water splitting, Chem. Eng. J., 2022, 431, 134072 CrossRef CAS.
- Y. Du, Recent advances in interface engineering strategy for highly-efficient electrocatalytic water splitting, InfoMat, 2023, 5(1), e12377 CrossRef CAS.
- N. T. T. Thao, Current Trends of Iridium-Based Catalysts for Oxygen Evolution Reaction in Acidic Water Electrolysis, Small Sci., 2024, 4(1), 2300109 CrossRef CAS.
- A. Das,
et al., Zinc doping induced WS2 accelerating the HER and ORR kinetics: a theoretical and, Catal. Today, 2023, 423, 113921 CrossRef CAS.
- M. Smialkowski and et al,
., Trimetallic Pentlandites (Fe,Co,Ni)9S8 for the Electrocatalytical HER in Acidic Media, ACS Mater. Au, 2022, 2(4), 474–481 CrossRef CAS PubMed.
- Y. J. Bai and et al,
., Tunable and Specific Formation of C@NiCoP Peapods with Enhanced HER Activity and Lithium Storage Performance, Chem.–Eur. J., 2016, 22(3), 1021–1029 CrossRef CAS PubMed.
- B. T. Jebaslinhepzybai and et al,
., One-pot solvothermal synthesis of Co2P nanoparticles: an efficient HER and OER electrocatalysts, Int. J. Hydrogen Energy, 2021, 46(42), 21924–21938 CrossRef CAS.
- C. J. Rupp, J. Anversa and R. J. Baierle, First principles of Si-doped BC2N single layer for hydrogen evolution reaction (HER), Int. J. Hydrogen Energy, 2023, 48(20), 7294–7304 CrossRef CAS.
- J. Verma and S. Goel, Cost-effective electrocatalysts for Hydrogen Evolution Reactions (HER): Challenges and Prospects, Int. J. Hydrogen Energy, 2022, 47(92), 38964–38982 CrossRef CAS.
- T. T. Wang and et al,
., Nanoframes of Co3O4-Mo2N Heterointerfaces Enable High-Performance Bifunctionality toward Both Electrocatalytic HER and OER, Adv. Funct. Mater., 2022, 32(7), 2107382 CrossRef CAS.
- S. T. Huang and et al,
., Ru-promoted NiFe oxyhydroxide anchored on the hierarchical porous N-doped carbon aerogel: electronic structures modulation for much enhanced OER/HER dual-functional characteristics, Appl. Catal., A, 2023, 664, 119331 CrossRef CAS.
- S. N. Lin and et al,
., The Mechanism of One-Step Synthesis of Oxides from Metal Chlorides and Their Electrocatalytic HER Study, Cryst. Res. Technol., 2022, 57(8), 2200008 CrossRef CAS.
- H. H. Do and et al,
., Cobalt compounds-based hollow structure electrocatalysts for water splitting: a review, Int.
J. Hydrogen Energy, 2024, 49, 613–632 CrossRef CAS.
- X.-Y. Zhang and et al,
., Amorphous-crystalline catalytic interface of CoFeOH/CoFeP with double sites based on ultrafast hydrolysis for hydrogen evolution at high current density, J. Power Sources, 2021, 507, 230279 CrossRef CAS.
- X. Feng and et al,
., Construction of hierarchical nickel/cobalt iron-hydroxide and nickel/cobalt selenide nanotubes for efficient electrocatalytic water splitting, New J. Chem., 2020, 44(18), 7552–7560 RSC.
- W. Peng and et al, Ru and Se Co-Doped Cobalt Hydroxide Electrocatalyst for Efficient Hydrogen Evolution Reactions, Molecules, 2023, 28(15), 5736 CrossRef CAS PubMed.
- A. Hanan and et al,
., MXenes and heterostructures-based electrocatalysts for hydrogen evolution reaction: recent developments and future outlook, J. Energy Chem., 2024, 92, 176–206 CrossRef CAS.
- M. Rahman and M. S. Al Mamun, Future prospects of MXenes: synthesis, functionalization, properties, and application in field effect transistors, Nanoscale Adv., 2024, 6(2), 367–385 RSC.
- S. B. Devi and R. Navamathavan, One-Pot Hydrothermal Synthesis of Ti3C2 (MXene)-CoS2 Nanocomposite as a Bifunctional Electrocatalyst (HER/OER) for a Clean Environment, J. Electrochem. Soc., 2023, 170(9), 096503 CrossRef CAS.
- D. Liu and et al,
., Nitrogen-Doped MoS2/Ti3C2Tx Heterostructures as Ultra-Efficient Alkaline HER Electrocatalysts, Inorg. Chem., 2021, 60(13), 9932–9940 CrossRef CAS PubMed.
- L. P. Hao, Synergistic Integration of MXene and Metal-Organic Frameworks for Enhanced Electrocatalytic Hydrogen Evolution in an Alkaline Environment, Catalysts, 2023, 13(5), 802 CrossRef CAS.
- N. H. Attanayake and et al,
., Vertically aligned MoS2 on Ti3C2 (MXene) as an improved HER catalyst, J. Mater. Chem. A, 2018, 6(35), 16882–16889 RSC.
- J. Y. Loh, F. M. Yap and W. J. Ong, 2D/2D heterojunction interface: Engineering of 1T/2H MoS2 coupled with Ti3C2Tx heterostructured electrocatalysts for pH-universal hydrogen evolution, J. Mater. Sci. Technol., 2024, 179, 86–97 CrossRef CAS.
- S. K. Raj and et al,
., Three-dimensional Ni/Fe doped graphene oxide@MXene architecture as an efficient water splitting electrocatalyst, Int. J. Hydrogen Energy, 2022, 47(99), 41772–41782 CrossRef CAS.
- Z. Z. Xu and et al,
., Facile fabrication of carbon fiber skeleton structure of MoS2 supported on 2D MXene composite with highly efficient and stable hydrogen evolution reaction, Compos. Sci. Technol., 2022, 222 Search PubMed.
- L. Ma and et al,
., CoP nanoparticles deposited on reduced graphene oxide sheets as an active electrocatalyst for the hydrogen evolution reaction, J. Mater. Chem. A, 2015, 3(10), 5337–5343 RSC.
- T. A. Le,
et al., Porosity-Engineering of MXene as a Support Material for a Highly Efficient Electrocatalyst toward Overall Water Splitting, ChemSusChem, 2020, 13(5), 945–955 CrossRef CAS PubMed.
- M. Y. Solangi and et al,
., In-situ growth of nonstoichiometric CrO0.87 and Co3O4 hybrid system for the enhanced electrocatalytic water splitting in alkaline media, Int. J. Hydrogen Energy, 2023, 36439–36451 CrossRef CAS.
- Z. Mahmoudi,
et al., Synthesis of Ti2AlC & Ti3AlC2 MAX phases by Arc-PVD using Ti–Al target in C2H2/Ar gas mixture and subsequent annealing, Ceram. Int., 2020, 46(4), 4968–4975 CrossRef CAS.
- V. I. Vershinnikov and D. Y. Kovalev, Preparation of Ti2AlC and Ti3AlC2 MAX Phases by Self-Propagating High-Temperature Synthesis with the Reduction Stage, Russ. J. Non-Ferrous Metals, 2020, 61(5), 554–558 CrossRef.
- R. R. Raja Sulaiman, Structurally modified MXenes-based catalysts for application in hydrogen evolution reaction: a review, Catalysts, 2022, 12(12), 1576 CrossRef CAS.
- J. Zhu and et al,
., Composites of TiO2 Nanoparticles Deposited on Ti3C2 MXene Nanosheets with Enhanced Electrochemical Performance, J. Electrochem. Soc., 2016, 163(5), A785 CrossRef CAS.
- A. Tariq and et al,
., Efficient Visible-Light Photocatalysis of 2D-MXene Nanohybrids with Gd3+- and Sn4+-Codoped Bismuth Ferrite, ACS Omega, 2018, 3(10), 13828–13836 CrossRef CAS PubMed.
- M. Mahmood and et al,
., Synthesis of Ultrathin MnO2 Nanowire-Intercalated 2D-MXenes for High-Performance Hybrid Supercapacitors, Energy Fuels, 2021, 35(4), 3469–3478 CrossRef CAS.
- S. Munir and et al,
., Exploring the Influence of Critical Parameters for the Effective Synthesis
of High-Quality 2D MXene, ACS Omega, 2020, 5(41), 26845–26854 CrossRef CAS PubMed.
- X. Li and et al,
., Advances in MXene Films: Synthesis, Assembly, and Applications, Trans. Tianjin Univ., 2021, 27(3), 217–247 CrossRef CAS.
- A. Sunny, S. Rajalekshmi and A. Pandikumar, Nanocomposite engineering: Tailoring MXene/Cobalt oxide for efficient electrocatalytic hydrogen and oxygen evolution reactions, J. Alloys Compd., 2024, 1003, 175532 CrossRef CAS.
- N. U. Kiran and et al,
., Comparative Study of Cold Electron Emission from 2D Ti3C2Tx MXene Nanosheets with Respect to Its Precursor Ti3SiC2 MAX Phase, ACS Appl. Electron. Mater., 2022, 4(6), 2656–2666 CrossRef CAS.
- J. Wang and et al,
., Fabrication of dispersive α-Co(OH)2 nanosheets on graphene nanoribbons for boosting their oxygen evolution performance, J. Mater. Sci., 2019, 54(10), 7692–7701 CrossRef CAS.
- M. R. da Silva Pelissari and et al,
., Ascorbic acid electrocatalytic activity in different electrolyte solutions using electrodeposited Co(OH)2, Ionics, 2019, 25(4), 1911–1920 CrossRef CAS.
- D. Liu and et al,
., Magnetic Ti3C2 MXene Nanomaterials for Doxorubicin Adsorption from Aqueous Solutions: Kinetic, Isotherms, and Thermodynamic Studies, ACS Omega, 2022, 7(36), 31945–31953 CrossRef CAS PubMed.
- L. Pu and et al,
., N-doped MXene derived from chitosan for the highly effective electrochemical properties as supercapacitor, Adv. Compos. Hybrid Mater., 2022, 5(1), 356–369 CrossRef CAS.
- S. I. Perez Bakovic and et al,
., Electrochemically active surface area controls HER activity for FexNi100−x films in alkaline electrolyte, J. Catal., 2021, 394, 104–112 CrossRef CAS.
- S. Maitra and et al,
., Electrochemical aspects of sol-gel synthesized MgCoO2 for aqueous supercapacitor and alkaline HER electrocatalyst applications, Curr. Appl. Phys., 2020, 20(12), 1404–1415 CrossRef.
- L. Huang and et al,
., Hierarchical MoS2 nanosheets integrated Ti3C2 MXenes for electrocatalytic hydrogen evolution, Int. J. Hydrogen Energy, 2019, 44(2), 965–976 CrossRef CAS.
- C. Zhang and et al,
., High-throughput production of cheap mineral-based two-dimensional electrocatalysts for high-current-density hydrogen evolution, Nat. Commun., 2020, 11(1), 3724 CrossRef CAS PubMed.
- J. Mei, Surface-Dependent Intermediate Adsorption Modulation on Iridium-Modified Black Phosphorus Electrocatalysts for Efficient pH-Universal Water Splitting, Adv. Mater., 2021, 33(49), 2104638 CrossRef CAS PubMed.
- B. Sarfraz, HF free greener Cl-terminated MXene as novel electrocatalyst for overall water splitting in alkaline media, Int. J. Energy Res., 2022, 46(8), 10942–10954 CrossRef CAS.
- J. Zhang, Carbon-Rich Nanomaterials: Fascinating Hydrogen and Oxygen Electrocatalysts, Adv. Mater., 2018, 30(40), 1800528 CrossRef PubMed.
- I. Khan and et al,
., Robust electrocatalysts decorated three-dimensional laser-induced graphene for selective alkaline OER and HER, Carbon, 2023, 213, 118292 CrossRef CAS.
- S.-Y. Pang and et al,
., Universal Strategy for HF-Free Facile and Rapid Synthesis of Two-dimensional MXenes as Multifunctional Energy Materials, J. Am. Chem. Soc., 2019, 141(24), 9610–9616 CrossRef CAS PubMed.
- Y. Tan and et al,
., Nb4C3Tx (MXene) as a new stable catalyst for the hydrogen evolution reaction, Int. J. Hydrogen Energy, 2021, 46(2), 1955–1966 CrossRef CAS.
- S. Tang and et al,
., Recent advances in transition metal nitrides for hydrogen electrocatalysis in alkaline media: from catalyst design to application, Front. Chem., 2022, 10, 1073175 CrossRef CAS PubMed.
- S. Pakhira, V. Kumar and S. Ghosh, Revealing the Superior Electrocatalytic Performance of 2D Monolayer WSe2 Transition Metal Dichalcogenide for Efficient H2 Evolution Reaction, Adv. Mater. Interfaces, 2023, 10(8), 2202075 CrossRef CAS.
- A. Kazemi, F. Manteghi and Z. Tehrani, Metal Electrocatalysts for Hydrogen Production in Water Splitting, ACS Omega, 2024, 9(7), 7310–7335 CAS.
- R. A. Mir, S. Upadhyay and O. P. Pandey, A review on recent advances and progress in Mo2C@C: a suitable and stable electrocatalyst for HER, Int. J. Hydrogen Energy, 2023, 48(35), 13044–13067 CrossRef CAS.
- S. Venkateshalu and et al,
., Synergistic MXene/LDH heterostructures with extensive
interfacing as emerging energy conversion and storage materials, J. Mater. Chem. A, 2023, 11(27), 14469–14488 RSC.
|
This journal is © The Royal Society of Chemistry 2024 |
Click here to see how this site uses Cookies. View our privacy policy here.