Structural coloration of textiles with PMMA photonic crystals†
Received
22nd July 2023
, Accepted 10th November 2023
First published on 11th November 2023
Abstract
To achieve the whole ecological preparation and application of structurally colored textiles with photonic crystals (PCs), degradable PMMA colloidal nanospheres in a high mass fraction (30 wt%) were synthetically prepared by an emulsion polymerization method, and then PMMA liquid photonic crystals (LPCs) with a pre-crystallized form were efficiently prepared by a rotary evaporation/surfactant method. The LPCs exhibited excellent dynamic reversibility and high color saturation. By utilizing the LPCs as assembly intermediates and combining with a shear-induced assembly technique, the large-scale and rapid structural coloration of textiles was realized. Thanks to a PU polymer layer pretreated on the fabric surface, the structural stability of the PMMA photonic crystals on textile substrates was significantly improved, ensuring consistency between the structural stability and color saturation of the PMMA photonic crystals. The dialysis of the colloidal PMMA nanospheres removed the oligomers and small molecule substances, further improving the structural stability of PMMA photonic crystals.
1. Introduction
Photonic crystals (PCs) are a kind of crystalline material formed by the periodic arrangement of two or more media with different refractive indices.1–4 Using colloidal nanospheres as the assembly units to construct biomimetic PC structures has become an important approach for biomimetic coloration of textiles, which is due to the controllability of the assembly process and excellent optical properties of the assembled structures.5–8 Colloidal nanospheres of inorganic silica (SiO2) and organic polystyrene (PS) are frequently used as building blocks for fabricating PCs.9 These two types of colloidal nanospheres are favorable building blocks due to their abundant raw material sources, uniform size, good monodispersity, high storage stability, and ability to construct PCs with beautiful structural colors and iridescent effects.10,11 However, so far, it is still a great challenge to prepare colloidal SiO2 nanospheres with high mass fraction and in large quantities efficiently.12–19 In addition, the Stöber method is the most commonly used method for the preparation of SiO2 nanospheres, but it needs to use large amounts of organic solvent.20,21 In turn, the preparation of PS colloidal nanospheres is simple, enabling one to realize their widespread fabrication in a controllable manner. The recent reports on PS liquid photonic crystals (LPCs) have confirmed the possibility of the rapid and large-scale production of structurally colored fabrics with PS photonic crystals.22,23 However, PS nanospheres contain benzene rings, which strongly absorb ultraviolet (UV) light, leading to a decreased weather resistance of the assembled PCs. Also, the presence of benzene rings makes PS nanospheres difficult to undergo natural degradation,24,25 which is not conducive to sustainable development of textiles. In view of the above background, the PMMA nanospheres are expected to replace SiO2 and PS as the assembly blocks of PCs suitable for textile coloration at an industrial scale.
Polymethyl methacrylate (PMMA) colloidal nanospheres are synthesized by using the methyl methacrylate (MMA) monomer, which has rich sources and possesses good degradability.26 In addition, the PCs assembled from PMMA colloidal nanospheres possess good biocompatibility and environmental stability, and can be degraded in soil after being discarded, which is beneficial to the full-range of ecological production and application of structurally colored textiles with PMMA PCs.27,28 However, there are still some challenges in the practical preparation and applications of PMMA colloidal nanospheres. The first issue is that the hydrophilicity of MMA is stronger than that of St, and thus the monodispersity and sphericity of PMMA nanospheres are more difficult to control than those of PS nanospheres when synthesizing a high mass fraction of PMMA colloidal nanospheres on a large scale.29,30 The second issue is that, when attempting to increase the mass fraction of the colloidal PMMA nanospheres to prepare PMMA-based LPCs, the nanospheres are prone to agglomerate due to their collision and coalescence. The third issue is that the structural stability of PMMA photonic crystals constructed by using common methods is not good enough for practical applications, since PMMA nanospheres have a rigid structure and the binding between them primarily relies on weak interactions such as van der Waals forces and hydrogen bonding, leading to disruption of the PC structure easily under external forces and thus color attenuation or even complete disappearance of the structural color.
This work studies the direct synthesis of high mass fraction (30 wt%) PMMA colloidal nanospheres with a particle size range of 190–380 nm, as well as good monodispersity (PDI < 0.08) and sphericity. In addition, the pre-crystallized PMMA-based LPCs were prepared via a physical rotary evaporation/surfactant method. The high mass fraction of PMMA-based LPCs was then applied onto the PU-pretreated textile substrate through a shear-induced assembly technique, enabling large-scale and rapid production of structurally colored fabrics with PMMA photonic crystals. Thanks to the migration and secondary curing of the interfacial PU molecules/chain segments into the PC structure, the structural stability of the PCs on textile substrates was significantly improved, ensuring the consistency between structural stability and color saturation of the PMMA photonic crystals, and realizing the whole ecological preparation and application of structurally colored textiles.
2. Experimental
2.1. Materials
Methyl methacrylate (MMA), sodium dodecyl sulfate (SDS), and potassium persulfate (KPS) (analytical grade, Aladdin Reagent Co., Ltd); nano-carbon black (Emperor 1800, Cabot Co., Ltd); polyurethane (PU) polymer (Shanghai Sisheng Polymer Materials Co., Ltd); nonionic surfactant JFC and crack-prevention agent (industrial grade, Zhejiang TransFar Chemicals Co., Ltd); nitrogen gas (N2) (purity 99.99%, Hangzhou Jingong Special Gas Co., Ltd); deionized water (conductivity of 18 MΩ cm−1, self-made); polyester fabric (80 g m−2, Haining Green-Gard Textile Sci-Tech Co., Ltd).
2.2. Preparation of the monodisperse PMMA nanosphere dispersion
A 30 wt% monodisperse PMMA nanosphere dispersion was prepared via an emulsion polymerization method. First, 250 mL of deionized water was added to a 500 mL four-neck round-bottom flask equipped with a mechanical agitator, nitrogen inlet tube, and condenser reflux apparatus. Then, 0.1 g of emulsifier (SDS) and 90 g of MMA were added and stirred at a speed of 350 rpm for 30 min. Once the water bath temperature reached 75 °C, 0.2 g of initiator (KPS) dissolved in 50 mL of deionized water was added to initiate the polymerization in the reaction system. After 2 h of the reaction, a monodisperse PMMA nanosphere dispersion with excellent sphericity and desirable particle size was obtained. By changing the amount of emulsifier or initiator, colloidal PMMA nanospheres with various particle sizes can be obtained.
2.3. Preparation of PMMA liquid photonic crystals
First, 200 mL of the prepared 30 wt% monodisperse PMMA nanosphere dispersion and an appropriate amount of dispersing agent (nonionic surfactant JFC) were poured into a rotary evaporation system. The rotary evaporation system was fixed and adjusted to an appropriate height. The rotation speed was set at 52 rpm, a temperature of 45 °C, and vacuum degree of 0.0974 MPa. After a certain time of rotary evaporation, the pre-crystallized LPCs with structural colors were obtained. By changing the rotary evaporation time, LPCs with various mass fractions were prepared. The evaporated LPCs were afterward placed in a dialysis bag with a molecular weight of 10
000 to perform dialysis, and the purified PMMA-based LPCs were finally extracted.
2.4. Preparation of large-area structurally colored fabrics with PMMA photonic crystals
To prepare the large-area structurally colored fabrics with PCs, a PU polymer layer was pretreated on the fabric surface and cured at 100 °C for 1 minute. The crack-prevention agent (5 wt%) and nano-carbon black (0.24 wt%) were introduced into the LPCs and ultrasonically mixed until a uniform LPC paste was obtained. The LPC paste was applied onto the PU pretreated fabric surface by using a wire rod, and then heated at 65 °C for 5 min to obtain the structurally colored fabric with solid PMMA photonic crystals.
2.5. Stabilization of PMMA photonic crystals
The above structurally colored fabric with PMMA photonic crystals was further heated for a certain time at specified temperatures (60 °C, 70 °C, 80 °C, 90 °C, and 95 °C) to obtain the structurally colored fabrics with different degrees of stabilization. The stabilization effect was evaluated according to the changes in color fastness and color saturation, and then the stabilization conditions were optimized as well.
2.6. Testing and characterization
The particle size and monodispersity of the nanospheres were characterized using a laser particle size analyzer (Nano-S, Malvern Instruments, UK). The surface zeta potential of the nanospheres was assessed by means of a zeta potential analyzer (Zeta PALS, Brookhaven Instruments Corp., USA). The arranged structures of the nanospheres and structurally colored photon crystals were examined via field emission scanning electron microscopy (FESEM, ULTRA55, Carl Zeiss, Germany). The colors of the liquid photon crystals and structurally colored films were monitored with a 3D video microscope (KH-7700, Koshida Corp., USA) and a digital camera (EOS600D, Canon, Japan). The reflective spectra of the liquid photon crystals and structural colored fabrics were recorded using a fiber optic spectrometer (Maya 2000, Ocean Optics Inc., USA).
3. Results and discussion
3.1. Preparation of the PMMA nanosphere dispersion
A series of 30 wt% PMMA colloidal nanospheres with various particle sizes were prepared via controllable emulsion polymerization, and their characterization results are shown in Fig. 1 and Fig. S1 (ESI†). According to the SEM images and the corresponding histograms of microsphere size distributions of PMMA nanospheres shown in Fig. 1a, the prepared PMMA nanospheres had uniform size and good sphericity. However, it should be noted that the dynamic light scattering (DLS) diameter of the colloidal PMMA nanospheres (Fig. 1b) was found to be larger than the actual diameter observed in the SEM images. The main reason for this difference was attributed to the presence of oxygen atoms on the PMMA nanosphere surface, which formed hydrogen bonds with water molecules, resulting in the emergence of a water molecule layer on the PMMA nanosphere surface and causing the increase in DLS-furnished diameters relative to those evaluated from the SEM images.31
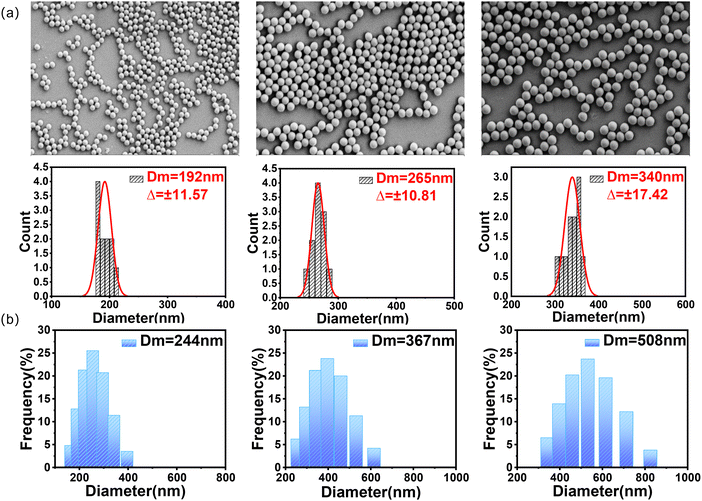 |
| Fig. 1 (a) SEM images and the corresponding histograms of the microsphere size distributions of PMMA nanospheres. (b) Hydrated particle size distributions of PMMA colloidal nanospheres. | |
3.2. Preparation of PMMA liquid photonic crystals
Adding a nonionic surfactant to the rotary evaporation system can prevent the aggregation and sedimentation of nanospheres, thereby resulting in a well-dispersed high-concentration nanosphere system. Fig. 2a illustrates the synergistic effects of the nonionic surfactant and the anionic surfactant in the prevention of microsphere aggregation. During the rotary evaporation process, the probability of microsphere collisions rose with the increasing mass fraction of nanospheres. The electrostatic repulsion induced by the introduction of the anionic surfactant during the synthesis was insufficient to counteract the collision between nanospheres. As a result, the nanospheres underwent aggregation and sedimentation. Once the nonionic surfactant was placed into the rotary evaporation system, the spatial resistance of the three-dimensional interface blocking layer between nanospheres increased through the synergistic effect of anionic/nonionic surfactants, preventing microsphere collisions and ensuring good dispersion stability.32Fig. 2b displays the microscopic images and reflection intensity plots of the rotary evaporation process with different amounts of nonionic surfactant. With an increase in the amount of the nonionic surfactant, the color of the nanosphere system became more vivid, indicating a significant improvement in crystallinity. However, when the surfactant dosage was too high, it could also interfere with the nanosphere pre-crystallization, thereby deteriorating the optical properties of the LPCs. Fig. 2c displays the optical images of LPCs with different particle sizes. Because of a high concentration of solvent in the LPCs, the structural color of the latter ones shifted toward longer wavelengths with the increase in lattice spacing. Fig. 2f shows the corresponding reflectance curves of LPCs with different particle sizes. The reflection peak of LPCs was high and narrow, proving that LPCs possessed high color saturation (brightness), which was consistent with Fig. 2c.
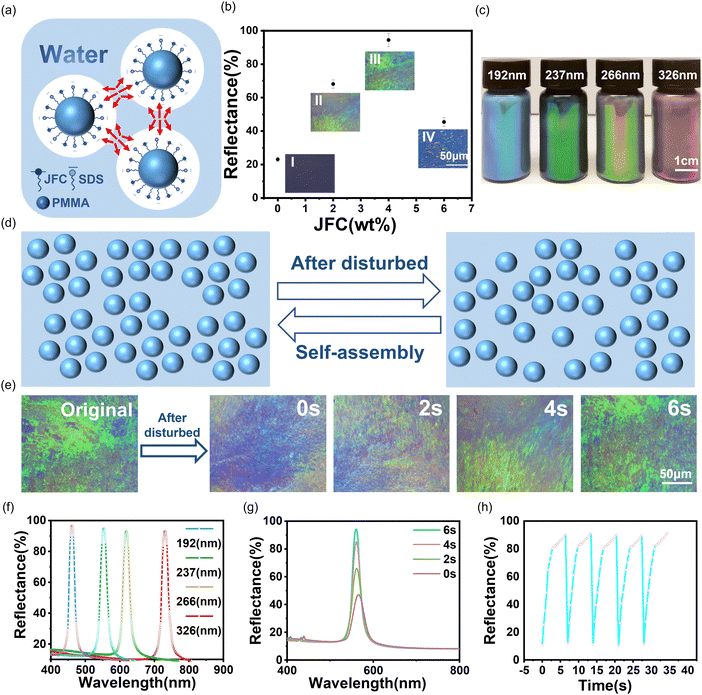 |
| Fig. 2 (a) Schematic diagram of anionic/nonionic synergistic interactions; (b) optical images of microsphere systems with 0, 2, 4 and 6 wt% dispersant nonionic surfactant JFC after rotary evaporation and corresponding reflection intensities; (c) optical images of LPCs with different particle sizes; (d) schematic diagram illustrating excellent dynamic reversibility of LPCs; (e) self-assembly process of LPCs after being disturbed; (f) reflection spectra of LPCs with different particle sizes; (g) corresponding changes in reflectance; (h) changes in reflection intensity during the reversible disassembly process. | |
Fig. 2d depicts the schematic diagram of the transition between the pre-crystallized form of the LPCs and the destruction of the crystal state after the external disturbance. When a certain external force was applied to the PMMA LPCs, the balance between the electrostatic repulsion and van der Waals forces between PMMA nanospheres was disrupted, leading to the disassembly of the ordered arrangement of nanospheres. Once the external force was removed, the nanospheres spontaneously reassembled into an ordered arrangement. Fig. 2e displays a self-assembly process of the LPCs after the external disturbance, and their structural color recovered the maximum order degree within 6 s. Fig. 2g represents the change in reflection peak intensity of PMMA LPCs before and after the external loading, revealing that the intensity of the reflection spectrum gradually increased after the release of the external load. Fig. 2h shows the reflection peak intensities of the disassembled and reassembled LPCs, which persist even after five consecutive disassembly and reassembly cycles. These results confirmed that the prepared LPCs exhibited excellent dynamic reversibility.
3.3. Preparation of large-area structurally colored fabrics with PMMA photonic crystals
Thanks to the excellent fluidity and dynamic reversibility of LPCs combined with external shear-induced assembly techniques, the LPCs on textile substrates can be transformed from a paste-like bulk phase accumulation state to a uniformly distributed flat film-like structure. Under the shear-induced effect, LPCs are first disassembled into disordered structures under external forces during the spreading process. Then, through the balance between electrostatic repulsion and van der Waals forces, nanospheres rapidly reassemble and reconstruct into a film-like structure. With the evaporation of the solvent (water), the resulting film transforms into a solid PC film with a vivid structural color. Fig. 3a displays the large-area structurally colored fabrics with photonic crystals. By changing the size of the PMMA nanospheres in the LPCs, the hue of structurally colored fabrics with photonic crystals could be controlled. As soon as the nanosphere size decreased from 326 nm to 192 nm, the lattice spacing of the PC structure decreased as well, causing the structural color to blue-shift. Fig. 3b presents the reflectance spectra of PC structurally colored fabrics with different particle sizes. Once the microsphere size decreased, the reflectance peak shifted toward shorter wavelengths. The reflection peaks were intense and narrow, indicating high color saturation of the PC structurally colored textiles. It is noteworthy that the production of structural colors in PC covered textiles is due to selective reflection of light, resulting in coherent diffraction and interference on the PC surface. Therefore, the measured reflectance may reach 100%. Fig. 3c and Fig. S2 (ESI†) illustrate the iridescent effect of structurally colored fabrics with PMMA photonic crystals. In natural environments, the PC structurally colored fabrics exhibited stunning structural colors and dazzling iridescent effects. The observed structural color tended to blue-shift when the viewing angle increased as the Bragg formula described (Fig. S2, ESI†).
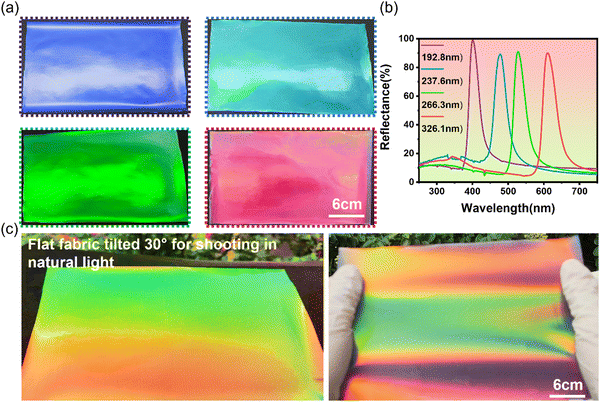 |
| Fig. 3 (a) Digital photos of large-area PC structurally colored fabrics with different nanosphere sizes; (b) reflectance spectra of PC structurally colored fabrics with different particle sizes; (c) iridescent effect of large-area structurally colored fabrics with PMMA photonic crystals. | |
3.4. Stabilization of structurally colored fabrics with PMMA photonic crystals
Since PMMA colloidal nanospheres have a rigid structure, the PCs were assembled through only weak interactions such as van der Waals forces and hydrogen bonding between nanospheres themselves and between nanospheres and fabrics. Under the action of external forces such as bending, washing and rubbing, the PMMA photonic crystals are easily destroyed, resulting in a decrease in color saturability and brightness (even completely achromatic) of the structural colors. In order to improve the stability of the PC structure and the durability of the structural color, a PU polymer layer was pretreated on the surface of the textile substrate. During the self-assembly process of the colloidal PMMA nanospheres, the interfacial PU molecules/chain segments of the underlying PU layer were relaxed under the joint action of heat, water and surfactant existing in LPCs. Being exposed to the induced effects of water evaporation and capillary force, the interfacial PU molecules or/and the chain segments moved upward to the interior of the PC layer and finally solidified again, which acted as a “ligament” between the structural units of the PMMA photonic crystals, and significantly improved the stability of the assembled PMMA photonic crystals to external forces (bending, rubbing, washing, etc.), and thus realized the consistency of the high PC stability with high color saturation.23,33
Fig. 4 depicts the bending fastness diagrams and corresponding reflectance curves of PC structurally colored fabrics stabilized by curing at 60 °C, 70 °C, 80 °C, 90 °C and 95 °C for various curing times after the self-assembly process. It can be seen that the bending fastness of the structurally colored fabrics was improved with the increase of the curing temperature or/and the extension of the curing time, which is because of the rising migration amount of the interfacial PU molecules/chain segments. The optimized bending fastness of the structurally colored fabric was achieved through curing at 90 °C for 30–60 min. According to the reflectance curves at various temperatures, the saturation and brightness of the PMMA photonic crystals remained unchanged when cured at 60 °C to 90 °C for a period of time. After curing at 95 °C for more than 30 min, the interfacial PU molecules/chain segments excessively migrated to the PCs, and thus the filling medium between the nanospheres changed from air to PU. As a result, the refractive index contrast between the PCs and the filling medium decreased, causing the structural color of the PCs to deteriorate. In order to prepare PMMA photonic crystals with both high structural stability and high color saturation, the curing temperature was controlled at 90 °C. The curing temperature must be below 100 °C because the glass transition temperature of PMMA nanospheres was approximately 100–105 °C so as to ensure their chain stability34 (Fig. S3, ESI†).
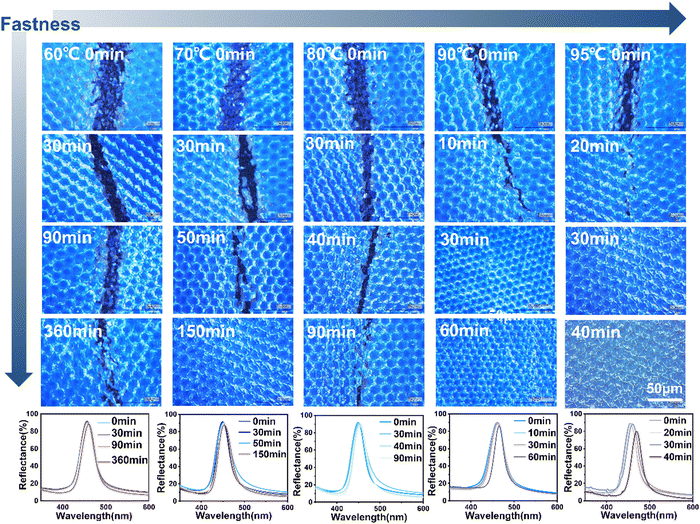 |
| Fig. 4 Optical images and reflectance spectra of the structurally colored fabrics with PMMA photonic crystals stabilized by curing at various temperatures and times. | |
Because of the presence of a small number of oligomers and salts in the PMMA-based LPC system, the effect of oligomers on the color and fastness of PC structurally colored fabric was further investigated. Fig. 5a is a schematic diagram of the co-assembly of oligomers with PMMA nanospheres. A few oligomers were distributed randomly among the PMMA nanospheres. As discussed before, during the assembly process and the stabilization process, the interfacial PU molecules/chain segments upward migrated and secondary cured in the PC layer, playing the role of a “ligament” between the nanospheres, but the stiff PMMA oligomers embedded in the flexible PU play the role of “stones”, deteriorating the toughness of the PU polymer. By performing dialysis with a 10
000 molecular weight cutoff bag, the oligomers and salt substances were removed from the LPCs, which increased the dielectric constant and pre-crystallization degree of the LPCs, as well as enhanced the structural stability of PMMA photonic crystals on textile substrates. Fig. 5b depicts the SEM images of PMMA nanospheres before and after dialysis. Obviously, the nanospheres after dialysis are much cleaner than those before dialysis. Fig. 5c shows the mass fraction of LPCs, which decreased after dialysis, further demonstrating that the dialysis eliminated oligomers from LPCs. Fig. 5d depicts the zeta potential of the LPCs, which significantly increased after dialysis, because the ions such as Na+ and K+ were removed, reducing the ionic strength of the system, increasing the dielectric constant of the medium, and thus enhancing the electrostatic repulsion potential between the nanospheres (Fig. S4, ESI†).23 As shown in Fig. 5e, the bending fastness was greatly improved, which confirmed that the presence of oligomers would have reduced the toughness of the PU migrated and cured in the PC layer.
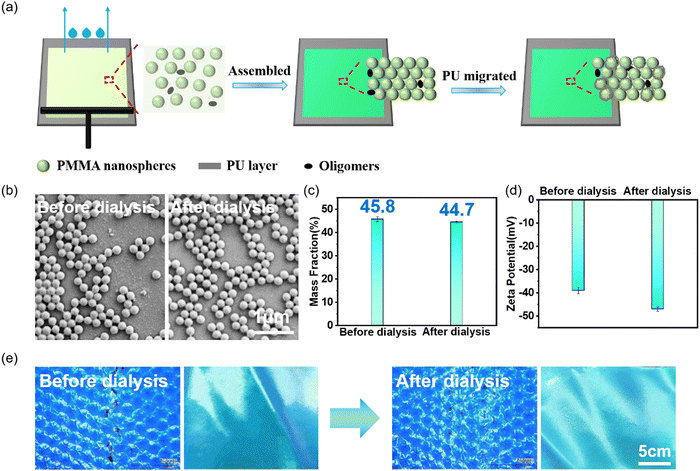 |
| Fig. 5 (a) Schematic diagram of the co-assembly of oligomers with PMMA nanospheres; (b) SEM images of PMMA nanospheres before and after dialysis; (c) solid contents of PMMA microsphere dispersion before and after dialysis; (d) zeta potentials of the colloidal nanosphere system before and after dialysis; (e) comparative images of structural color and bending resistance of structurally colored fabrics. | |
4. Conclusions
In this work, the controllable synthesis of high mass fraction (30 wt%) of colloidal PMMA nanospheres by emulsion polymerization was studied, and the prepared colloidal PMMA nanospheres possessed good monodispersity (PDI < 0.08) and excellent sphericity even if synthesizing a high mass fraction (30 wt%) of PMMA colloidal nanospheres in a large scale of 1.5 L. Then, PMMA liquid photonic crystals (LPCs) with a pre-crystallized form were efficiently prepared by a rotary evaporation/surfactant method. The prepared LPCs exhibited excellent dynamic reversibility and high color saturation. By utilizing the LPCs as assembly intermediates and combining with a shear-induced assembly technique, the large-scale and rapid structural coloration of textiles was realized. Thanks to a polyurethane polymer layer pretreated on the fabric surface, the structural stability of the PMMA photonic crystals was significantly enhanced, ensuring consistency between high structural stability and high color saturation of PMMA photonic crystals constructed on textile substrates. The high bending fastness of the structurally colored fabric was achieved through an optimized curing at 90 °C for 30–60 min. The dialysis of the colloidal PMMA nanospheres removed the oligomers and small molecule substances, further improving the bending fastness of the structurally colored fabrics.
Data availability statement
The data that support the findings of this study are available from the corresponding author upon reasonable request.
Author contributions
Yanan Wang: conceptualization, formal analysis, investigation, validation, methodology, and writing; Xinyang Li: investigation, formal analysis; Yalan Zhang: investigation; Xiaohui Wang: formal analysis; Yichen Li: investigation; Yijia Liu: investigation; Dong Wang: investigation; Guojin Liu: investigation; Yi Huang: formal analysis; Jianzhong Shao: conceptualization, supervision, funding acquisition, review and editing.
Conflicts of interest
The authors have declared no conflicts of interest.
Acknowledgements
This work was supported by the National Natural Science Foundation of China (No. 51773181, 52003242, and 52203275), China Postdoctoral Science Foundation (2022M712302), Zhejiang Provincial Natural Science Foundation of China (LY20E030006), and the Excellent Postgraduate Thesis Program of Zhejiang Sci-Tech University (LW-YP2021003).
References
- E. Yablonovitch, Phys. Rev. Lett., 1987, 58, 2059–2062 CrossRef CAS PubMed.
- S. John, Phys. Rev. Lett., 1987, 58, 2486–2490 CrossRef CAS.
- G. V. Freymann, V. Kitaev, B. V. Lotsch and G. A. Ozin, Chem. Soc. Rev., 2013, 42, 2528–2554 RSC.
- Z. Y. Cai, Z. W. Li, S. Ravaine, M. He, Y. L. Song, Y. D. Yin, H. B. Zheng, J. H. Teng and A. Zhang, Chem. Soc. Rev., 2021, 50, 5898–5951 RSC.
- Y. L. Wang, H. Q. Cui, Q. L. Zhao and X. M. Du, Matter, 2019, 1, 626–638 CrossRef.
- Y. J. Zhao, Z. Y. Xie, H. C. Gu, C. Zhu and Z. Z. Gu, Chem. Soc. Rev., 2012, 41, 3297–3317 RSC.
- S. Sacanna and D. J. Pine, Curr. Opin. Colloid Interface Sci., 2011, 16, 105 CrossRef.
- M. Wang and Y. Yin, J. Am. Chem. Soc., 2016, 138, 6315–6323 CrossRef CAS PubMed.
- J. Teyssier, S. V. Saenko, D. V. D. Marel and M. C. Milinkovitch, Nat. Commun., 2015, 6, 6368 CrossRef CAS PubMed.
- F. F. Liu, S. F. Zhang, Y. Meng and B. T. Tang, Small, 2020, 16, 2002319 CrossRef CAS.
- D. P. Josephson, M. Miller and A. Stein, Z. Anorg. Allg. Chem., 2014, 640, 655–662 CrossRef CAS.
- Y. L. Zhao, R. Li, B. S. Wang, Y. Huang, P. Lyu, F. Wang, Q. Y. Jiang, Y. Han, S. L. Zhang, X. K. Wu, S. M. Zhao, N. Zhu and R. F. Zhang, ACS Nano, 2023, 17(3), 2893–2900 CrossRef CAS.
- W. Wang, X. A. Fu, J. A. Tian and L. Jiang, Colloids Surf., A, 1993, 81, 177–180 CrossRef CAS.
- M. H. J. H. Al-Atia, H. K. Saeed, A. R. Fliayh and A. J. Addie, Colloids Surf., A, 2017, 520, 590–596 CrossRef CAS.
- G. J. Liu, L. Zhou, G. Q. Zhang, Y. C. Li, L. Q. Chai, Q. G. Fan and J. Z. Shao, Mater. Des., 2017, 114, 10–17 CrossRef CAS.
- B. T. Zhu, Q. Q. Fu, K. Chen and J. Q. Ge, Angew. Chem., Int. Ed., 2018, 57, 252–256 CrossRef CAS PubMed.
- C. Wang, X. Lin, C. G. Schafer, S. Hirsemann and J. P. Ge, Adv. Funct. Mater., 2021, 31(9), 2008601 CrossRef CAS.
- H. Kaur, S. Chaudhary, H. Kaur, M. Chaudhary and K. C. Jena, ACS Appl. Nano Mater., 2022, 5(1), 411–422 CrossRef CAS.
- H. Y. He, L. Y. Liu, Q. Q. Fu and J. P. Ge, Adv. Funct. Mater., 2022, 32, 2200330 CrossRef.
- M. Meier and J. U. M. K. H. Nirschl, Colloids Surf., A, 2018, 538, 559–564 CrossRef CAS.
- A. D. S. D. Silva and J. H. Z. D. Santos, Adv. Colloid Interface Sci., 2023, 102888 CrossRef.
- Y. C. Li, Q. S. Fan, X. H. Wang, G. J. Liu, L. Q. Chai, L. Zhou, J. Z. Shao and Y. D. Yin, Adv. Funct. Mater., 2021, 31, 2010746 CrossRef CAS.
- X. Y. Li, X. H. Liang, X. H. Wang, Y. C. Li, G. J. Liu, M. G. Hu, L. Zhou and J. Z. Shao, Dyes Pigm., 2023, 209, 110913 CrossRef CAS.
- A. A. A. Alim, S. S. M. Shirajuddin and F. H. Anuar, J. Chem., 2022, 2022, 7670819 Search PubMed.
- C. Caicedo, L. M. López, C. J. C. Alvarado, V. C. Delgado and C. A. Á. Orta, DYNA, 2019, 86, 288–299 CrossRef.
- C. B. Xie, K. W. Leng, J. Sheng, X. M. Wang, Q. M. Li, L. Song, L. Liu, H. J. Sun, X. W. Huang, Z. W. Wang and Q. Yu, Colloid Polym. Sci., 2020, 298, 1285–1291 CrossRef CAS.
- Y. F. Gao, J. M. Zhang, J. Liang, D. M. Yuan and W. Z. Zhao, Eur. Polym. J., 2022, 175, 111379 CrossRef CAS.
- R. Elashnikov, O. Lyutakov, P. Ulbrich and V. Svorcik, Biomater. Adv., 2016, 64, 229–235 CAS.
- I. V. Nemtsev, O. V. Shabanova, N. P. Shestakov, A. V. Cherepakhin and V. Y. Zyryanov, Appl. Phys. A: Mater. Sci. Process., 2019, 125, 738 CrossRef.
- W. P. Kang, Y. Bao, H. L. Wang, N. P. Cao and S. X. Cui, Chin. J. Chem., 2023, 41, 2289–2295 CrossRef CAS.
- W. Barbour, S. Saika, T. Miyamoto and Y. Ohnishi, Ophthalmic Res., 2005, 37, 255–261 CrossRef CAS.
- T. Joshi and J. M. P. Bahadur, Colloids Surf., A, 2005, 260, 209–215 CrossRef CAS.
-
J. Z. Shao, X. H. Wang, Z. P. Tang, M. G. Hu, Y. Gao and Y. J. Liu, China Pat., CN202111437620.8, 2021.
- J. Wie and J. Kim, Polymers, 2019, 11, 1347 CrossRef.
|
This journal is © The Royal Society of Chemistry 2024 |
Click here to see how this site uses Cookies. View our privacy policy here.