DOI:
10.1039/D4NA00539B
(Paper)
Nanoscale Adv., 2025,
7, 310-319
Enhanced field emission performance of gold nanoparticle decorated Bi2S3 nanoflowers†
Received
2nd July 2024
, Accepted 12th November 2024
First published on 13th November 2024
Abstract
Au nanoparticles (NPs) are decorated on hydrothermally synthesized Bi2S3 nanorods (NRs) to enhance the field electron emission (FEE) performance as compared to bare Bi2S3 nanorods, resulting in reduction in turn-on field from 3.7 to 2.7 V μm−1 (at the current density of 1.0 μA cm−2) with significant increment in maximum emission current density from 138 to 604.8 μA cm−2 (at a field of 7.8 V μm−1) respectively. FESEM/TEM reveals that Bi2S3 nanoflowers are assembled from Bi2S3 NRs of a typical diameter of 120 ± 10 nm, and Au NPs of diameter about 5–10 nm are uniformly decorated onto the surface of NRs to form an Au/Bi2S3 composite. XRD analysis suggests that the as-synthesized product consists of orthorhombic Bi2S3 NRs decorated with face-centered cubic Au NPs. The XPS spectrum shows the elemental mapping of the as-synthesized Au/Bi2S3. Improvement in field emission properties is mainly attributed to a reduction in work function and increasing emitting sites due to Au NP decoration.
1 Introduction
Field emission is a quantum mechanical tunneling effect in which electrons are emitted from a metal/semiconductor surface into a vacuum under a sufficiently intense electric field. FEE cathodes possess diverse advantages over thermionic emitters with respect to durability, current density, and low energy consumption, which place FEE devices in the competition for next-generation electronics. FEE cathodes are widely used in certain electron-beam devices, such as flat panel displays,1 scanning electron microscopes,2 and X-ray sources.3 The FEE properties of emitters are related to their composition, tip sharpness, conductivity, field enhancement factor, and work function. To increase the field enhancement factor β it is required to reduce tip sizes and modify the work function, by doping, decorating or preparing composites of nanostructured materials. In this sense, much effort has been made to study nanostructure cathodes for FEE applications such as LaB6 nanowires,4 carbon nanotubes (CNTs),1,5 ZnO,6 TiS nanosheets,7 as well as composites such as CNTs-LaB6,8 rGO-ZnS,9 rGO-ZnO,10 Au-ZnO,11etc. have been reported as possible field emitter materials.
Since the last few years, metal sulfides have been extensively studied due to their tunable optical and electronic properties, so they are extensively used in energy conversion and storage device applications such as solar cells,12 supercapacitors,13 Na-ion batteries,14etc. Among various metal sulfides, Bi2S3 is the most extensively researched direct bandgap semiconductor. As shown in Fig. 1b, the Bi2S3 crystal structure is formed by the polymerization of the tightly bonded [Bi4S6] unit, where each unit is connected by weak Bi–S and S–S interaction.15 The benefits of low cost, abundance, non-toxicity, and excellent optoelectronic and electrical properties make Bi2S3 an excellent material for practical applications, such as in photodetectors,16 solar cells,17 photocatalysis,18,19 supercapacitors,20etc. Improvement in FEE performance was mainly attempted by increasing the field enhancement factor (depending on the shape/morphology of the emitters) and reducing the work function of nanostructured emitters. In order to improve FEE performance, it is preferred to study composite/heterostructure properties of nanostructured metal sulfides. Recently, number of investigations were made to improve the FEE performance by blending rGO,21,22 metal oxides,23,24 sulfides,25,26 and materials with various metal sulfides of 1D/2D nanostructures. In addition, many efforts have been made to study the morphology-dependent FEE performance of Bi2S3 nanostructures.27–30 However, FEE improvement by work function modulation is less explored for the Bi2S3 nanostructure. Our group has investigated the FEE performance of CdS-Bi2S3 (ref. 25) and rGO-Bi2S3 (ref. 31) composites.
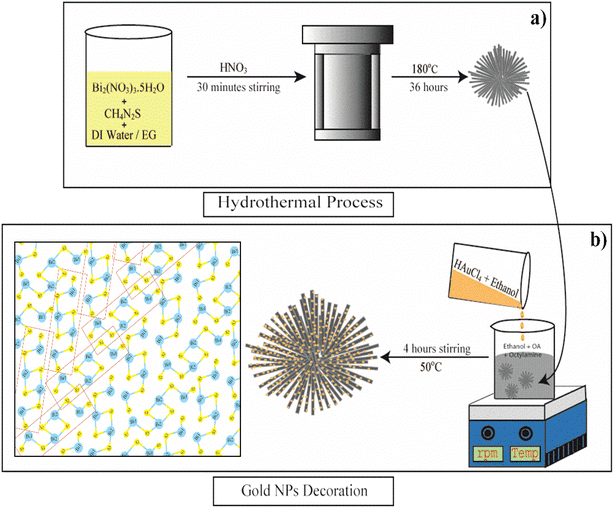 |
| Fig. 1 Schematic depiction of the mechanism of (a) the hydrothermal synthesis of Bi2S3 microflowers, (b) Au decoration process on as-synthesized Bi2S3 NRs and schematic structure of Bi2S3. | |
Noble metal NPs (Au, Ag, and Pt) on semiconductors have been extensively studied and exploited in several applications, such as photocatalysis,32,33 solar cells,34,35 light-emitting diodes,36 photodetectors,37,38 FETs,39etc. These reports suggest that the decoration of noble metals on nanomaterials enhances the performance of bare nanomaterials due to the Surface Plasmon Resonance (SPR) phenomenon. In the literature survey, it was found that the properties of FEE emitters were greatly influenced by the surface decoration of various morphology nanostructures (ZnO-nanopillars,11 CdO nanosheets,40 Si nanowires,41 SiC nanowires,42 and graphene sheets43) with Au NPs, owing to increase in emitting sites and decrease of the work function. Au metal is well known for its low resistivity, high oxidation resistance, and high structural, electrical and chemical stability. The influence of the decoration of Au NPs on the properties of nanostructures of Bi2S3 FEE emitters has not been reported to date.
It has been observed from a literature survey that most nanostructured FEE emitters are decorated with Au NPs by a sputtering process.11,40–42,44 In this report, we follow a cost-effective and simple chemical method to decorate Au NPs on hydrothermally synthesized Bi2S3 NRs to explore the FEE performance. It is observed that the turn-on field of Au/Bi2S3 NRs drastically decreased from 3.7 to 2.7 V μm−1 at the current density of 1 μA cm−2 and the maximum current density increased from 138 to 604.8 μA cm−2 at an applied field of 7.8 V μm−1 as compared to that of pristine Bi2S3 NRs. Enhancement in FEE characteristics indicates that metal NP decoration could be an effective route to significantly enhance the FEE performances of the Bi2S3 cathodes.
2 Experimental
2.1 Synthesis of Bi2S3 nanoflowers
Bismuth sulfide (Bi2S3) micro-flowers were synthesised as per the literature report with slight modifications.45 In a typical synthesis, 2.4 g of bismuth nitrate [Bi(NO3)3·5H2O] (bismuth precursor) and 0.38 g of thiourea [CS(NH2)2] (sulfur precursor) were dissolved well into a mixture of 45 ml distilled water and 20 ml ethylene glycol (EG). The resultant solution was subjected to 30 minutes of stirring and 10 minutes of ultra-sonication at room temperature. This yellow homogeneous mixture was then transferred into a Teflon-lined stainless steel autoclave of 80 ml capacity and a few drops of concentrated HNO3 were added to it. The sealed autoclave was then kept at 180 °C for 36 hours in a muffle furnace. After the reaction, the resulting black precipitate was thoroughly washed (three times) with distilled water and absolute ethanol and collected by centrifugation (4000 rpm for 10 min). The final product was dried at 60 °C for 24 h in a vacuum. The schematic representation of the Bi2S3 microflower synthesis process is shown in Fig. 1a. The resultant black powder was used for further characterization and Au decoration.
2.2 Synthesis of Au/Bi2S3 nanostructures
Bi2S3 NRs were decorated with Au NPs by following a modified literature report.46 Briefly, 82 mg of as-synthesized Bi2S3 microflowers was dispersed in a 30 ml solvent mixture of 24 ml ethanol, 1.4 ml oleic acid (OA) and 4 ml octylamine. At the same time, 34 mg tetrachloroauric(III) acid (HAuCl4·3H2O) was dispersed into 10 ml of ethanol. Then, the gold precursor solution was added dropwise to the above mixture and stirred for 4 hours at about 50 °C. The grey precipitation was washed several times with ethanol, separated by centrifugation, and then dried overnight in a vacuum. The schematic representation of Au decoration of Bi2S3 NRs is shown in Fig. 1b. The collected sample (Au/Bi2S3) was used for further characterization and FEE comparison studies with pristine Bi2S3.
2.3 Field electron emission measurements
The FEE characteristics of pristine and Au/Bi2S3 samples were investigated at room temperature using an in-house developed setup. The distance between emitter sites (cathode) and phosphor screen (anode) is kept at 2 mm, with base pressure being maintained at ∼10−8 mbar during measurements. A high voltage power source (Spellman, U.S.) was used to supply the voltage between two electrodes. The voltage was increased by a step of 20 V and the corresponding increasing current was measured using an electrometer (Keithley 6514) with picoampere sensitivity.
3 Characterization of materials
To examine the crystalline phases of both structures, the X-ray diffraction pattern was obtained by using a Rigaku MicroMax-007 HF with a rotating anode copper X-ray source of wavelength λ Cu Kα = 1.54 Å which was operated at 40 kV and 30 mA. The morphological and structural characterizations were done using a field emission scanning electron microscope (FESEM, FEI Nova Nano SEM450) and transmission electron microscope. Further structural details and lattice fringe width calculations of both samples were accomplished by high-resolution transmission electron microscopy (HRTEM TEM, JEOL JEM-F200 operated at 200 kV accelerating voltage). X-ray photoelectron spectroscopy was achieved with the use of a near-ambient-pressure X-ray photoelectron spectrometer (XPS, Thermo K-Alpha+ Spectrometer using Al-Kα X-rays, 1486.6 eV) under ultra-high vacuum conditions to study elemental compositions of as-synthesized structures.
4 Results and discussion
4.1 XRD analysis
The phase and crystallinity of samples were studied by the X-ray diffraction technique. Fig. 2a and b show the typical XRD pattern of the as-prepared Au NP decorated Bi2S3 and pristine Bi2S3 sample respectively. The XRD pattern illustrates that the synthesized sample has an orthorhombic crystal structure with lattice parameters a = 3.981 Å, b = 11.14 Å, c = 11.30 Å (Fig. 2d, JCPDS # 17-0320) which also existed in the Au/Bi2S3 sample. The average crystallite size of Bi2S3 NRs was found to be ∼110 ± 10 nm, as calculated using the Debye–Scherer equation. Fig. 2a displays a peak at about 2θ = 38.10°, corresponding to the (111) plane of face-centered cubic (fcc) gold, (JCPDS # 04-0784), which gives the evidence for uniform gold decoration. The peak at about 2θ = 30.50° can be attributed to the characteristic peak of BiS2 (JCPDS #17-0267) indicating the negligible amount of BiS2 in the as-synthesized samples.
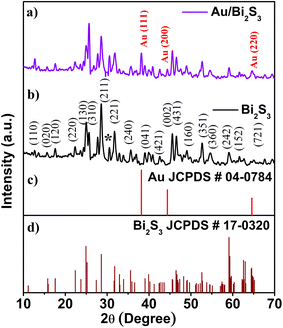 |
| Fig. 2 XRD of the (a) Au/Bi2S3 sample and (b) Bi2S3; (c) and (d) are JCPDS cards of Au and Bi2S3 crystals, respectively. | |
4.2 FESEM and TEM analysis
FESEM and TEM were used to examine surface morphology, size, and Au particle distribution on the as-prepared sample. Fig. 3a depicts an overview of the sample, where the Bi2S3 micro-flowers are observed with approximately ∼4 to 5 μm diameter. Fig. 3b shows typical images assembled from the number of NRs. The inset in Fig. 3b is the high -magnification FESEM image of Bi2S3 micro-flowers. It is seen from the TEM image (Fig. 3c) that bare Bi2S3 NRs have a smooth surface with length in few microns and about ∼120 nm in diameter, which is also endorsed by the average crystallite size calculated from the XRD pattern using the Debye–Scherer equation. The typical enlarged TEM image (Fig. 3d) of the Au decorated Bi2S3 nanorods reveals that the Au NPs were uniformly decorated over the entire surface of the Bi2S3 NRs without extended agglomerations. The high-resolution TEM (HRTEM) images displayed in Fig. 4a illustrate that the decorated Au NPs have an average diameter of 4–10 nm. The fine HRTEM fringes (Fig. 4b) reveal that the distance between two consecutive planes is 0.239 nm which is identical to the interplanar spacing of face-centered cubic (fcc) Au(111) planes.47Fig. 4c and d display the Selected Area Electron Diffraction (SAED) pattern of pristine Bi2S3 and Au/Bi2S3 NRs.
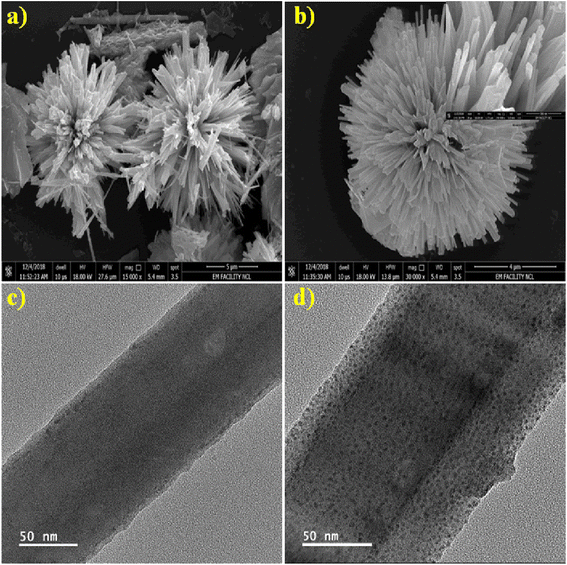 |
| Fig. 3 FESEM images: (a) low magnification Bi2S3 microflowers, (b) high magnification Bi2S3 with the inset displaying nanorods; TEM images (c and d) of bare Bi2S3 and Au decorated Bi2S3 NRs, respectively. | |
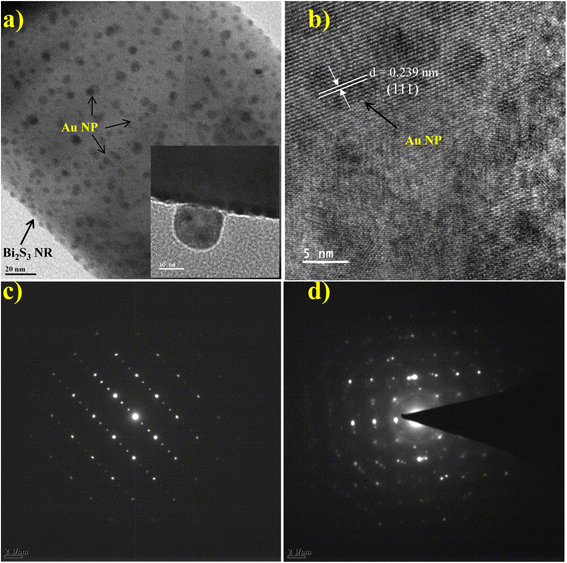 |
| Fig. 4 TEM image of (a) Au/Bi2S3 NRs with the inset displaying the NPs; (b) HRTEM image of Au/Bi2S3; (c and d) SAED pattern of bare Bi2S3 NRs and Au/Bi2S3 NRs, respectively. | |
4.3 XPS analysis
Surface chemical compositions and oxidation states of the as-synthesized product were investigated by XPS analysis. Fig. 5a shows the survey spectra of the sample with and without Au decoration, and gives strong evidence for the existence of Bi and S elements along with the successful decoration of Au on Bi2S3. The O and C peaks also arise due to the adsorbed oxygen species on the sample surface, which is commonly observed for samples exposed to the atmosphere and adsorbed carbon species during XPS measurement respectively. Adventitious C1s (284.6 eV) spectra were taken as the reference for calibration. Au 4f7/2 and 4f5/2 doublets with binding energies of 83.69 and 87.39 eV are observed in Fig. 5b, which asserts that decorated Au (Au0 state) is in the metallic form (ref. 48 and 49). As shown in Fig. 5c, the high-resolution spectrum of Bi4f (Bi3+ state) shows a doublet at 159.14 (4f7/2) and 164.39 eV (4f5/2) attributed to spin–orbital coupling separated by 5.31 eV.49 The binding energy for S2s (S2− state) (Fig. 5d) in the Au/Bi2S3 sample is at 224.1 eV which is lower than the binding energy of S2s (S2− state) (225.7 eV) in pristine Bi2S3 as per the literature.49 The Bi and S binding energies are in good agreement with reported values. It is observed that the Au decoration does not severely change the crystallinity of Bi2S3 NRs. All XPS plots validate the successful formation of Au/Bi2S3.
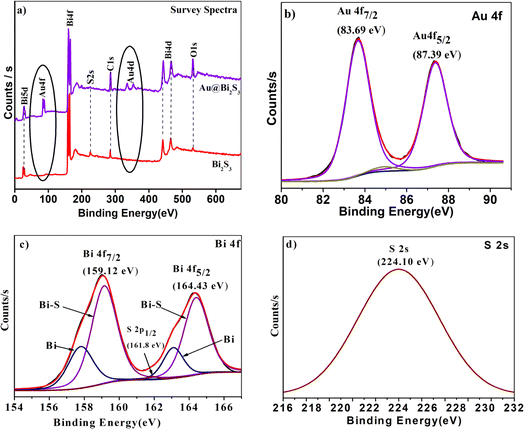 |
| Fig. 5 XPS images of (a) Bi2S3 and Au/Bi2S3 survey spectra; high resolution spectra of (b) Au0 state, (c) Bi3+ state, (d) S2− state of the Au/Bi2S3 sample. | |
4.4 Reaction mechanism
In our earlier report, we discussed the synthesis mechanism of Bi2S3 microflowers prepared by a simple one-pot hydrothermal method for FEE studies.31 For the decoration of Au NPs on Bi2S3 NRs, HAuCl4·3H2O was decomposed at 50 °C in an octylamine–oleic acid mixture under ambient conditions. In this reaction, the octylamine–oleic acid mixture acts as both a capping and a reducing agent, which prevents gold NP aggregation and oxidation, as well as makes the particle surface hydrophobic.50 The polar ethanol solvent was primarily used to dissolve HAuCl4·3H2O. The presence of the hydrocarbon surfactant between gold particles possibly prevents the growth of particles beyond 5–7 nm.51 To ensure the even mixing of the reactants, the solution was continuously stirred beyond the decoration time and also this ensured the uniform deposition of Au NPs onto the Bi2S3 surface as seen in TEM images (Fig. 3d). The pristine Bi2S3 and Au/Bi2S3 nanocomposites have been fully characterized and their FEE properties studied.
4.5 Field electron emission (FEE) performance
The dependence of FEE current density over an applied field (J–E) is described by the modified Fowler–Nordheim (F–N) equation:52 | 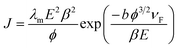 | (1) |
where J is the emission current density, E is the applied average electric field, λm is the macroscopic pre-exponential factor (=1.54 × 10−6 A eV V−2), b is a constant (=6.83 eV−3/2 V nm−1), ϕ is the work function of the emitter, β is the field enhancement factor, and νF (correction factor) is a particular value of the principal Schottky–Nordheim barrier function ν.
Eqn (1) asserts that FEE property enhancement can be achieved by either/both tuning the morphology or/and lowering the work function of the emitter. We have tried to improve the FEE performance of Bi2S3 NRs by Au decoration, resulting in reduced work function of Bi2S3.
The turn-on and threshold fields, measured from the J–E plot (Fig. 6a), are arbitrarily defined at emission current densities of 1 and 100 μA cm−2 respectively. The Au/Bi2S3 emitters show the turn-on and threshold fields of 2.7 and 5.2 V μm−1 respectively, whereas pristine Bi2S3 emitters show the turn-on and threshold fields of 3.7 and 6.8 V μm−1 respectively. It is obvious from the results that the FEE properties of pure Bi2S3 can be dramatically improved by Au decoration.
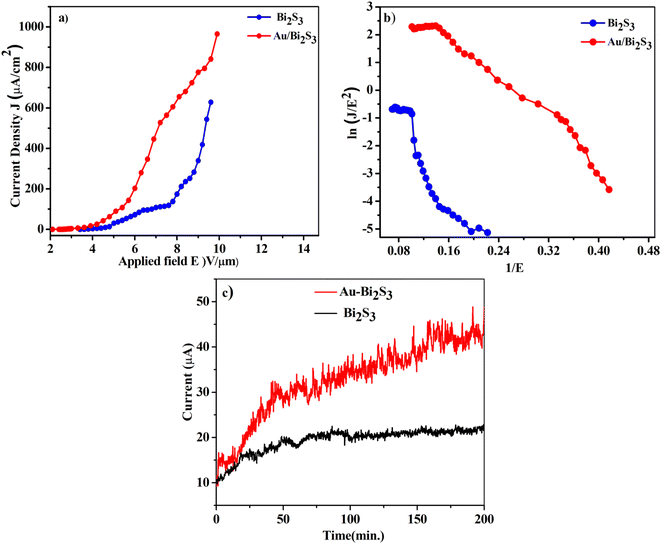 |
| Fig. 6 Plot of field emission current density versus applied electric field (J–E) (a), Fowler–Northeim (F–N) plot (b) of Bi2S3 and Au/Bi2S3, respectively. (c) Field emission current stability plots at 10 μA. | |
A wide range of research groups have reported a significant enhancement in FEE performance due to Au decoration on semiconductor materials.11,41–44 Zang et al.53 reported that the reduction of Au NP size below 10 nm decreases the work function up to 3.6 eV. In this work, decorated Au NPs have an average size of 4–10 nm, indicating that the work function of Au NPs would be around 3.6 eV, which is less than the work function of Bi2S3 NRs (4.93 eV).25 When Au (metal) and Bi2S3 (semiconductor) come into contact, they form a metal–semiconductor junction (Schottky barrier junction). The thermal equilibrium has been achieved through the transfer of electrons from Au (higher) to Bi2S3 (lower). The bending of the conduction band (CB) and valence band (VB) in Bi2S3 takes place due to the alignment of work functions. This energy band bending reduces the work function of the Bi2S3 emitter. Such work function reduction, due to NP decoration of the nanomaterial, shows an improvement of the FEE properties.54
The F–N plots (Fig. 6b) derived from the observed (J–E) curves show deviation from the linear nature, in contrast to the expectation of the F–N model. Such discrepancy has been observed in a diverse range of metal–semiconductor composites. This discrepancy can be supported by emission from the lower edge of the CB being dominant at a low field. On the other hand, at a high field, the emission current also contributes to the electrons in the upper edge of the CB.62 It is observed that the nonlinearity of the F–N plot diminishes in the Au/Bi2S3 emitter, indicating a more metallic behavior of the emitter. Furthermore, a careful observation reveals ‘flattening’ of the F–N plots in the high field region. For planar emitters, most of the researchers have noticed a ‘non-linear’ nature of the F–N plots, along with the tendency to show ‘flattening’ in the high field region, which had been attributed to various effects like the field screening effect, field penetration and band bending (for semiconducting emitters), etc. However, one of the root causes of such discrepancy is failure of the fundamental F–N model. It has been realized that the effect and/or contribution due to space charge limited currents has been ignored. Various researchers have focused their studies towards amending the fundamental F–N equation so as to justify the FEE behaviour of planar emitters. Herein, a planar emitter is referred to as an assembly of nanostructures deposited in a thin film form on suitable substrates. In tune with the advancement in planar emitter based electron sources and new devices, these alterations are important in providing better formulations for simulations.
In this context, Richard Forbes has put in significant and consistent efforts towards modification of the fundamental F–N model.52,63,64 In tune with these efforts, Zhang et al. have presented an overview of the fundamental physics of space–charge interactions in various media addressing the critical developments on various theoretical aspects of the space–charge limited current (SCLC) model, its physics at the nanoscale, and transitions between electron emission mechanisms and material properties.65 Very recently, in order to corroborate the advancements in utilization of electron sources, particularly simulations of the devices, Kevin Jensen has provided useful formulations considering the effect of “space–charge” (commonly described by the Child–Langmuir law) so as to guarantee correct numerical evaluation of the fundamental equations describing the various electron emission models.66
Furthermore, to showcase the technological importance of the observed FEE parameters, an attempt is made to compare the values of turn-on and threshold fields along with maximum emission current density extracted from similar emitters, Table1.
Table 1 FEE characteristic parameters of various semiconducting planar emitters
Sr. no. |
Emitter details |
Morphology |
Turn-on field or voltage |
Reference |
1 |
MoS2 |
Nanoflowers |
12 V μm−1 |
55
|
2 |
CNTs |
Nanotubes |
50 to 100 V |
56 and 57 |
3 |
Au/ZnO |
Nanowires |
6.2 V μm−1 |
58
|
4 |
AuBN |
Nanocomposites |
3.9 V μm−1 at 10 nA cm−2 |
59
|
5 |
Au/graphene |
Nanosheets |
3.84 V μm−1 |
60
|
6 |
Au/TiO2 |
Nanotubes |
2.8 V μm−1 at 10 μA cm−2 |
61
|
7 |
Au/Bi2S3 |
Micro-flowers |
2.7 V μm−1 at 1 μA cm−2 |
Present work |
Finally, to test the quality of the as-synthesized Au/Bi2S3 emitters in comparison with pristine Bi2S3, we have investigated the long-term current stability (I–t) plot. The stability of the emitters has been observed for more than 3 h corresponding to a current density of 10 μA cm−2. It is observed that fluctuations in current density for the Au/Bi2S3 emitter (the standard deviation of ∼3.86%) are somewhat more than for the bare Bi2S3 (the standard deviation of about ∼2.07%), possibly due to increase in emitting sites for the Au/Bi2S3 emitter. The fluctuation in electron emission current can be attributed to the adsorption/desorption and bombardments of ions/atoms on the emitter surface.67,68 The smoothness in the current stability (I–t) plot of the Au/Bi2S3 sample is also attributed to the protection of Bi2S3 NR emitters from ion/atom bombardments during the FEE mechanism due to the good chemical stability of Au NPs. The emitter also reveals good repeatability of results by testing the same samples several times.
5 Conclusions
In summary, we have reported the synthesis of Bi2S3 emitters with 120 ± 10 nm diameter, via a simple hydrothermal route followed by the uniform decoration of Au NPs with size ∼4–10 nm. FEE results show that the turn-on field of the Au/Bi2S3 emitter reduced from 3.7 to 2.7 V μm−1 at 1.0 μA cm−2 and the maximum current density increased from 138 to 604 μA cm−2 at field 7.8 V μm−1 as compared to that of pristine Bi2S3. The improved FEE performance of Au/Bi2S3 is attributed to the lowering of the work function of pristine Bi2S3 and increasing emission sites due to Au NP decoration. Therefore, Au decoration on Bi2S3 was achieved by a simple and cost-effective chemical method compared to the sputtering process. The Au decorated FEE emitters can be used as a potential candidate for flexible flat panel displays, efficient electron guns, and e-paper applications.
Data availability
All data generated or analyzed during this study are included in this published article and its ESI.†
Author contributions
GG and MD performed the synthesis experiments, characterization, and analysis. FEE analysis was done by SB and MM. GG wrote the first draft of the paper. GG, DL, and MM revised the writing of the manuscript and data calculations. RLML and J. Ribeiro-Soares in the revised the writing of the manuscript and data calculations. DL guided the research and conceived the final project.
Conflicts of interest
The authors declare no conflicts of interest.
Acknowledgements
This work was financially supported by the SPPU Research Foundation research grant. DJL thanks Foundation for Research of the State of Minas Gerais (UFLA, Brazil) - FAPEMIG (Project Grant No. APQ-04229-23) for funding. GG thanks Rashtriya Uchchatar Shiksha Abhiyan (RUSA) for research funding.
References
- W. Choi, D. Chung, J. Kang, H. Kim, Y. Jin, I. Han, Y. Lee, J. Jung, N. Lee and G. Park, Fully sealed, high-brightness carbon-nanotube field-emission display, Appl. Phys. Lett., 1999, 75(20), 3129–3131 CrossRef CAS.
- A. V. Crewe, M. Isaacson and D. Johnson, A simple scanning electron microscope, Rev. Sci. Instrum., 1969, 40(2), 241–246 CrossRef.
- F. Baker, A. Osborn and J. Williams, Field emission from carbon fibres: a new electron source, Nature, 1972, 239(5367), 96 CrossRef CAS.
- H. Zhang, J. Tang, Q. Zhang, G. Zhao, G. Yang, J. Zhang, O. Zhou and L. C. Qin, Field emission of electrons from single LaB6 nanowires, Adv. Mater., 2006, 18(1), 87–91 CrossRef CAS.
- S. Fan, M. G. Chapline, N. R. Franklin, T. W. Tombler, A. M. Cassell and H. Dai, Self-oriented regular arrays of carbon nanotubes and their field emission properties, Science, 1999, 283(5401), 512–514 CrossRef CAS.
- C. J. Lee, T. Lee, S. Lyu, Y. Zhang, H. Ruh and H. Lee, Field emission from well-aligned zinc oxide nanowires grown at low temperature, Appl. Phys. Lett., 2002, 81(19), 3648–3650 CrossRef CAS.
- A. S. Pawbake, R. T. Khare, J. O. Island, E. Flores, J. R. Ares, C. Sanchez, I. J. Ferrer, M. Pawar, O. Frank and M. A. More, Titanium Trisulfide Nanosheets and Nanoribbons for Field Emission-Based Nanodevices, ACS Appl. Nano Mater., 2023, 6(1), 44–49 CrossRef CAS.
- R. Patra, S. Ghosh, E. Sheremet, M. Jha, R. Rodriguez, D. Lehmann, A. Ganguli, H. Schmidt, S. Schulze and M. Hietschold, Enhanced field emission from lanthanum hexaboride coated multiwalled carbon nanotubes: correlation with physical properties, J. Appl. Phys., 2014, 116(16), 164309 CrossRef.
- S. R. Bansode, M. A. More and R. B. Sharma, ZnS–RGO nanocomposite structures: synthesis, characterization and field emission properties, New J. Chem., 2023, 47(5), 2273–2278 RSC.
- W. T. Zheng, Y. M. Ho, H. W. Tian, M. Wen, J. L. Qi and Y. A. Li, Field emission from a composite of graphene sheets and ZnO nanowires, J. Phys. Chem. C, 2009, 113(21), 9164–9168 CrossRef CAS.
- Y.-M. Chang, M.-L. Lin, T.-Y. Lai, H.-Y. Lee, C.-M. Lin, Y.-C. S. Wu and J.-Y. Juang, Field Emission Properties of Gold Nanoparticle-Decorated ZnO Nanopillars, ACS Appl. Mater. Interfaces, 2012, 4(12), 6676–6682 CrossRef CAS.
- X. Yu, J. Zhu, Y. Zhang, J. Weng, L. Hu and S. Dai, SnSe2 quantum dot sensitized solar cells prepared employing molecular metal chalcogenide as precursors, Chem. Commun., 2012, 48(27), 3324–3326 RSC.
- M. Acerce, D. Voiry and M. Chhowalla, Metallic 1T phase MoS2 nanosheets as supercapacitor electrode materials, Nat. Nanotechnol., 2015, 10(4), 313 CrossRef CAS.
- K. Zhang, Z. Hu, X. Liu, Z. Tao and J. Chen, FeSe2 microspheres as a high performance anode material for Na ion batteries, Adv. Mater., 2015, 27(21), 3305–3309 CrossRef CAS PubMed.
- R. Caracas and X. Gonze, First-principles study of the electronic properties of A2B3 minerals, with A = Bi,Sb and B = S,Se, Phys. Chem. Miner., 2005, 32(4), 295–300 CrossRef CAS.
- G. Chen, Y. Yu, K. Zheng, T. Ding, W. Wang, Y. Jiang and Q. Yang, Fabrication of Ultrathin Bi2S3 Nanosheets for High Performance, Flexible, Visible–NIR Photodetectors, Small, 2015, 11(24), 2848–2855 CrossRef CAS.
- B. Xu, G. Wang and H. Fu, Enhanced photoelectric conversion efficiency of dye-sensitized solar cells by the incorporation of flower-like Bi2S3:Eu3+ sub-microspheres, Sci. Rep., 2016, 6, 23395 CrossRef CAS.
- A. Sarkar, A. B. Ghosh, N. Saha, A. K. Dutta, D. N. Srivastava, P. Paul and B. Adhikary, Enhanced photocatalytic activity of Eu-doped Bi2 S3 nanoflowers for degradation of organic pollutants under visible light illumination, Catal. Sci. Technol., 2015, 5(8), 4055–4063 RSC.
- J. Zhou, G. Tian, Y. Chen, Y. Shi, C. Tian, K. Pan and H. Fu, ERRATUM: Growth rate controlled synthesis of hierarchical Bi2S3/In2S3 core/shell microspheres with enhanced photocatalytic activity, Sci. Rep., 2014, 4, 4027 CrossRef PubMed.
- S. P. Vattikuti, A. K. R. Police, J. Shim and C. Byon, Sacrificial-template-free synthesis of core–shell C@Bi2 S3 heterostructures for efficient supercapacitor and H2 production applications, Sci. Rep., 2018, 8(1), 4194 CrossRef.
- C. S. Rout, P. D. Joshi, R. V. Kashid, D. S. Joag, M. A. More, A. J. Simbeck, M. Washington, S. K. Nayak and D. J. Late, Enhanced field emission properties of doped graphene nanosheets with layered SnS2, Appl. Phys. Lett., 2014, 105(4), 043109 CrossRef.
- C. S. Rout, P. D. Joshi, R. V. Kashid, D. S. Joag, M. A. More, A. J. Simbeck, M. Washington, S. K. Nayak and D. J. Late, Superior field emission properties of layered WS2-RGO nanocomposites, Sci. Rep., 2013, 3, 3282 CrossRef PubMed.
- C. Song, K. Yu, H. Yin, H. Fu, Z. Zhang, N. Zhang and Z. Zhu, Highly efficient field emission properties of a novel layered VS2/ZnO nanocomposite and flexible VS2 nanosheet, J. Mater. Chem. C, 2014, 2(21), 4196–4202 RSC.
- J. Li, K. Yu, Y. Tan, H. Fu, Q. Zhang, W. Cong, C. Song, H. Yin and Z. Zhu, Facile synthesis of novel MoS2@SnO2 hetero-nanoflowers and enhanced photocatalysis and field-emission properties, Dalton Trans., 2014, 43(34), 13136–13144 RSC.
- P. K. Bankar, M. S. Pawar, A. S. Pawbake, S. S. Warule, D. J. Late and M. A. More, Spatially branched CdS–Bi2 S3 heteroarchitecture: single step hydrothermal synthesis approach with enhanced field emission performance and highly responsive broadband photodetection, RSC Adv., 2016, 6(97), 95092–95100 RSC.
- S. R. Bansode, K. V. Harpale, P. Mutadak, K. M. Sonawane, M. G. Chaskar, M. A. More and R. B. Sharma, Morphology-dependent field emission investigations from the 2-dimensional Bi2Se3-RGO nanocomposites, Mater. Sci. Eng., B, 2021, 274, 115450 CrossRef CAS.
- X. Yu and C. Cao, Photoresponse and field-emission properties of bismuth sulfide nanoflowers, Cryst. Growth Des., 2008, 8(11), 3951–3955 CrossRef CAS.
- Y. Yu, C. Jin, R. Wang, Q. Chen and L.-M. Peng, High-quality ultralong Bi2S3 nanowires: structure, growth, and properties, J. Phys. Chem. B, 2005, 109(40), 18772–18776 CrossRef CAS PubMed.
-
S. S. Warule, R. Kashid, D. Shinde, N. S. Chaudhari, B. Kale and M. More, Architectured Bi2S3 nanoflowers: photoenhanced field emission study, 2012, 14.
-
Y. Li, S. Nambiar, Y. Sun, C. N. R. Rao and J. T. W. Yeow, In Experimental study on field emission performance of bismuth sulfide nanoflowers, 14th IEEE International Conference on Nanotechnology, 2014, pp. 1–4 Search PubMed.
- G. H. Gote, S. R. Bhopale, M. A. More and D. J. Late, Realization of Efficient Field Emitter Based on rGO-Bi2S3 Heterostructures, Phys. Status Solidi A, 2019, 216(18), 1900121 CrossRef.
- P. Wang, B. Huang, X. Zhang, X. Qin, H. Jin, Y. Dai, Z. Wang, J. Wei, J. Zhan and S. Wang, Highly efficient visible light plasmonic photocatalyst Ag@AgBr, Chem.–Eur. J., 2009, 15(8), 1821–1824 CrossRef CAS PubMed.
- Y. Tian and T. Tatsuma, Mechanisms and applications of plasmon-induced charge separation at TiO2 films loaded with gold nanoparticles, J. Am. Chem. Soc., 2005, 127(20), 7632–7637 CrossRef CAS.
- L.-B. Luo, C. Xie, X.-H. Wang, Y.-Q. Yu, C.-Y. Wu, H. Hu, K.-Y. Zhou, X.-W. Zhang and J.-S. Jie, Surface plasmon resonance enhanced highly efficient planar silicon solar cell, Nano Energy, 2014, 9, 112–120 CrossRef CAS.
- V. E. Ferry, J. N. Munday and H. A. Atwater, Design considerations for plasmonic photovoltaics, Adv. Mater., 2010, 22(43), 4794–4808 CrossRef CAS PubMed.
- K. Okamoto, I. Niki, A. Shvartser, Y. Narukawa, T. Mukai and A. Scherer, Surface-plasmon-enhanced light emitters based on InGaN quantum wells, Nat. Mater., 2004, 3(9), 601 CrossRef CAS.
- L.-B. Luo, L.-H. Zeng, C. Xie, Y.-Q. Yu, F.-X. Liang, C.-Y. Wu, L. Wang and J.-G. Hu, Light trapping and surface plasmon enhanced high-performance NIR photodetector, Sci. Rep., 2014, 4, 3914 CrossRef.
- C.-C. Chang, Y. D. Sharma, Y.-S. Kim, J. A. Bur, R. V. Shenoi, S. Krishna, D. Huang and S.-Y. Lin, A surface plasmon enhanced infrared photodetector based on InAs quantum dots, Nano Lett., 2010, 10(5), 1704–1709 CrossRef CAS.
- J. Lin, H. Li, H. Zhang and W. Chen, Plasmonic enhancement of photocurrent in MoS2 field-effect-transistor, Appl. Phys. Lett., 2013, 102(20), 203109 CrossRef.
- V. S. Bagal, G. P. Patil, A. B. Deore, S. R. Suryawanshi, D. J. Late, M. A. More and P. G. Chavan, Surface modification of aligned CdO nanosheets and their enhanced field emission properties, RSC Adv., 2016, 6(47), 41261–41267 RSC.
- F. Zhao, G.-a. Cheng, R.-t. Zheng, D.-d. Zhao, S.-l. Wu and J.-h. Deng, Field emission enhancement of Au–Si nano-particle-decorated silicon nanowires, Nanoscale Res. Lett., 2011, 6(1), 176 CrossRef PubMed.
- Q. Chen, S. Chen, F. Gao, L. Wang, Z. Xie and W. Yang, Enhanced field emission of Au nanoparticle-decorated SiC nanowires, J. Mater. Chem. C, 2016, 4(7), 1363–1368 RSC.
- L. Chen, H. He, D. Lei, Q. Menggen, L. Hu and D. Yang, Field emission performance enhancement of Au nanoparticles doped graphene emitters, Appl. Phys. Lett., 2013, 103(23), 233105 CrossRef.
- H. Chen, H. Zhang, L. Fu, Y. Chen, J. S. Williams, C. Yu and D. Yu, Nano Au-decorated boron nitride nanotubes: Conductance modification and field-emission enhancement, Appl. Phys. Lett., 2008, 92(24), 243105 CrossRef.
- Y. Tian, T.-t. Ding, X.-l. Zhu, Y.-f. Tu and G. Zheng, Bi2S3 microflowers assembled from one-dimensional nanorods with a high photoresponse, J. Mater. Sci., 2015, 50(16), 5443–5449 CrossRef CAS.
- J. L. Chen, V. Nalla, G. Kannaiyan, V. Mamidala, W. Ji and J. J. Vittal, Synthesis and nonlinear optical switching of Bi2S3 nanorods and enhancement in the NLO response of Bi2S3@Au nanorod-composites, New J. Chem., 2014, 38(3), 985–992 RSC.
- X. Huang, S. Li, Y. Huang, S. Wu, X. Zhou, S. Li, C. L. Gan, F. Boey, C. A. Mirkin and H. Zhang, Synthesis of hexagonal close-packed gold nanostructures, Nat. Commun., 2011, 2, 292 CrossRef PubMed.
- J. Luo, Y. Liu, Y. Niu, Q. Jiang, R. Huang, B. Zhang and D. Su, Insight into the chemical adsorption properties of CO molecules supported on Au or Cu and hybridized Au–CuO nanoparticles, Nanoscale, 2017, 9(39), 15033–15043 RSC.
- S. K. Saha and A. J. Pal, Schottky diodes between Bi2S3 nanorods and metal nanoparticles in a polymer matrix as hybrid bulk-heterojunction solar cells, J. Appl. Phys., 2015, 118(1), 014503 CrossRef.
- P. d. l. Presa, M. Multigner, J. d. l. Venta, M. A. García and M. L. Ruiz-González, Structural and magnetic characterization of oleic acid and oleylamine-capped gold nanoparticles, J. Appl. Phys., 2006, 100(12), 123915 CrossRef.
-
Y. Min, M. Akbulut, K. Kristiansen, Y. Golan and J. Israelachvili, The role of interparticle and external forces in nanoparticle assembly, in Nanoscience and Technology: A Collection of Reviews from Nature Journals, World Scientific, 2010, pp. 38–49 Search PubMed.
- R. G. Forbes, Extraction of emission parameters for large-area field emitters, using a technically complete Fowler–Nordheim-type equation, Nanotechnology, 2012, 23(9), 09570 CrossRef PubMed.
- Y. Zhang, O. Pluchery, L. Caillard, A.-F. Lamic-Humblot, S. Casale, Y. J. Chabal and M. Salmeron, Sensing the Charge State of Single Gold Nanoparticles via Work Function Measurements, Nano Lett., 2015, 15(1), 51–55 CrossRef CAS PubMed.
- C. Ye, Y. Bando, X. Fang, G. Shen and D. Golberg, Enhanced field emission performance of ZnO nanorods by two alternative approaches, J. Phys. Chem. C, 2007, 111(34), 12673–12676 CrossRef CAS.
- F. Giubileo, A. Grillo, M. Passacantando, F. Urban, L. Iemmo, G. Luongo and A. Di Bartolomeo, Field emission characterization of MoS2 nanoflowers, Nanomaterials, 2019, 9(5), 717 CrossRef CAS PubMed.
- F. Giubileo, A. Di Bartolomeo, L. Iemmo, G. Luongo and F. Urban, Field emission from carbon nanostructures, Appl. Sci., 2018, 8(4), 526 CrossRef.
- S. B. Fairchild, T. A. de Assis, J. H. Park, M. Cahay, J. Bulmer, D. E. Tsentalovich and M. Pasquali, Strongly anisotropic field emission from highly aligned carbon nanotube films, J. Appl. Phys., 2021, 129(12), 125103 CrossRef CAS.
-
K. Zheng, Z. Zhang, X. Li, J. She, S. Deng, N. Xu and J. Chen, Field emission from Au nanoparticles decorated ZnO nanowires, in 2018 31st International Vacuum Nanoelectronics Conference (IVNC), IEEE, 2018, pp. 1–2 Search PubMed.
- H. Chen, H. Zhang, L. Fu, Y. Chen, J. S. Williams, C. Yu and D. Yu, Nano Au-decorated boron nitride nanotubes: Conductance modification and field-emission enhancement, Appl. Phys. Lett., 2008, 92(24), 243105 CrossRef.
- J. Xu, Q. Wang, Z. Tao, Z. Qi, Y. Zhai, W. Lei and X. Zhang, Enhanced electron emission of directly transferred few-layer graphene decorated with gold nanoparticles, RSC Adv., 2016, 6(81), 78170–78175 RSC.
- G. P. Patil, V. S. Bagal, S. R. Suryawanshi, D. J. Late, M. A. More and P. G. Chavan, Observation of enhanced field emission properties of Au/TiO2 nanocomposite, Appl. Phys. A: Mater. Sci. Process., 2016, 122, 1–11 CrossRef CAS.
- A. A. Al-Tabbakh, M. A. More, D. S. Joag, I. S. Mulla and V. K. Pillai, The Fowler–Nordheim plot behavior and mechanism of field electron emission from ZnO tetrapod structures, ACS Nano, 2010, 4(10), 5585–5590 CrossRef CAS PubMed.
- R. G. Forbes, The theoretical link between voltage loss, reduction in field enhancement factor, and Fowler–Nordheim-plot saturation, Appl. Phys. Lett., 2017, 110(13), 133109 CrossRef.
- T. A. de Assis, F. F. Dall'Agnol and R. G. Forbes, Field emitter electrostatics: a review with special emphasis on modern high-precision finite-element modelling, J. Phys.: Condens. Matter, 2022, 34(49), 493001 CrossRef CAS PubMed.
- P. Zhang, Y. S. Ang, A. L. Garner, Á. Valfells, J. W. Luginsland and L. K. Ang, Space–charge limited current in nanodiodes: ballistic, collisional, and dynamical effects, J. Appl. Phys., 2021, 129(10), 100902 CrossRef CAS.
- K. L. Jensen, Tutorial: the equations of electron emission and their evaluation, J. Appl. Phys., 2024, 135(11), 111101 CrossRef CAS.
- C. Kleint, Surface diffusion model of adsorption-induced field emission flicker noise: I. Theory, Surf. Sci., 1971, 25(2), 394–410 CrossRef CAS.
- H. Todokoro, N. Saitou and S. Yamamoto, Role of ion bombardment in field emission current instability, Jpn. J. Appl. Phys., 1982, 21(10R), 1513 CrossRef CAS.
|
This journal is © The Royal Society of Chemistry 2025 |
Click here to see how this site uses Cookies. View our privacy policy here.