DOI:
10.1039/D4NR03135K
(Paper)
Nanoscale, 2025,
17, 345-360
Copper–cobalt peroxide nanoparticles: a biomimetic cascade reaction for enhanced Fenton-like therapy at physiologically relevant pH†
Received
30th July 2024
, Accepted 19th October 2024
First published on 29th October 2024
Abstract
Traditional Fenton-like reactions, commonly employed in chemodynamic therapy (CDT) for cancer treatment, face limitations due to the mildly acidic tumor microenvironment (TME) and scarce H2O2 availability. Aiming to overcome these hurdles, we report herein the preparation of copper–cobalt peroxide (CCp) nanoparticles, a novel catalyst that enables a pH-activated, self-supplying H2O2-mediated cascade reaction. In the slightly acidic TME (pH 6.5–7.0), CCp nanoparticles degrade, generating H2O2in situ. This intrinsic H2O2 production eliminates the need for external H2O2 sources and enables activation in a significantly higher pH range. Simultaneously, released Cu and Co ions, primarily in lower oxidation states, synergistically drive a catalytic loop for sustained hydroxyl radical (˙OH) production. The non-ferrous bimetallic approach exhibits exquisite pH sensitivity and self-sufficiency, surpassing traditional Fenton reactions. Comparative studies highlight CCp's superior performance against copper-based bimetallic peroxides containing Fe and Ce, confirming the synergistic power of Cu–Co pairing. In vitro experiments demonstrate that the synthesized CCp-NPs exhibit greater toxicity toward breast cancer cells (4T1) than towards non-cancerous cells, showcasing their therapeutic potential. Furthermore, CCp-NPs outperform other nanoparticles in inhibiting cancer cell proliferation, colony formation, and migration. Density Functional Theory (DFT) calculations suggest that Co doping enhances CCp's ability to participate in Fenton reactions. Overall, this work is pioneering in relation to the design of a new class of smart nanoparticles for CDT. The combination of self-generated H2O2, high pH activation, and synergistic metal effects in CCp opens the door for next-generation cancer theranostic nanoparticles with unprecedented efficiency and precision, minimizing side effects.
1. Introduction
Despite improvements in overall human health, cancer continues to be a significant threat to life.1,2 Breast cancer, the leading malignancy in women globally, exhibits a high propensity for bone metastasis in advanced stages.3 Unfortunately, current treatment options for cancer, such as chemotherapy (CT), radiotherapy (RT), photothermal therapy (PTT), and surgical resection, often fall short due to limited effectiveness and significant side effects.4 For example, chemotherapy often requires high doses, leading to a plethora of side effects, such as myelosuppression, nausea, vomiting, hair loss and drug resistance.5 The limitations of existing therapies, particularly their impact on healthy tissues, have prompted the exploration of newer approaches such as photodynamic therapy (PDT)6 and chemodynamic therapy (CDT).7–10 However, PDT encounters hurdles in clinical translation due to limited light penetration and the hypoxic nature of solid tumors.11 On the other hand, CDT is a cancer treatment strategy that utilizes Fenton-like reactions to generate highly reactive hydroxyl radicals (˙OH) with cytotoxic effects.12–20 This approach is attracting increasing interest due to its ability to induce apoptosis in cancer cells while minimizing potential harm to healthy tissues. CDT utilizes reactive oxygen species (ROS), specifically hydroxyl radicals (˙OH), to kill tumor cells without relying on oxygen for its action.21,22 The tumor microenvironment (TME)23 provides an ideal environment for this process, as it naturally holds higher concentrations of hydrogen ions (acidic media pH ≈ 6.5) and hydrogen peroxide (H2O2) (1–100 μM) compared to those in healthy tissues.24 These conditions facilitate the Fenton reaction, a key component of CDT's tumor-killing mechanism. Exploiting these distinct properties of the TME (i.e. elevated hydrogen ion and hydrogen peroxide concentrations), CDT effectively targets and eliminates tumor cells while minimizing damage to healthy tissues.25–27 This targeted approach, coupled with its unique features, such as endogenous stimulation activation, high tumor specificity and ability to regulate the TME, offers significant advantages over traditional therapies.28–30 Despite the potential of metal ions like Fe2+, Cu2+, Mn2+, Sn2+, Co2+, and Ce4+ in Fenton-based cancer treatments,19,21,22,31–38 their insufficient catalytic efficiency has led to the development of various strategies to enhance their effectiveness. For example, the classical Fenton reaction based on iron has two main disadvantages: the first one is the slow reduction reaction rate of Fe(III) with H2O2 (kFe(III)/H2O2 = 0.001–0.01 M−1 s−1) and the second is the effectiveness of this reaction only under highly acidic conditions (pH ∼ 2–4)39,40 due to precipitation of iron at higher pH. Moreover, the concentration of endogenously produced hydrogen peroxide in cancerous cells is 100 μM, which is not sufficient for continuous generation of active oxygen species (ROS).21,41,42 Based on the above, possible solutions to enhance CDT performance through Fenton reactions should include (1) reactions that work at pH values typical of the TME and (2) reactions able to self-supply hydrogen peroxide to compensate for the shortage of intratumoral H2O2 levels.21
Recently, different strategies such as heterogeneous catalysis, chelating ligand addition and iron cycling have been put forth to solve the aforementioned problems.39 On the other hand, it is widely known that multicomponent metallic composites can achieve enhanced catalytic performance, leading to higher activity and selectivity, compared to single-component materials. This is due to the “synergetic catalytic effect,” which has been extensively studied in the field of catalysis.39,43–47
Metal peroxides,48 compounds where metal ions bond with peroxide groups (O22−) through ionic or covalent interactions, hold promising potential as alternative sources of hydrogen peroxide in acidic environments.32 In recent advancements, Zhang21 and Hyeon40 have proposed a straightforward approach for synthesizing metal-doped cerium (M-CeOx) and copper–iron (Cu–Fe) peroxides, respectively. These innovative materials offer promising solutions for self-generating hydrogen peroxide and potentially enhance CDT efficacy. In general, two crucial factors govern the selection of metals for synthesizing bimetallic peroxides for enhancing CDT efficacy: (1) multiple oxidation states, with candidate metals exhibiting diverse oxidation states such as Ce3+/Ce4+, Fe2+/Fe3+, and Co2+/Co3+, and (2) suitable reduction potential, where the synergistic catalytic effect in Fenton/Fenton-like reactions arises when the reduction potential of chosen metals leads to a more positive ΔErxn (internal energy change of a chemical reaction) and correspondingly a more negative ΔGrxn (free energy change of a chemical reaction).49,50
Several studies have demonstrated that Cu+ can efficiently catalyze Fenton-like reactions under mildly acidic and neutral conditions.7,51 Notably, the reaction rate of Cu+ is significantly higher than that of Fe2+, reaching up to 1 × 104 M−1 s−1, which represents a nearly 160-fold increase. Additionally, cobalt (Co2+), an essential trace element and a crucial component of vitamin B12, has been shown to outperform Fe2+ as a Fenton-like catalyst for converting H2O2 into hydroxyl radicals (˙OH) within tumor cells for more effective CDT.52,53
Based on the above, we report herein a novel and robust CDT system based on copper–cobalt metal peroxide nanoparticles (CCp NPs). The synthesis of these nanoparticles, facilitated by hydroxyl groups (OH−) under room temperature and pressure, utilizes polyvinylpyrrolidone (PVP) to ensure their stability. CCp exhibits a synergistic catalytic activity for Fenton-like reactions within the tumor microenvironment. We anticipate that the presence of hydroxyl groups (OH−) in basic media will induce the deprotonation of H2O2, thereby facilitating its coordination with the metallic components of the nanoparticles. Employing the same strategy, two types of additional bimetallic peroxide nanoparticles, CuFe and CuCe, were synthesized. The selection of these metals was based on the availability of their different oxidation states and reduction potentials. We then compared and analyzed the Fenton-like catalytic activities of the synthesized nanoparticles. Our findings demonstrate that the optimal combination of Cu and Co at a ratio of 7
:
3 in the CCp NPs produces the highest Cu+/Cu2+ ratio, resulting in the most efficient catalytic agent for ultrasensitive chemodynamic therapy. Furthermore, the Fenton-like catalytic activity of CCp NPs significantly surpasses that of monometallic Cu and other bimetallic counterparts, particularly at a pH of 7.0. This superior performance suggests the potential of this composite for in situ self-generation of H2O2, effectively addressing the limited endogenous H2O2 levels within tumor tissues. The metal peroxide clusters served as models for the actual systems under study by DFT calculations. The obtained results revealed that a critical Cu/Co ratio exists that balances this enhancement with the preservation of robust O–O bonds within the cluster. These O–O bonds are essential for the self-production of H2O2. Mechanistically, Co doping can influence the electronic structure of oxygen, leading to an increase in its charge density and enhanced nucleophilicity under acidic conditions. In vitro cell culture studies were performed on the breast cancer cell line 4T1 and the non-cancerous cell line HFF. The cell cytotoxicity of the nanoparticles was investigated using the MTT assay and fluorescence cell proliferation assay. All nanoparticles showed higher toxicity in cancer cells than in non-cancerous cells. Moreover, CCp NPs exhibit excellent anti-tumor activity when compared with other nanoparticles. CCp NPs also significantly inhibit cell migration and almost completely prevent colony formation in cancer cells.
2. Materials and methods
2.1. Materials
All reagents and solvents were obtained commercially and used without further purification. Cobalt(II) chloride hexahydrate (CoCl2·6H2O), copper(II) chloride dihydrate (CuCl2·2H2O), cerium(III) chloride heptahydrate (CeCl3·7H2O), ferric chloride hexahydrate (FeCl3·6H2O), polyvinylpyrrolidone (PVP, K30), hydrogen peroxide (H2O2, 30%) solution, 3,3′,5,5′-tetramethylbenzidine (TMB), potassium permanganate (KMnO4), sulfuric acid (H2SO4), and sodium hydroxide (NaOH) were purchased from Sigma-Aldrich. Besides, deionized water was used in all experiments (resistivity > 18 MΩ cm). All culture media were purchased from Bio Idea (Ide-Zist Notarkib Company, Iran). Fetal bovine serum (FBS), trypsin–EDTA, and penicillin–streptomycin were obtained from Gipco (USA). Phosphate buffered saline (PBS) tablets, 3-[4,5-dimethylthiazole-2-yl]-2,5-diphenyltetrazolium bromide (MTT) powder and dimethyl sulfoxide (DMSO) were obtained from Sigma-Aldrich (USA). Cell staining dyes were from ChemoMetec (Denmark) and crystal violet was from Merck (Germany).
2.2. Characterization analysis
The morphology of CCp NPs was characterized using a transmission electron microscope (JEOL JEM-2100F), which was operated at an acceleration voltage of 200 kV. Elemental mapping and EDS analysis were performed by scanning electron microscopy using a TESCAN-Vega 3. Hydrodynamic size measurements and zeta potential were determined using a Cilas Zetasizer Nano-DS system. XPS spectra were obtained by X-ray photoelectron spectroscopy using an ESCALAB 250 from Thermo Fisher Scientific. The concentrations of copper and cobalt metal ions were measured by Atomic Absorption Spectroscopy (AAS) using an Analytik Jena. The UV-vis absorption spectra were obtained using an Analytik Jena SPECORD 250 spectrophotometer. Fourier transform infrared spectra (FT-IR) were acquired using a Jasco 4600 spectrometer. Raman spectra were obtained in the range of 400–1100 cm−1 using a LabRam HR Confocal Raman Spectrometer from Horiba.
2.3. Synthesis of copper-based peroxide nanoparticles
PVP (3 g) was dissolved in 15 ml of distilled water to create a homogeneous solution. For the synthesis of CCp NPs with various Cu-to-Co ratios, mixtures of two aqueous solutions containing CuCl2·2H2O (0.02 M) and CoCl2·6H2O (0.02 M) at appropriate ratios were added to the above solution. The resulting mixture was stirred for several minutes (20 min) to ensure homogeneity. Afterwards, NaOH (30 ml, 0.02 M) and H2O2 (600 μL, 9.8 M) were added sequentially to the mixture and stirred quickly for 30 minutes. Once the reaction was complete, the CCp NPs were isolated using ultracentrifugation and then filtered using a 0.2 μm filter. Following a similar procedure, copper-ferric nanoparticles, CFp-NPs, and copper–cerium nanoparticles, CCep-NPs, were also prepared and studied. Besides, copper peroxide, Cup, was synthesized as the control and reference sample.
2.4. Colorimetric assay of peroxo groups
In order to confirm the existence of peroxo groups, the synthesized Cu-based NPs were treated with an aqueous KMnO4 solution (40 μg ml−1) containing 0.1 M H2SO4 for 5 minutes. The same procedure was done for cobalt and copper (CuO and CoO). Finally, the UV-vis spectra were recorded from 400 to 650 nm.32,40
2.5. OH generation activity of a Cu based NP driven Fenton-like reaction
CCp-NPs were added into TMB (0.3 mg ml−1) as a sensitive probe for the characterization of peroxidase activity at various pH values (5.4, 6.6, 7.0 and 7.4) for 45 minutes. This substrate yields a blue reaction product that has maximum absorbance at 650 nm.21,32 For the sake of comparison with CCp NPs, CFp and CCep NPs were studied at two different pH values (acidic pH 5.4 and neutral pH 7.0).
2.6. Computational details
The calculations were done using the Vienna Ab initio Simulation Package (VASP)54,55 with the generalized gradient approximation (GGA)56 and Perdew–Burke–Ernzerhof (PBE)57 functional. A plane-wave cutoff energy of 400 eV was used with a convergence criterion of 10−4 eV for the electronic self-consistent loop to ensure high accuracy. Spin polarization was considered for all atoms. For the cluster models, a 15 Å × 15 Å × 15 Å box size was applied with a 1 × 1 × 1 K-point mesh. First, cubic Cu32O64 and Co32O64 clusters were optimized as control models. Then, Co atoms were doped at different possible positions in the Cu32O64 cluster, one at a time. After each doping, the structure was electronically optimized, and the most stable structure was selected for the next doping.
Adsorption energies of a proton (H+) on Cu32O64 and Cu22Co10O64 clusters were calculated employing the following equation:
Eads(tot) = E(cluster + H+) − E(cluster) − E(H+) |
where
Eads(tot) is the total adsorption energy of the proton and cluster, while
E(cluster + H
+) is the total energy of the cluster in the presence of a positive charge of the proton, and
E(H
+) is the energy of the isolated proton. According to this definition, a more negative energy corresponds to stable proton adsorption/stability of the cluster in the presence of a proton.
2.7. Cell culture studies
2.7.1. Cell lines.
Cell culture studies were performed using 4T1, as a breast cancer cell line, and HFF, as a non-cancerous cell line. The 4T1 and HFF cell lines were grown in 25 cm2 T flasks containing RPMI and high glucose DMEM culture media, respectively. Penicillin–streptomycin (1%) and fetal bovine serum (10%) were added for supplementation of culture media. Culture flasks were maintained in an incubator at 37 °C under 5% CO2 and 95% humidity. The cells were detached using 0.25% trypsin–EDTA solution when their confluency reached 80–90%.
2.7.2. MTT assay.
The cytotoxicity of NPs on cells was assessed using the MTT assay. Briefly, 6 × 103 cells were seeded in each well of 96 well plates and maintained at 37 °C in a CO2 incubator for 24 h. The cells were treated with different doses (0.312, 0.625, 1.25, 2.5, 5 and 10 mg ml−1) of NPs. After 24 h, the medium of plates was withdrawn and replaced with 75 μl of medium containing 0.5 mg ml−1 of 3-[4,5-dimethylthiazol-2-yl]-2,5-diphenyl tetrazolium bromide (MTT). The plates were maintained in an incubator for 4 h. Accordingly, plate media was replaced with 75 μl of dimethyl sulfoxide (DMSO) and shaken for 20 minutes at room temperature. The absorbance (optical density) readings of the plates were measured using an ELISA reader (CLARIOstar, BMG LABTECH, Germany) at 570 nm. Viability was calculated via the following formula:
2.7.3. Fluorescence cell proliferation assay.
Cells were cultured in 6 well plates (2 × 105 per well) and allowed to incubate for 24 h to facilitate plate attachment. The cells were treated with 2.5 mg ml−1 of NPs and incubated for 24 h. After that, the cells were harvested and washed with PBS and stained with acridine orange (AO) and propidium iodide (PI) dyes. The stained cells were observed under an inverted fluorescent microscope (KERN, Germany). The PI-stained cells are seen in red and the AO-stained cells are seen in green. Thus, in the merged image of AO and PI channels, green cells are viable and red, orange and yellow cells are dead.
2.7.4. Colony formation assay.
The survival ability of single cancer cells to generate colonies was investigated using a colony formation (clonogenic) assay. Briefly, 4T1 cancer cells were cultured in 6-well plates with a density of 800 cells per well and treated with 2.5 mg ml−1 of NPs after cell attachment. The plates were maintained in an incubator for 6 days. The plates were washed with PBS and subsequently 1 ml of 0.5% crystal violet (dissolved in 50% methanol) was added to each well and incubated at room temperature for 1 h. Then, the plates were washed with PBS 3 times to remove any excess crystal violet. Finally, photographs of the plates were taken and the number of colonies was determined with ImageJ 1.44p software. Only considerable colonies in size (approximately ≥50 densely-packed cells) were counted.
2.7.5. Caspase 3,7 activity assay.
The Caspase 3,7 activity level was measured using a calorimetry-based kit (Kiazist Life Sciences (KCAS37), Iran) according to manufacturer instructions. Briefly, 4T1 cells were seeded in 6 well plates (2 × 105 per well) and maintained in an incubator for 24 h. After that, the cells were treated with 2.5 mg ml−1 of NPs and again incubated for 24 h. Then, the cells were harvested and counted and 500 μl of caspase lysis buffer was added to 5 × 105 cells. Cell lysate was obtained by incubation of cell-containing buffer at 4 °C for 20 min. Subsequently, the cell lysate was centrifuged at 12
000 rpm for 15 min at 4 °C and stored at −80 for analysis. 50 μl of each sample, 50 μl of caspase buffer, 0.5 μl of DTT, and 5 μl of caspase substrate were added to each well of a 96-well plate. The plate was incubated for 5 h at 37 °C and then its absorbance was read at 405 nm using a plate reader. Finally, the Caspase 3,7 activity was measured according to the absorbance of kit recommended standard solutions.
2.7.6. Scratch wound healing assay.
The scratch wound healing assay was performed to examine the effect of NPs on the migration ability of 4T1 cells. Briefly, 2 × 105 cells were seeded into each well of 6-well plates. When plates reach 80–90% confluency, a scratch was created using a sterile pipette tip (10 μL). After washing the plates with PBS, culture media containing 2% FBS were added to each well. Then, photographs were obtained from the scratch area and subsequently the cells were treated with 2.5 mg ml−1 of NPs. After 24 h, photographs of the scratch areas were taken again.
3. Results and discussion
3.1. Preparation and characterization of copper–cobalt metallic peroxides
Based on the two crucial factors in the selection of metals for bimetallic peroxides, which have been mentioned earlier in the Introduction part, it was expected that cobalt
, cerium
and iron
were good candidates for CDT applications. In this work, copper–cobalt peroxide was chosen for a comprehensive study based on the higher positive value of ΔErxn and two other metals were prepared at the optimum ratio of CuCo peroxide NPs for comparison. CCp-NPs with different Cu to Co ratios (CuxCo1−xp, x = 0.8, 0.7, 0.6, 0.5, 0.4 and 0.3, henceforth referred to as 8CCp, 7CCp, 6CCp, 5CCp, 4CCp and 3CCp) were prepared varying the Cu to Co ratios (Fig. 1). This approach allows for the exploration of the effects of different compositions on the catalytic activity of the NPs, providing valuable insights for potential biomedical applications. The morphology and size of 7CCp- and 6CCp-NPs were investigated using transmittance electron microscopy and dynamic light scattering (Fig. 2a–d). CCp NPs were found to be well-dispersed in water (Fig. 2e), with an average hydrodynamic diameter of 8 nm. This is advantageous for potential biomedical applications, as it may allow the accumulation of nanoparticles in tumours or other diseased tissues, which tend to have a more permeable vasculature and show a higher retention of nanoparticles (EPR effect). Moreover, the zeta potential values of the 7CCp and 6CCp NPs were found to be similar (Fig. 2f), regardless of their different Cu-to-Co ratios. This observation is important to understand, as it indicates that their composition-dependent catalytic activity (vide infra) is not solely due to differences in their physical characteristics, yet it is likely that the differences in composition affect the electronic and chemical properties of the NPs, which in turn influence their catalytic activity. It should be noted that since DLS measures the hydrodynamic diameter, the mean hydrodynamic radius obtained by DLS (Fig. 2c and d) was slightly higher than that determined by TEM (Fig. 2a and b). Energy-dispersive spectroscopy (EDS) elemental mapping revealed that the Cu and Co elements coexist throughout the CCp NPs and are uniformly distributed (Fig. 3a and c). On the other hand, the atomic weight percent (% A) values obtained from EDS studies are consistent with the expected composition of 7CCp and 6CCp-NPs. Furthermore, X-ray diffraction analysis, as shown in Fig. 3f, confirms the amorphous nature of the synthesized copper–cobalt peroxide using a PVP-stabilized reducing agent. This finding is consistent with those in previous literature reports.21,40 The FT-IR analysis of the 7CCp NPs showed (Fig. S1†) a strong absorption peak at 1674 cm−1, which is characteristic of the C
O group in polyvinylpyrrolidone (PVP). In addition, the stretching vibration peaks of C–N and CH2 were observed at 1250 and 1427 cm−1, respectively. These results suggest that PVP is present on the surface of the NPs and is likely responsible for stabilizing the particles and preventing agglomeration. Raman spectroscopy (Fig. S2a†) was utilized to confirm the presence of O–O bonds in 7CCp NPs. The peak observed at 850 cm−1 is attributed to the O–O stretching vibration, which is not present in the Raman spectra of copper oxide40 and cobalt oxide (Fig. S2b†).
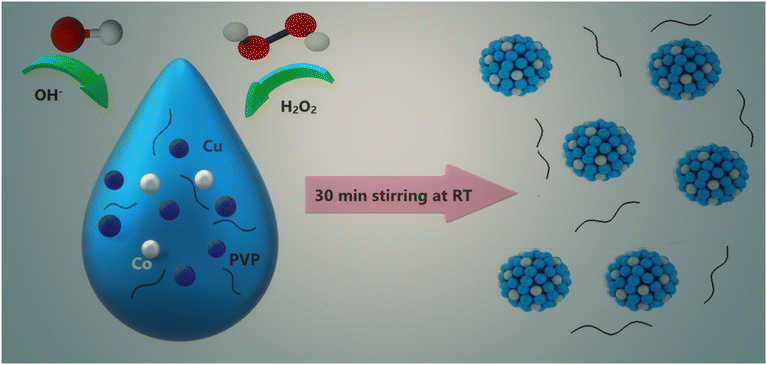 |
| Fig. 1 Schematic representation of CCp-NP synthesis. | |
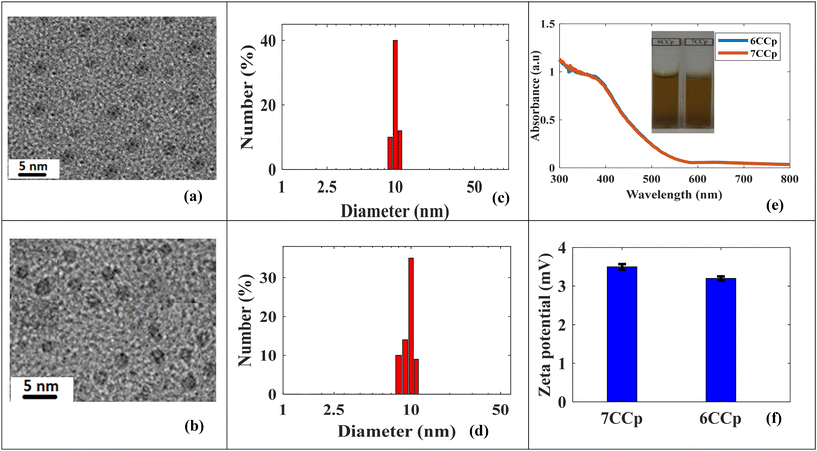 |
| Fig. 2 (a and b) TEM images of 7CCp and 6CCp-NPs. (c and d) Hydrodynamic diameter of 7CCp and 6CCp-NPs in water. (e) UV-vis spectra of 7CCp and 6CCp-NPs. (f) Zeta-potential data analysis of 7CCp and 6CCp-NPs. | |
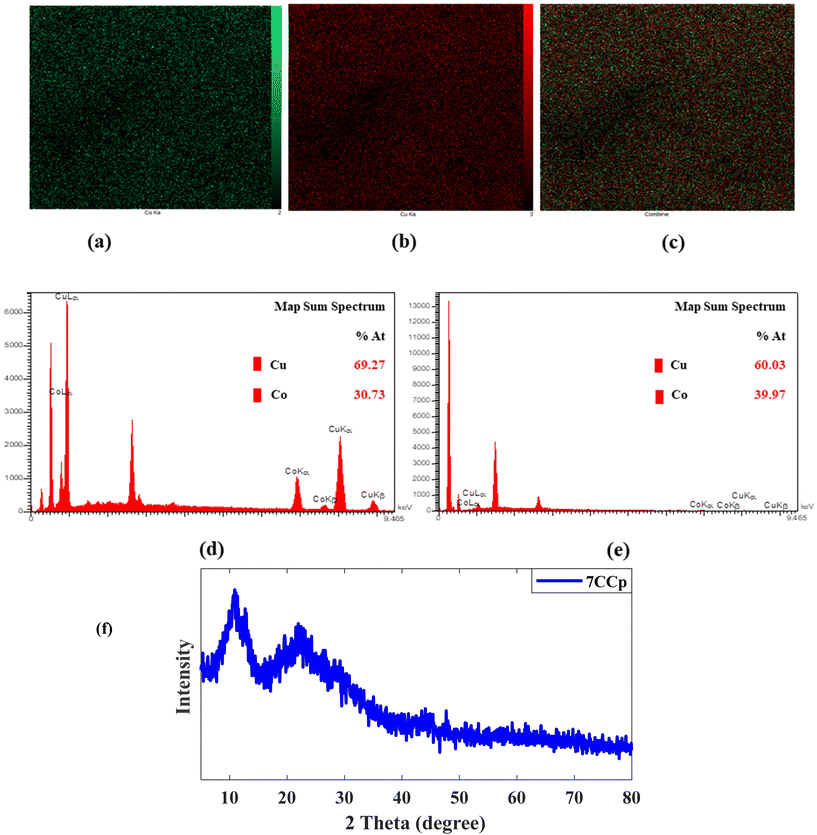 |
| Fig. 3 EDS elemental mapping analysis of (a) Cu and (b) Co elements and (c) their combination in as-prepared 7CCp-NPs. EDS spectra of (d) 7CCp NPs (69.27 at% Cu and 30.73 at% Co), (e) 6CCp NPs (60.03 at% Cu and 39.97 at% Co) and (f) XRD patterns of 7CCp-NPs. | |
In addition to Raman analysis, the presence of peroxo groups was confirmed using the potassium permanganate-based colorimetric method (the peroxo group can convert permanganate (MnO4−) to colorless Mn2+) and XPS analysis. Fig. 4a and b demonstrate that the purple color of permanganate (MnO4−) in an acidic solution vanished after the addition of 8CCp, 7CCP, 6CCp and 5CCp NPs. Furthermore, by increasing the ratio of Co/Cu in the CCp NP compositions, the color changes of permanganate become slower and finally there is no color change in purely cobalt-based NPs (Fig. 4a). Moreover, this color disappearance is very fast for 7CCp compared to other CCp NPs. The corresponding videos for 7CCp and 3CCp were recorded and are shown in Movies S1 and S2.† Furthermore, X-ray photoelectron spectroscopy (XPS) analysis of oxygen, O, for 7CCp nanoparticles revealed two distinct O 1s peaks at 530.5 eV and 532.6 eV (Fig. 6a). These peaks can be attributed to carbonyl (C
O) and peroxo (O–O) bonding environments, respectively. This observation suggests the presence of both polyvinylpyrrolidone (PVP) and peroxo groups on the surface of the CCp-NPs. BET surface area, BJH pore volume and cumulative pore volume analyses from the adsorption branch of 7CCp (Fig. S3†) confirmed the non-porous nature of the synthesized bimetallic peroxide nanoparticles.
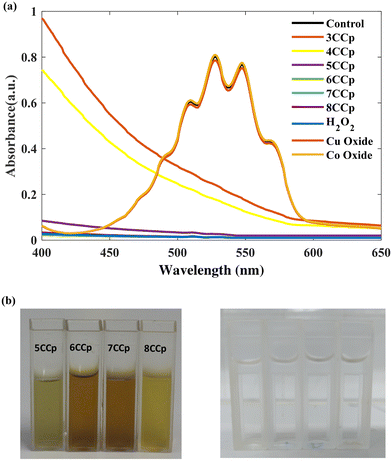 |
| Fig. 4 UV-vis absorption spectra (a) and photographs (b) of the permanganate-based colorimetric assay of various CCp-NP compositions. | |
To explore the possible cause of this observation, the theoretical analysis of structural changes of peroxo groups from Cup-NPs to NPs that are exclusively composed of cobalt has been conducted. To simulate our experimental nanoparticles, Density Functional Theory (DFT) calculations were utilized to optimize the structures and electronic characteristics of selected metal peroxide clusters as representative compounds of the actual systems studied. The Cu32O64 cluster in Fig. 5a was optimized as a reference for Cup-NPs, Cu22Co10O64 (Fig. 5b) as a model for NPs with a Cu/Co ratio of 70
:
30, Cu10Co22O64 (Fig. 5c) for a Cu/Co ratio of 30
:
70, and Co32O64 (Fig. 5d) as a reference for Cop-NPs. The geometry optimized structures of two Cu/Co peroxide clusters shown in Fig. 5b and c reveal some interesting trends. The cubic structure of the Cu32O64 cluster transforms into a more semispherical structure in the Cu/Co clusters, while the Co atoms are distributed both on the surface and in the core (Fig. 5b). Gao et al.58 showed theoretically and experimentally that Co single-atom catalysts possess an optimal d-band center and can function as highly active and selective catalysts for H2O2 synthesis compared to Cu and other transition metals. This suggests that doping Co into Cu32O64 may increase the ability to self-supply H2O2 after exposure to an acidic environment, where the produced O2 molecules absorb on the top sites of Co atoms according to Gao et al.58 Although comparing the number of peroxide bonds (Fig. 5a and b) reveals that incorporating 30% Co atoms in Cu22Co10O64 decreases the number of O–O bonds compared to Cu32O64, Co single-atom catalytic activity apparently increases according to experimental observation. This indicates that while doping Co may enhance H2O2 synthesis,58 the optimal Cu/Co ratio also depends on the number of O–O bonds in the cluster. At higher Co doping levels, these peroxy bonds mostly convert to metal–O–metal bonds, which is completely evident from the optimized structures of Cu10Co22O64 and Co32O64 (Fig. 5c and d). This theoretical evidence is in agreement with the Raman shift spectra of cobalt oxide NPs (Fig. S2†). To summarize, the incorporation of Co appears to enhance the ability to synthesize H2O2. However, there exists an optimal Cu/Co ratio that balances this effect with the maintenance of adequate O–O bonds within the cluster, which is crucial for effective self-supply of H2O2. Additionally, we studied the adsorption of one proton on different sites of the most stable configuration of Cu32O64 and Cu22Co10O64 clusters and let the structures relax again to obtain the most stable configuration to estimate the stability of these clusters under acidic conditions. In the case of Cu22Co10O64, the proton prefers to interact with oxygen in the Cu–O–O–Co site while the Cu–O–Cu or Cu–O–Co sites did not energetically converge in this study and we could not obtain a relaxed configuration (Fig. 5e and f).
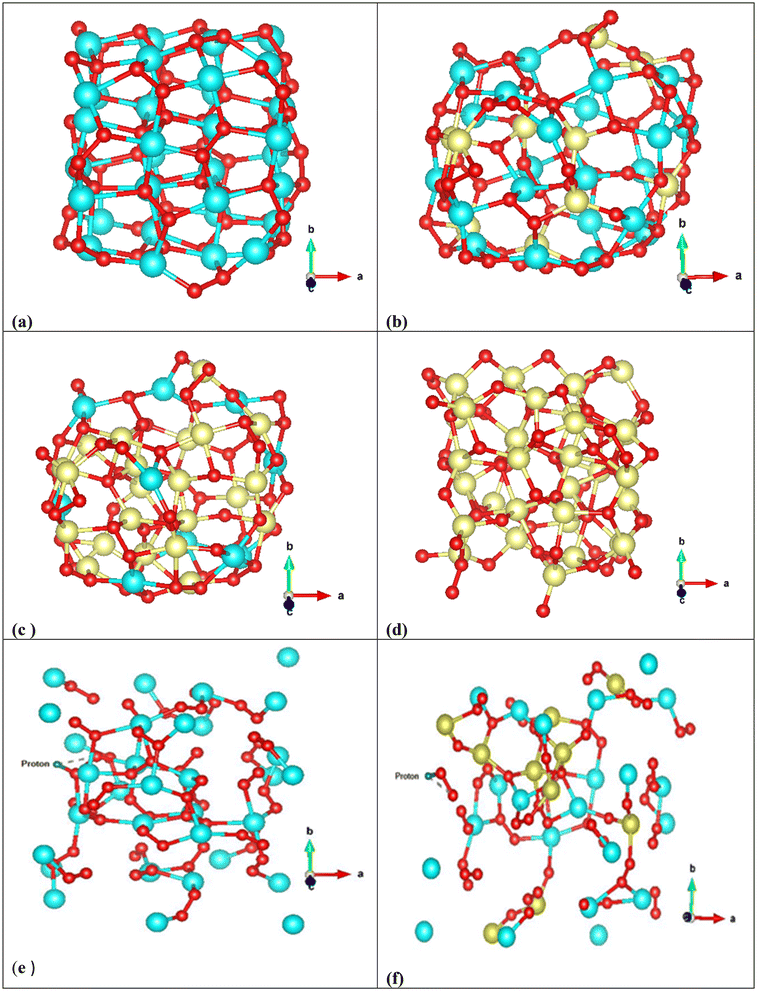 |
| Fig. 5 Optimized geometry of (a) Cu32O64, (b) Cu22Co10O64, (c) Cu10Co22O64 and (d) Co32O64, (e) adsorption of a proton on Cu32O64 and (f) adsorption of a proton on Cu22Co10O64. Cu in blue, Co in yellow and O in red. | |
After proton adsorption, the geometrical parameters of the clusters changed significantly such as the average Cu–O–Cu, Cu–Co, Cu–O–O–Co and Co–O bond distances, which are apparently evident from the final geometries denoting an interaction between the proton and the surface oxygen atoms. However, this interaction leads to weakened stability of clusters, and according to the final configurations, the surface atoms have shifted from their binding states. Interestingly, in Cu22Co10O64, H–O–O, O–O and Cu–O have been distanced from their original locations and seem to be completely separated. These geometrical changes can be evidence of instability of this cluster under acidic conditions, which leads to self-supply of H2O2 after producing free H–O–O or metal–O–O (Fig. 5e and f). From the adsorption energies of both clusters (Eads/total(Cu32O64H+) = +108.24 eV and Eads/tot(Cu22Co10O64H+) = +299.13 eV), it is clear that acidic conditions considerably destabilized both configurations; however, this effect is more important in Cu22Co10O64, in which the obtained structure is +299.13 eV less stable than the isolated cluster and the proton. According to the found results of geometry optimization, Co doping almost increases the total charge of oxygen in Cu–O1–O2–Co in the metal–O–O–metal bonds, and the average charge value of O2 is approximately 4.95, while it is 4.92 in Cu32O64. However, in proton added Cu22Co10O64, the total charge of O1 (proton–O1–O2) reduces to 4.90 from 4.94, while that of O2 decreases from 4.95 to 4.91, and hydrogen gains 0.56 during charge transfer from the O–O bond, and these charges are 4.90 and 4.93 in Cu32O64, respectively. It can be concluded that doping Co can impact the electronic structure of oxygen, leading to an increase in its charge density and making it more reactive (nucleophile) under acidic conditions. This probably helps to synthesize hydrogen peroxide more easily.
Moreover, one of the important chemical components in a bimetallic cycling Fenton-like reaction based on copper is the presence and the amount of reduced copper (Cu+).7,40,59 Cu+ in a lower energy state is significant for two processes: the reducing power of Cu+ accelerates the transformation of Co3+ to Co2+, Ce4+ to Ce3+ and Fe3+ to Fe2+ rxn (1)–(3), and it facilitates the generation of ˙OH through a Fenton-like reaction rxn (4). According to Li et al.,59 this step can be considered as one of the possible pathways to decompose H2O2 to ˙OH.
| Cu+ + Co3+ → Cu2+ + Co2+ ΔE° ≅ 1.7 V | (1) |
| Cu+ + Ce4+ → Cu2+ + Ce3+ ΔE° ≅ 1.29 V | (2) |
| Cu+ + Fe3+ → Cu2+ + Fe2+ ΔE° ≅ 0.62 V | (3) |
| Cu+ + H2O2 → Cu2+ + ˙OH + OH− | (4) |
The superior catalytic performance of 7CCp, in comparison with its 7CFp and 7CCep counterparts, can be contributed to the enhancement of the Co3+/Co2+ cycling reaction facilitated by the Cu2+/Cu+ redox pair (ΔE° ≅ 1.7 V), as demonstrated in rxn (1), which is thermodynamically spontaneous.
Photoelectron spectroscopy (XPS, Fig. 6) analysis of 7CCp was conducted to determine the surface composition and valence electron state of copper in 7CCp-NPs. As shown in Fig. 6a, two distinct peaks corresponding to 2p1/2 and 2p3/2 along with a weak satellite peak are characteristic of 7CCp-NPs. The obtained binding energies for 2p3/2 and 2p1/2 are 952.1 and 931.9 eV, respectively, which shift to lower values compared to copper (Cup) and copper-ferrite peroxidase.40 Besides, to calculate the Cu+/Cu2+ ratio in 7CCp, we deconvoluted (Fig. 6b) the Cu 2p3/2 and 2p1/2 XPS peaks. It is noteworthy that the proportion of Cu+ to Cu2+ in 7CCp was 2.34, which was greater than that observed in the recently produced bimetallic peroxides 7CFp and Cup under similar synthesis conditions.40 This suggests that 7CCp contains a higher amount of Cu+ than both Cup and copper-ferrite peroxide (7CFp). This higher reduction tendency in bimetallic structures such as CCp-NPs can be largely attributed to the presence of oxygen vacancies, known as OV, in their structures.52,60 It is widely accepted that OV can enhance the Fenton reaction52,59,61,62via increasing the Cu2+/Cu+ and Co3+/Co2+ cycles (rxn (1)) and elongating the length of the O–O bond in H2O2, thereby promoting the activation of H2O2 into ˙OH. In accordance with previous studies,59,63,64 the reduction of both copper and cobalt (Cu2+/Co3+ to Cu+/Co2+) can be mainly affected by an increment of the oxygen vacancies in the structure. Several theoretical studies, based on DFT calculations, reveal that there is a correlation between oxygen vacancy variations and band gap changes60,63 and show that a larger number of oxygen vacancies corresponds to a smaller optical bond gap. Fig. 7a and b show the UV-vis spectra and Tauc plot for our three Cu based metal peroxides. Tracing the figures to intercept (hν = 0) indicated that the direct band gap was estimated to be 2.55 eV, 2.81 eV, and 3.16 eV for 7CCp, 7CCep and 7CFp, respectively. This enhancement facilitates the charge transfer of redox pairs and extends the length of the O–O bond in H2O2, thereby promoting the activation of H2O2 into ˙OH.
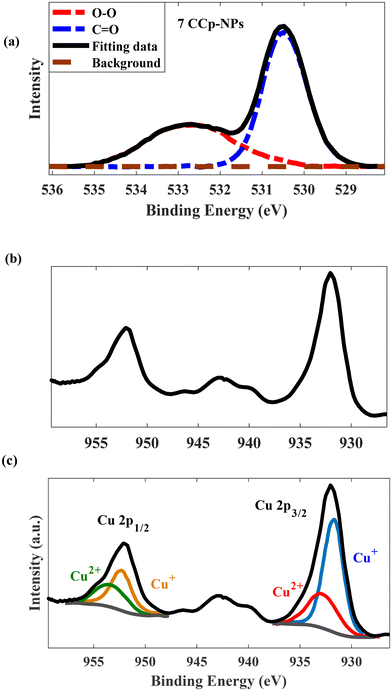 |
| Fig. 6 High-resolution XPS spectra of (a) O 1s and (b and c) Cu 2p for 7CCp-NPs. | |
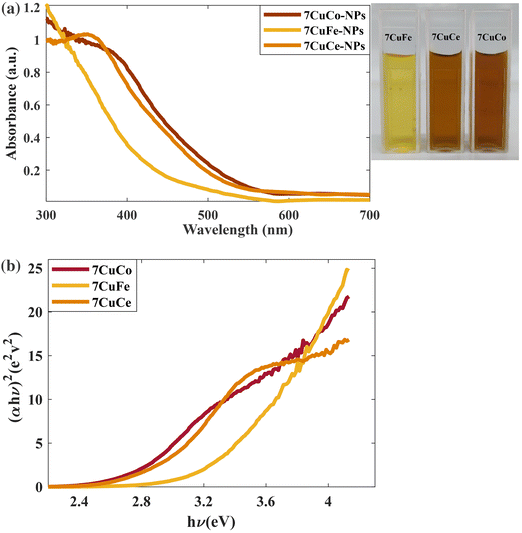 |
| Fig. 7 (a) UV-vis spectra of 7CCp, 7CFp and 7CCep-NPs. (b) Determination of the direct optical bandgap using Tauc plots of 7CCp, 7CFp and 7CCep-NPs. | |
Another crucial factor that should be investigated in the synthesis of CCp-NPs is the OH− concentration. Therefore, we performed the synthesis process with different OH−/Cu2+ mole ratios. It is not surprising that, without OH− addition, no obvious color change was seen upon mixing metal mixtures with hydrogen peroxide. This implies that the inclusion of OH− is required to obtain CCp-NPs. As the molar ratio of OH− to Cu2+ increased (2
:
1), more composites of copper/cobalt were synthesized (Fig. S4†). Nevertheless, a further increase in the molar ratio (3
:
1) resulted in unexpected precipitation and a larger size of CCp-NPs. According to the above results, the concentration of O22−, produced from deprotonation of H2O2, to coordinate with metals depends on the NaOH concentration.
3.2. Fenton-like reactions using copper-based bimetallic peroxides
To evaluate the enzyme mimetic activity of our novel bimetallic peroxide (7CCp-NPs) through Fenton-like reactions, the typical peroxidase substrate was carried out using 3,3′,5,5′-tetramethylbenzidine (TMB). This colorimetric test is based on the principle that a highly reactive species such as ˙OH can oxidize the colorless TMB probe to produce a blue derivative with an absorbance at ∼650 nm, which can be monitored using UV-vis.21,32 As shown in Fig. S5 and S6† neither Cu2+ nor H2O2 was able to cause any change after 60 min in the absorbance of TMB. In contrast, the combination of Cu2+ and H2O2 has the ability to oxidize TMB and change its colour under acidic conditions (pH 5.4) (Fig. 8a), and 7CCp-NPs perform the reaction more efficiently (at pH 5.4, TMB oxidation absorbance increased approximately 2 fold compared with the Cu2+ and H2O2 combination). On the other hand, as shown in Fig. 8a, the catalytic activity of Cu–Co bimetallic peroxidase NPs stops under neutral conditions (pH ∼ 7.4), which indicates that the activation of 7CCp depends on the pH. To complement the colorimetric analysis using 3,3′,5,5′-tetramethylbenzidine dihydrochloride (TMB) as an indicator, electron paramagnetic resonance (EPR) spectroscopy was employed to directly detect hydroxyl radicals (˙OH). By utilizing 5,5-dimethyl-1-pyrroline N-oxide (DMPO) as a spin trap, we were able to capture and identify ˙OH. In alignment with the spectrophotometric findings, the EPR spectrum of 7CCp at pH = 6.4 exhibited a characteristic 1
:
2
:
2
:
1 quartet signal (Fig. 8b), confirming the generation of ˙OH.1
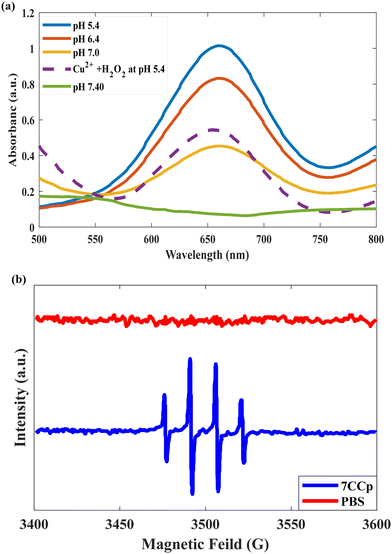 |
| Fig. 8 (a) Colorimetric detection of ˙OH generated by 7CCp-NPs at different pH values based on TMB assay. (b) EPR spectra of 7CCp-NPs with 5,5-dimethyl-1-pyrroline N-oxide as the ˙OH trap at pH 6.4. | |
In addition, we measured the release of Cu and Co from 7CCp-NPs in different pH media. According to Fig. S7,† over 96% of Cu and Co metals are released as ions at pH 5.4, while the release is insignificant at pH 7.4. As the typical pH range in the tumor microenvironment is around 6.5–7.0, the activation of CCp-NPs via ultrasensitive pH stimuli provides a promising alternative to increase the effectiveness of CDT. In addition, a comparison of the catalytic activity between 7CCp and 6CCp NPs for oxidizing the TMB probe was conducted (Fig. S8†). The results confirmed the superior performance of 7CCp NPs at various pH values. These results suggest that the 7CCp NPs are highly responsive to changes in pH and readily release Cu and Co ions. It is hypothesized that in the Cu–Co heterogeneous system, Cu and Co ions are liberated upon exposure to an acidic environment, which enables the formation of a catalytic loop. This loop facilitates efficient interconversion between metal ions with varying oxidation states, leading to a synergistic Fenton-like reaction.21,32,39 Another important parameter that should be considered for the synergistic catalytic performance of CCp NPs in the Fenton reaction is the availability of lower oxidation state ions (Cu+ and Co2+). In other words, CCp NPs that are capable of releasing larger amounts of Co2+ and Cu+ ions have been shown to demonstrate a greater Fenton catalytic efficiency than Cup nanoparticles (eqn (1)). This is due to the fact that Co2+ and Cu+ ions are the active species responsible for the Fenton reaction, which produces highly reactive ˙OH. We exposed CCp NPs with different Cu/Co compositions to various pH conditions and the Cu+/Cu2+ ratio was measured (Fig. S9†). As we can see, the highest ratio of reduced Cu was observed in 7CCp NPs at pH 5.4, with values of 3.7 times higher than those of Cup. Interestingly, the activity of the nanocomposite decreases as the amount of cobalt in the composition increases (4CCp and 3CCp), which is attributed to the formation of stable oxide structures. The same trends (not shown) have been seen for reduced Co states between our bimetallic composites; however, it should be mentioned that we did not have any stable peroxide structure for cobalt (Fig. S2b†). Besides, by decomposing bimetallic peroxide in an acidic environment (such as that in the TME) and generating H2O2in situ, H2O2 self-supply can be achieved in Fenton-like reactions. In order to compare the efficiency of 7CCp-NPs in producing free ˙OH during Fenton reactions, 7CFp and 7CCep at two different pH values (5.4 and 7.0) were compared. The UV-vis absorption spectra (Fig. 9) of the TMB-based colorimetric assay clearly confirmed the superiority of 7CCp compared to 7CFp and 7CCep NPs. These observations are in agreement with the Tauc curve, which predicts the OV generation in 7CCp.
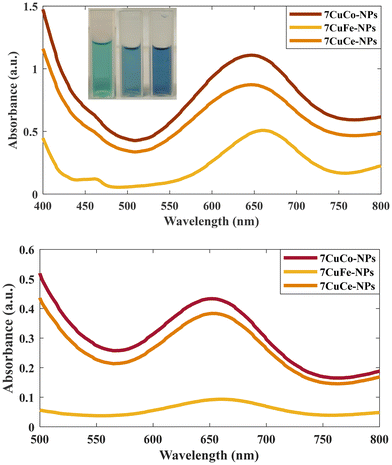 |
| Fig. 9 Comparison of the UV-vis absorption spectra of the TMB colorimetric assay of 7CCp, 7CFp and 7CCep-NPs at (a) pH 5.4 and (b) pH 7.0. | |
Therefore, theoretical and experimental evidence suggests that incorporating cobalt (Co) into copper-based nanoparticles (Cu-NPs) enhances their ability to synthesize hydrogen peroxide (H2O2). However, there exists an optimal Cu/Co ratio (70
:
30) that balances this effect while maintaining adequate O–O bonds within the cluster. This delicate balance is crucial for efficient self-supply of H2O2 in acidic environments. Additionally, Co doping impacts the electronic structure of oxygen in peroxy bonds, likely increasing oxygen's charge density and rendering it more reactive (nucleophilic) under acidic conditions. This enhanced hydrogen peroxide production plays a key role in activating the Fenton reaction, leading to increased OH radical generation.65,66
Finally, in order to confirm the H2O2 generation by CCp-NPs under different pH conditions, Ti(SO4)2-based colorimetric detection has been introduced. In this way, a solution of 7CCp (0.625 mg ml−1) was prepared with phosphate-buffered saline (PBS) at various pH values (5.4, 6.5, 7.0, and 7.4). To each PBS solution, 0.1 ml of a titanium sulfate (Ti(SO4)2)–sulfuric acid (H2SO4) solution (containing 1.33 ml of 24% Ti(SO4)2 and 8.33 ml of H2SO4 in 50 ml of deionized water) was added. The concentration of hydrogen peroxide (H2O2) was monitored over time using UV-Vis spectrophotometry at 405 nm, with measurements taken at 10-second intervals. A calibration curve was used to quantify the H2O2 concentration. Our data revealed (Fig. S10†) a strong pH-dependent trend in the catalytic activity of 7CCp towards hydrogen peroxide production. The highest H2O2 yields were observed at acidic pH levels, while it was negligible at pH 7.4. This correlation between pH and H2O2 generation is consistent with our previous findings on the formation of hydroxyl radicals and the oxidation of TMB under acidic conditions.
3.3. Cell culture studies
The MTT assay revealed contrasting cytotoxicity profiles of the nanoparticles (NPs) in human foreskin fibroblast (HFF) and breast cancer (4T1) cells (Fig. 10a). NPs exhibited minimal toxicity (below 20%) towards HFF cells even at doses as high as 2.5 mg ml−1. According to previous studies, percentages of cell viability above 80% can be considered non-cytotoxic.67–69 In contrast, significant cytotoxicity was observed in 4T1 cells at doses as low as 0.625 mg ml−1 and above. Notably, CCp displayed significantly higher toxicity (p < 0.001) compared to other NPs in 4T1 cells at 2.5 mg ml−1, while no significant differences (p > 0.05) were observed among the NPs in HFF cells at the same dose. While HFF cell toxicity increased at higher doses (5 and 10 mg ml−1, exceeding 40%), it remained significantly lower compared to that of 4T1 cells. Consequently, 2.5 mg ml−1 was chosen as the optimal dose for further studies. Fig. 10b shows the fluorescence microscopy images of cells treated with 2.5 mg ml−1 of NPs and stained with PI/AO. In the merged images, green cells are alive and red, orange and yellow cells are dead. As the figures show, the relative count of live/dead cells in the HFF cells is not considerably different. In contrast, in 4T1 cells, dead cells are much more evident when we compare the NPs’ treated groups with the control group. Also, dead cells in CCp treated cells are considerably higher than those in cells treated with the other two types of nanoparticles. It is hypothesized that the higher toxicity of NPs against 4T1 cells compared to HFF cells is mainly due to the higher growth rate of 4T1 cells. Cancerous 4T1 cells grow faster and produce more acidic metabolites and as a result lower the pH of the culture medium. On the other hand, it is known that an acidic tumor microenvironment facilitates the Fenton reaction, a key component of CDT's tumor-killing mechanism,23,24 which effectively targets and eliminates tumor cells, while minimizing damage to healthy tissues.25–27 Colony formation assay findings (Fig. 11) showed that 7CCep and 7CCp strongly inhibit colony forming cells compared with control (p < 0.001) and Cup (p < 0.01) groups, so almost no colonies were observed in the group treated with CCp. A clonogenic assay is the gold standard for the evaluation of cancer stem cell (CSC) stemness by measuring their ability to form colonies and determining the effectiveness of chemo/radio-therapies.70 Also, clonogenic activity is considered as an indicator of undifferentiated cancer stem cells.71 Therefore, CCp has the ability to almost completely inhibit colony formation of 4T1 cells at 2.5 mg ml−1 concentration.
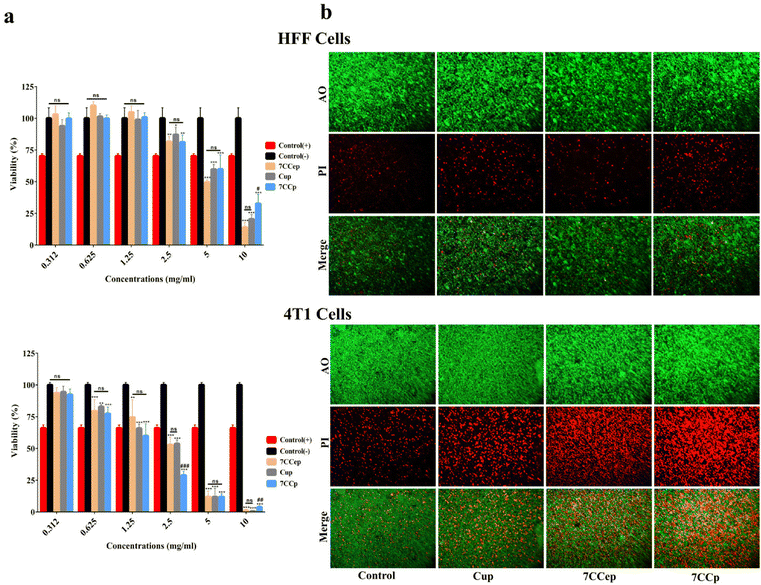 |
| Fig. 10 MTT and fluorescence cell proliferation assays. (a) MTT assay of NPs in HFF and 4T1 cell lines. ns: not significant. *, ** and *** indicate differences with the control group at p < 0.05, p < 0.01 and p < 0.001, respectively. #, ## and ### indicate differences with the Cup group at p < 0.05, p < 0.01 and p < 0.001, respectively. (b) Fluorescence cell proliferation assay of NPs in HFF and 4T1 cell lines. | |
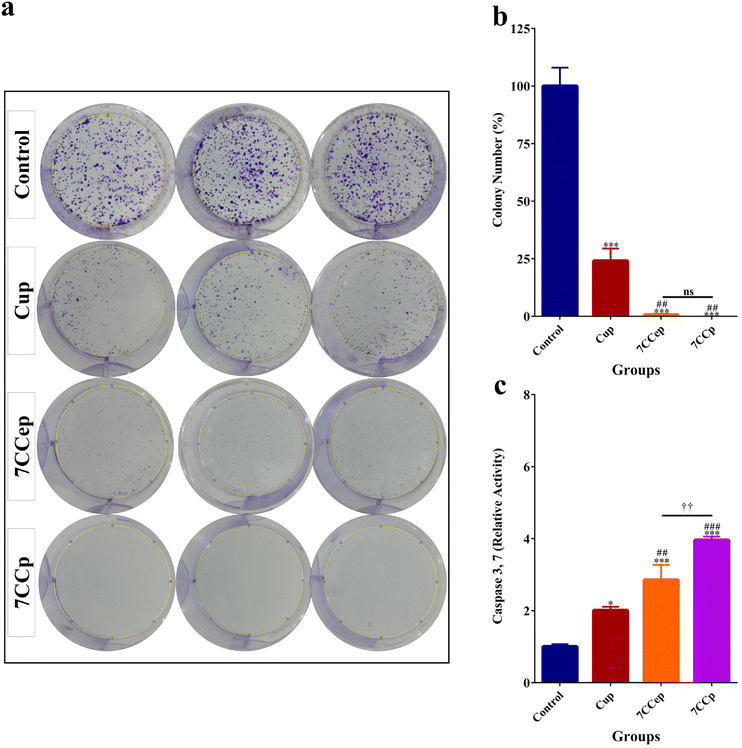 |
| Fig. 11 Colony formation assay and Caspase 3,7 assay. (a) Formed colonies in the control group and groups treated with NPs. (b) Comparison of counted colonies in the control group and groups treated with NPs. (c) Comparison of Caspase 3,7 activity in NP treated groups with the control group. * indicates a difference with the control group at p < 0.05. ** indicates a difference with the control group at p < 0.001. ## indicates a difference with the Cup group at p < 0.01. ### indicates a difference with the Cup group at p < 0.001. †† indicates a difference between 7CCp and 7CCep groups at p < 0.01. | |
The caspase activity assay (Fig. 11c) showed that 7CCp treated cells have a significantly higher level of Caspase 3,7 activity in comparison with other groups (p < 0.01). Also, the Caspase 3,7 activity level in the 7CCep group was higher than those in the Cup (p < 0.01) and control (p < 0.001) groups. A significant difference was observed between the Cup and control groups (p < 0.05). Caspase 3/7 activity is an indicator of apoptosis, involved in both the extrinsic and intrinsic apoptosis pathways.72–74 This means that the treatment of 4T1 cells with 7CCp nanoparticles induces a higher rate of apoptosis than other nanoparticles. In fact, Fenton reactions produce free radicals, including hydroxyl, and these free radicals cause various types of cell deaths, including apoptosis.75–77 Cuproptosis, a recently identified form of regulated cell death driven by copper ion accumulation, may also be triggered by the copper ions released from the bimetallic nanoparticles, although it was not explored in our study.78
In general, the robust anticancer efficacy of CCp in this study can be attributed to the excellent synergistic effects of CuCo bimetallic peroxide nanoparticles that enable more efficient catalytic Fenton-like reactions than Cu alone or other bimetallic peroxide nanoparticles such as CuFe and CuCe. Copper–cobalt peroxides enhance hydroxyl radical formation over a highly efficient catalytic loop, resulting in considerable anti-cancer activity. As seen in Fig. 12, the scratch gap reduced considerably in untreated cells (control) after 24 h. However, no observable changes occurred in the scratch gap of Cup-treated cells after 24 h. On the other hand, a significant increase in the scratch gap is evident following 24 h treatment with 7CCep or 7CCp nanoparticles, especially 7CCp-NPs. Therefore, 7CCp nanoparticles also have great ability to inhibit the migration of cancer cells. Invasion and migration are two important properties of metastatic cancer cells.79 Metastasis is a main cause of cancer deaths80 and a major challenge in cancer treatment.79
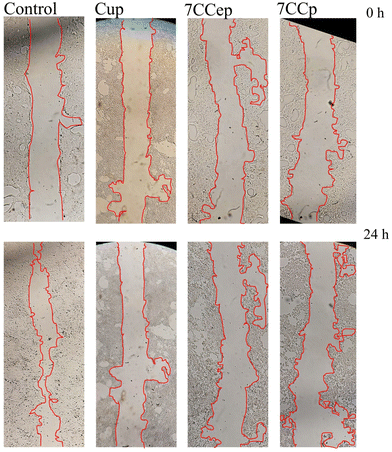 |
| Fig. 12 Scratch wound healing assay. The scratch area was photographed at 0 h and 24 h after treatment with NPs. | |
4. Conclusion
In summary, three types of copper-based metallic peroxide nanoparticles namely (CCp-, CFp- and CCep-NPs) as highly potent nanocatalytic Fenton-like systems were synthesized and their catalytic activity was studied and compared. The prepared bimetallic peroxides were shown to have a high pH-activated, pH responsive release of Cu and Co/Fe/Ce ions, a synergistic catalytic loop, and H2O2 self-supply-mediated Fenton chemistry. This improved the limited efficiency of the classical Fenton reaction, which was insensitive to the relatively high pH of tumor sites and the inadequate levels of H2O2 within tumors. The synthesis strategy for CCp is remarkably simple, involving a straightforward one-pot reaction. This streamlined approach makes CCp more amenable to large-scale production and potential clinical translation. Moreover, higher oxygen vacancies in Cu–Co tend to form more easily, readily transfer their excess electrons to Co3+/Cu2+, and are able to more effectively reduce Cu/Co species. All these factors contribute to the generally stronger reduction tendency observed in the Cu–Co system compared to Cu–Fe and Cu–Ce. The DFT studies showed the importance of finding the optimal Cu/Co ratio to achieve a balance between enhanced H2O2 syntheses and maintaining the stability of O–O bonds for effective self-supply under acidic conditions. Doping Cu32O64 clusters with optimal Co may enhance their ability to self-generate H2O2 upon exposure to acidic environments. This enhancement likely arises from an increase in the electron density of oxygen atoms within the O–O bonds. Cell culture studies revealed that CCp-NPs can considerably inhibit breast cancer cell proliferation with minimal effects on non-cancerous cells when used in optimal concentration. Also, it could significantly suppress cell migration and colony formation in cancer cells.
Data availability
Most data supporting this article have been included as part of the main text and the ESI.† Further data (Caspase 3,7 activity assay, raw MTT assay, and all optimized structures) are available from the corresponding authors upon reasonable request.
Conflicts of interest
The authors declare no competing interests.
Acknowledgements
RMM thanks the project PID2021-126304OB-C41 funded by MCIN/AEI/10.13039/501100011033/ and by the European Regional Development Fund – A way of doing Europe. RMM also thanks the Generalitat Valenciana (CIPROM/2021/007) for support. HM and MF would like to thank the Deputy of Research and Technology of Bushehr University of Medical Sciences, Iran, for supporting this research project. SK wishes to acknowledge the financial support of the Persian Gulf University Research Council in conducting the present study.
References
- W. Zuo, N. Liu, Z. Chang, J. Liu, Q. Jin, L. Chen and X. Zhu, Chem. Eng. J., 2022, 442, 136125 CrossRef CAS.
- R. Lin, W. Yu, X. Chen and H. Gao, Adv. Healthcare Mater., 2021, 10, 2001212 CrossRef CAS.
- P. Sun, F. Qu, C. Zhang, P. Cheng, X. Li, Q. Shen, D. Li and Q. Fan, Adv. Sci., 2022, 9, 2204718 CrossRef CAS.
- C. Jia, Y. Guo and F. G. Wu, Small, 2022, 18, 2103868 CrossRef CAS.
- X. Chu, L. Zhang, Y. Li, Y. He, Y. Zhang and C. Du, Small, 2023, 19, 2205414 CrossRef CAS.
- H. Chen, G. D. Wang, Y.-J. Chuang, Z. Zhen, X. Chen, P. Biddinger, Z. Hao, F. Liu, B. Shen and Z. Pan, Nano Lett., 2015, 15, 2249–2256 CrossRef CAS PubMed.
- B. Ma, S. Wang, F. Liu, S. Zhang, J. Duan, Z. Li, Y. Kong, Y. Sang, H. Liu and W. Bu, J. Am. Chem. Soc., 2018, 141, 849–857 CrossRef PubMed.
- G. Zhu, P. Zheng, M. Wang, W. Chen and C. Li, Inorg. Chem. Front., 2022, 9, 1006–1015 RSC.
- Y. Deng, M. Guo, L. Zhou, Y. Huang, S. Srivastava, A. Kumar and J.-Q. Liu, Biomater. Sci., 2024, 12, 3725–3744 RSC.
- M. Li, Z. Zhang, Y. Yu, H. Yuan, A. Nezamzadeh-Ejhieh, J. Liu, Y. Pan and Q. Lan, Mater. Adv., 2023, 4, 5050–5093 RSC.
- M. Shu, J. Tang, L. Chen, Q. Zeng, C. Li, S. Xiao, Z. Jiang and J. Liu, Biomaterials, 2021, 268, 120574 CrossRef CAS.
- J. Qi, G. Jiang, Y. Wan, J. Liu and F. Pi, Chem. Eng. J., 2023, 466, 142960 CrossRef CAS.
- Y. Liu, X. Wang, H. Chen, T. Wu, Y. Cao and Z. Liu, ACS Appl. Mater. Interfaces, 2023, 15, 8937–8945 CrossRef CAS PubMed.
- Q. Li, F. Wang, L. Shi, Q. Tang, B. Li, X. Wang and Y. Jin, ACS Appl. Mater. Interfaces, 2022, 14, 37280–37290 CrossRef CAS PubMed.
- X. Nie, L. Xia, H.-L. Wang, G. Chen, B. Wu, T.-Y. Zeng, C.-Y. Hong, L.-H. Wang and Y.-Z. You, ACS Appl. Mater. Interfaces, 2019, 11, 31735–31742 CrossRef CAS PubMed.
- X. Hu, X. Song, Y. Yuan, X. Yao, X. Chen, G. Li and S. Li, J. Mater. Chem. B, 2023, 11, 6404–6411 RSC.
- E. Ju, M. Peng, Y. Xu, Y. Wang, F. Zhou, H. Wang, M. Li, Y. Zheng and Y. Tao, J. Mater. Chem. B, 2023, 11, 6595–6602 RSC.
- Z. Qin, M. Qiu, Q. Zhang, S. Yang, G. Liao, Z. Xiong and Z. Xu, J. Mater. Chem. B, 2021, 9, 8882–8896 RSC.
- F. Gao, M. Sun, J. Zhang, Y. Chang, W. Gao, G. Ma, X. Ma and Y. Guo, J. Colloid Interface Sci., 2022, 616, 369–378 CrossRef CAS PubMed.
- H. Zhang, R. Han, P. Song, X. Wei, Y. Hou, J. Yu and K. Tang, J. Colloid Interface Sci., 2023, 629, 103–113 CrossRef CAS.
- H. Zhou, X. Li, D. Niu, Y. Li, X. Liu, C. Li, W. Si, J. Cao, Y. Song, G. Wen, Z. Niu and L. Zhang, Adv. Healthcare Mater., 2021, 10, 2002126 CrossRef CAS.
- Y. Jiang, H. Lu, X. Yuan, Y. Zhang, L. Lei, Y. Li, W. Sun, J. Liu, D. Scherman and Y. Liu, J. Mater. Chem. B, 2022, 10, 8082–8093 RSC.
- R. A. Gatenby and R. J. Gillies, Nat. Rev. Cancer, 2004, 4, 891–899 CrossRef CAS.
- M. López-Lázaro, Cancer Lett., 2007, 252, 1–8 CrossRef.
- Z. Cao, L. Zhang, K. Liang, S. Cheong, C. Boyer, J. J. Gooding, Y. Chen and Z. Gu, Adv. Sci., 2018, 5, 1801155 CrossRef.
- Y. Liu, W. Zhen, Y. Wang, J. Liu, L. Jin, T. Zhang, S. Zhang, Y. Zhao, S. Song and C. Li, Angew. Chem., 2019, 131, 2429–2434 CrossRef.
- Y. Liu, W. Zhen, L. Jin, S. Zhang, G. Sun, T. Zhang, X. Xu, S. Song, Y. Wang and J. Liu, ACS Nano, 2018, 12, 4886–4893 CrossRef CAS PubMed.
- D.-H. Zhao, C.-Q. Li, X.-L. Hou, X.-T. Xie, B. Zhang, G.-Y. Wu, F. Jin, Y.-D. Zhao and B. Liu, ACS Appl. Mater. Interfaces, 2021, 13, 55780–55789 CrossRef CAS.
- B. Liu, Y. Bian, S. Liang, M. Yuan, S. Dong, F. He, S. Gai, P. Yang, Z. Cheng and J. Lin, ACS Nano, 2021, 16, 617–630 CrossRef.
- Q. Jiang, B. Qiao, X. Lin, J. Cao, N. Zhang, H. Guo, W. Liu, L. Zhu, X. Xie and L. Wan, Theranostics, 2022, 12, 59 CrossRef CAS.
- A. D. Bokare and W. Choi, J. Hazard. Mater., 2014, 275, 121–135 CrossRef CAS.
- L.-S. Lin, T. Huang, J. Song, X.-Y. Ou, Z. Wang, H. Deng, R. Tian, Y. Liu, J.-F. Wang and Y. Liu, J. Am. Chem. Soc., 2019, 141, 9937–9945 CrossRef CAS PubMed.
- J. Ou, H. Tian, J. Wu, J. Gao, J. Jiang, K. Liu, S. Wang, F. Wang, F. Tong and Y. Ye, ACS Appl. Mater. Interfaces, 2021, 13, 38050–38060 CrossRef CAS.
- X. Zhu, Z. Chu, B. Chen, Q. Jin, X. Ma, J. Yang, Y. Jiang, W. Wang, Z. Zha and H. Qian, Mater. Chem. Front., 2022, 6, 1522–1532 RSC.
- Z. Zhou, Z. Gao, W. Chen, X. Wang, Z. Chen, Z. Zheng, Q. Chen, M. Tan, D. Liu and Y. Zhang, Acta Biomater., 2022, 151, 600–612 CrossRef CAS PubMed.
- H. Li, Y. Liu, S. Li, S. Zhang, B. Huang, R. Cui, Y. Liu and P. Jiang, Nanoscale, 2022, 14, 3788–3800 RSC.
- F. Liu, L. Lin, S. Sheng, C. Xu, Y. Wang, Y. Zhang, D. Wang, J. Wu, Y. Li and H. Tian, Nanoscale, 2020, 12, 1349–1355 RSC.
- Q. Huang, Y. Chen, M. Ye, S. Zhuang, A. Zhong, J. Liu, G. Maduraiveeran, Y. Peng and Y. Huang, Mater. Today Chem., 2024, 40, 102235 CrossRef CAS.
- Z. Yang, C. Shan, J. J. Pignatello and B. Pan, Environ. Sci. Technol., 2022, 56, 6621–6630 CrossRef CAS.
- S. Koo, O. K. Park, J. Kim, S. I. Han, T. Y. Yoo, N. Lee, Y. G. Kim, H. Kim, C. Lim, J. S. Bae, J. Yoo, D. Kim, S. H. Choi and T. Hyeon, ACS Nano, 2022, 16, 2535–2545 CrossRef CAS PubMed.
- S. Wang, Z. Wang, G. Yu, Z. Zhou, O. Jacobson, Y. Liu, Y. Ma, F. Zhang, Z. Y. Chen and X. Chen, Adv. Sci., 2019, 6, 1801986 CrossRef PubMed.
- H.-Y. Wang, Z.-C. Su, X.-W. He, W.-Y. Li and Y.-K. Zhang, Nanoscale, 2021, 13, 12553–12564 RSC.
- J. Wen, X. Liu, L. Liu, X. Ma, A. Fakhri and V. K. Gupta, Colloids Surf., A, 2021, 610, 125683 CrossRef CAS.
- W. Ma, H. Zhang, S. Li, Z. Wang, X. Wu, R. Yan, F. Geng, W. Mu and Y. Jin, ACS Biomater. Sci. Eng., 2022, 8, 1354–1366 CrossRef CAS.
- X. Jing, L. Meng, S. Fan, T. Yang, N. Zhang, R. Xu, X. Zhao, H. Yang, Z. Yang and D. Wang, Chem. Eng. J., 2022, 442, 136096 CrossRef CAS.
- S. Koo, Y. G. Kim, N. Lee, T. Hyeon and D. Kim, Nanoscale, 2023, 15, 13498–13514 RSC.
- L. Peng, X. Yang, S. Wang, Y. K. Chan, Y. Chen, Z. Yang, Y. Mao, L. Li, W. Yang and Y. Deng, Nanoscale, 2022, 14, 2052–2064 RSC.
- Y. Li, J. Wang, T. Zhu, Y. Zhan, X. Tang, J. Xi, X. Zhu, Y. Zhang and J. Liu, Nanoscale, 2024, 16, 8479–8494 RSC.
- P. Zhao, H. Li and W. Bu, Angew. Chem., Int. Ed., 2023, 62, e202210415 CrossRef CAS PubMed.
- S.-L. Li, X. Chu, H.-L. Dong, H.-Y. Hou and Y. Liu, Coord. Chem. Rev., 2023, 479, 215004 CrossRef CAS.
- W. Zuo, Z. Fan, L. Chen, J. Liu, Z. Wan, Z. Xiao, W. Chen, L. Wu, D. Chen and X. Zhu, Acta Biomater., 2022, 147, 258–269 CrossRef CAS.
- L. Liu, C. Han, G. Ding, M. Yu, Y. Li, S. Liu, Y. Xie and J. Liu, Chem. Eng. J., 2022, 450, 138302 CrossRef CAS.
- S. Gao, Y. Jin, K. Ge, Z. Li, H. Liu, X. Dai, Y. Zhang, S. Chen, X. Liang and J. Zhang, Adv. Sci., 2019, 6, 1902137 CrossRef CAS.
- G. Kresse and J. Furthmüller, Phys. Rev. B: Condens. Matter Mater. Phys., 1996, 54, 11169 CrossRef CAS.
- G. Kresse and J. Furthmüller, Comput. Mater. Sci., 1996, 6, 15–50 CrossRef CAS.
- J. P. Perdew, K. Burke and M. Ernzerhof, Phys. Rev. Lett., 1996, 77, 3865 CrossRef CAS PubMed.
- J. P. Perdew, M. Ernzerhof and K. Burke, J. Chem. Phys., 1996, 105, 9982–9985 CrossRef CAS.
- J. Gao, H. b. Yang, X. Huang, S.-F. Hung, W. Cai, C. Jia, S. Miao, H. M. Chen, X. Yang and Y. Huang, Chem, 2020, 6, 658–674 CAS.
- L. Xie, X. Liu, J. Chang, C. Zhang, Y. Li, H. Zhang, S. Zhan and W. Hu, Chem. Eng. J., 2022, 428, 131352 CrossRef CAS.
- B. Yu, W. Wang, W. Sun, C. Jiang and L. Lu, J. Am. Chem. Soc., 2021, 143, 8855–8865 CrossRef CAS PubMed.
- H. Li, J. Shang, Z. Yang, W. Shen, Z. Ai and L. Zhang, Environ. Sci. Technol., 2017, 51, 5685–5694 CrossRef CAS.
- R. X. Y. Zhang, S. Wang, H. Zhu, H. Song, G. Chen, H. Lin, J. Zhang and J. Xiong, J. Hazard. Mater., 2020, 398, 122863 CrossRef PubMed.
- S. Zhan, H. Zhang, X. Mi, Y. Zhao, C. Hu and L. Lyu, Environ. Sci. Technol., 2020, 54, 8333–8343 CrossRef CAS PubMed.
- Y. Zhang, R. Xiao, S. Wang, H. Zhu, H. Song, G. Chen, H. Lin, J. Zhang and J. Xiong, J. Hazard. Mater., 2020, 398, 122863 CrossRef CAS PubMed.
- H. Tada, Nanoscale Adv., 2019, 1, 4238–4245 RSC.
- Y. Yi, L. Wang, G. Li and H. Guo, Catal. Sci. Technol., 2016, 6, 1593–1610 RSC.
- E. J. Jeyaraj, Y. Y. Lim and W. S. Choo, Sci. Rep., 2022, 12, 14890 CrossRef CAS PubMed.
- J. López-García, M. Lehocký, P. Humpolíček and P. Sáha, J. Funct. Biomater., 2014, 5, 43–57 CrossRef PubMed.
- J. López-García, Z. Kuceková, P. Humpolíček, J. Mlček and P. Sáha, Molecules, 2013, 18, 13435–13445 CrossRef PubMed.
- H. Esquer, Q. Zhou, A. D. Abraham and D. V. LaBarbera, SLAS Discovery, 2020, 25, 734–743 CrossRef CAS PubMed.
-
V. Rajendran and M. V. Jain, Cancer Stem Cells: Methods and Protocols, 2018, pp. 89–95 Search PubMed.
- M. Lamkanfi and T.-D. Kanneganti, Int. J. Biochem. Cell Biol., 2010, 42, 21–24 CrossRef CAS.
- K. Boland, L. Flanagan and J. H. Prehn, Cell Death Dis., 2013, 4, e725 CrossRef CAS PubMed.
- S. Gungordu and E. Aptullahoglu, Invest. New Drugs, 2024, 1–9 Search PubMed.
- T. K. Das, M. R. Wati and K. Fatima-Shad, Arch. Neurosci., 2015, 2, e60038 Search PubMed.
- C. You, Z. Gao, H. Wu, K. Sun, L. Ning, F. Lin, B. Sun and F. Wang, J. Mater. Chem. B, 2019, 7, 314–323 RSC.
- H. Nakamura and K. Takada, Cancer Sci., 2021, 112, 3945–3952 CrossRef CAS PubMed.
- K. Li, L. Wu, H. Wang, Z. Fu, J. Gao, X. Liu, Y. Fan, X. Qin, D. Ni and J. Wang, J. Nanobiotechnol., 2024, 22, 546 CrossRef CAS PubMed.
- A. M. Muscarella, W. Dai, P. G. Mitchell, W. Zhang, H. Wang, L. Jia, F. Stossi, M. A. Mancini, W. Chiu and X. H.-F. Zhang, npj Breast Cancer, 2020, 6, 42 CrossRef CAS PubMed.
- X. Guan, Acta Pharm. Sin. B, 2015, 5, 402–418 CrossRef.
|
This journal is © The Royal Society of Chemistry 2025 |
Click here to see how this site uses Cookies. View our privacy policy here.