DOI:
10.1039/D4QI02213K
(Research Article)
Inorg. Chem. Front., 2025,
12, 205-216
Heterogeneous interface engineering to enhance oxygen electrocatalytic activity for rechargeable zinc–air batteries†
Received
1st September 2024
, Accepted 14th November 2024
First published on 20th November 2024
Abstract
The electrocatalytic activity of catalysts can be significantly enhanced through the utilization of heterogeneous structures. Nevertheless, the optimization of both catalytic activity and durability via heterojunction engineering remains a considerable challenge. In this work, we fabricated electrocatalysts of Co/CoO heterojunctions on a highly porous hollow carbon material. The formation of heterojunctions increases the abundance of accessible active sites and optimizes the electrocatalytic reaction kinetics and reactivity. Thus, the prepared catalysts (Co/CoO@N–C-40) deliver robust and stable bifunctional oxygen electrocatalytic activity during the oxygen reduction/evolution reaction (ORR/OER) process. The performance of rechargeable zinc–air batteries (ZABs) greatly depends on bifunctional oxygen electrocatalysts, which are crucial for efficient charging and discharging processes. Consequently, the Co/CoO@N–C-40-based ZABs have superior cycling stability (750 h) and show a stable energy efficiency of 55.10% at 10 mA cm−2 (53.46% after 555 h). This work offers a high-quality oxygen electrocatalyst for ZABs and extends the application of heterogeneous interfacial catalysts in various energy storage and conversion devices.
1. Introduction
The dwindling availability of resources and the increasing scarcity of fossil fuels are pivotal motivations for the advancement of clean and renewable energy alternatives. ZABs have gained substantial attention owing to their notable attributes including high safety standards, cost-effectiveness, and environmental sustainability. Consequently, they have become popular in various applications such as portable electronic devices, electric vehicles, and renewable energy storage systems.1–4 A highly active bifunctional electrocatalyst is typically required at the air cathode to drive ZABs. ORR and OER primarily govern the discharging and charging of rechargeable ZABs on the air cathode, respectively. However, the round-trip energy efficiency is greatly reduced in the ORR and OER processes due to the slower kinetics of multiple electron transfers.5–8 Effective catalysts are essential to accelerate reaction rates. Although precious metal catalysts such as Pt and Ir/Ru demonstrate satisfactory catalytic performance, they are restricted to single reactions (ORR or OER) and cannot be used as multifunctional electrocatalysts.9–12 Furthermore, the limited availability, high expense, and durability of precious metal catalysts significantly obstruct their widespread application. Thus, it is necessary to design cost-effective, high-performing, and robust bifunctional electrocatalysts to facilitate the commercial application of rechargeable ZABs.
Metal–nitrogen–carbon (M–N–C) materials show good bifunctional oxygen electrocatalytic activity and obvious economic benefits, which become ideal cathode candidate materials for ZABs. The incorporation of additional nitrogen atoms leads to a symmetric distribution of charge around the metal center, which enhances the optimization of the adsorption/desorption of O-intermediates, thus boosting the kinetics and activity of the catalyst. Typically, M–N–C materials like Co–Nx and Fe–Nx demonstrate rewarding performance in ORR, but their performance in the OER is weak. The interfacial modulation strategy is a highly effective approach for constructing high-performance bifunctional oxygen electrocatalysts.13–15 The interfacial structure formed between two or more components can modify the electronic structure of active sites and atomic arrangement, leading to improved catalytic performance.16–18 According to previous studies, the interfacial synergistic effect between different components effectively reduced the gap between ORR and OER while lowering the overpotential. In addition, heterogeneous structures can enhance the electrical conductivity and oxygen diffusion/transport capacity at the interface, thus achieving satisfactory electrocatalytic performance. Xu et al.19 designed a c-CoSe2/CoN heterostructures catalyst through phase/interface engineering strategy and realized excellent/highly stable activity in ORR/OER. The heterostructure lengthened the Co–Se/N bond and modulated the local coordination environment. Meanwhile, the d orbitals of the Co atoms were confined to the heterostructure, lowering the thermodynamic barrier of the ORR/OER. The c-CoSe2–CoN cathode of ZAB delivered a stable cycling performance of 250 h. Fan et al.20 fabricated Fe-CoP/Co heterostructures using a heterogeneous interface and doping strategy. The Fe-Co2P/Co@NC catalysts exhibited remarkable accumulation at the Fe–Co interface. The assembled ZABs with Fe-CoP/Co@NC catalysts demonstrated an exceptional open-circuit voltage (OCV) of 1.54 V and prolonged cycling stability of more than 155 h. Despite recent advances in interfacial engineering to improve ORR/OER activity, a well-balanced performance between competing activity and durability remains challenging. Thus, the catalysts with a large number of easily accessible bifunctional active sites and electrochemical stability are essential for enhancing the performance of ZABs.
Herein, we successfully prepared a cobalt/nitrogen-doped bifunctional catalyst (Co/CoO@N–C-40) with Co/CoO heterojunctions in porous hollow carbon substrates by pyrolysis of metal–organic frameworks (MOF)-based materials. The Co/CoO heterogeneous interfaces promote the electron transfer of oxygen-related intermediates and modulate surface engineering. Moreover, this ideal open hollow structure makes active sites more accessible and reduces the diffusion distances between reactants and intermediates within the Co/CoO@N–C-40 catalysts, thereby improving catalytic efficiency. Benefiting from the Co/CoO interface, highly accessible active sites, and the hollow open structure with multistage pores and large specific surface area (SSA), the resulting Co/CoO@N–C-40 catalysts show rapid kinetic and excellent stability towards ORR and OER, with a low potential difference (0.74 V). The Co/CoO@N–C-40 as air cathodes assembled ZABs deliver a fascinating power density of 186 mW cm−2 and remarkable charge/discharge cycling durability of 750 h at 10 mA cm−2.
2. Experimental section
2.1. Chemicals and materials
ZnSO4·7H2O, CoSO4·7H2O, 2-methylimidazole, isopropanol, zinc acetate dihydrate, and potassium hydroxide, were purchased from Aladdin. Pt/C (20 wt%), ruthenium oxide (RuO2), and Nafion solution (5 wt%) were supplied from Sigma-Aldrich.
2.2. Synthesis of Co/CoO@N–C materials
1 mmol of ZnSO4·7H2O and 4 mmol of CoSO4·7H2O were dissolved in 100 mL of methanol to form solution A. 40 mmol of 2-methylimidazole was dissolved in 100 mL of methanol to form solution B. Subsequently, the solution B was poured into solution A under stirring at 20 °C, 40 °C, and 60 °C for 4 h. The resulting precipitates were centrifuged and washed several times with methanol, and then dried overnight at 60 °C and then calcined at 900 °C under Ar atmosphere for 2 h (5 °C min−1) to obtain the final samples, named Co@N–C-20, Co/CoO@N–C-40, and Co@N–C-60, respectively.
3. Results and discussion
3.1. Synthesis, and characterization of electrocatalyst materials
The hollow Co/CoO@N–C-40 materials were fabricated through self-assembling zinc/cobalt sulfate colloids in methanol solution at different heating temperatures. As the reaction temperature increased, the internal dissolution of the spheres led to the development of a hollow structure (Fig. 1).
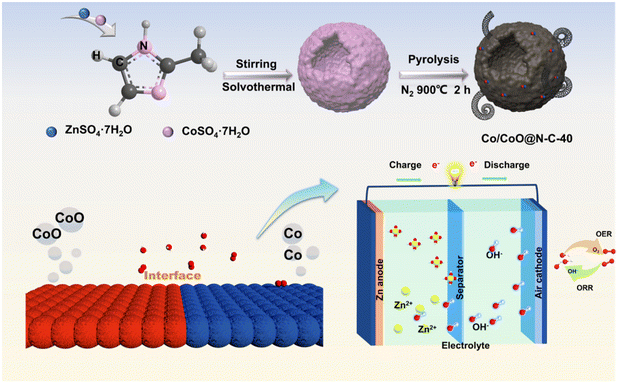 |
| Fig. 1 The synthesis method of Co/CoO@N–C-40 catalyst and schematic illustration of zinc–air battery. | |
The synthesis temperature plays an important role in the morphology of precursor samples. At different temperatures, the reaction kinetics and thermodynamics change, which directly affects the growth and morphology of materials. We explore the effect of different heating temperatures on catalyst precursors, the synthesis of the Co@N–C-20, Co/CoO@N–C-40, and Co@N–C-60 was carried out at 20 °C, 40 °C, and 60 °C, and followed by high-temperature pyrolysis under inert gas. The morphology of catalysts was characterized using scanning electron microscopy (SEM). For Co@N–C-20, the reactant species have lower heat energy, and the migration of atoms and ions in the reactant species is limited. It can be observed that the internal microcrystals are not dissolved and cavity structures are not obtained (Fig. S1†). Co/CoO@N–C-40, due to the increased temperature and the mobility of reactant species increases, the internal microcrystals are completely dissolved, and the thickness of the shell layer is thinned, forming a perfect open hollow structure (Fig. 2a–c). However, when the temperature is further increased, the migration of reactive species becomes faster and more difficult to control, resulting in the collapse of the Co@N–C-60 structure (Fig. S2†). The diameter of the spheres decreases with increasing temperature, Co@N–C-20 around 1 μm and Co/CoO@N–C-40 about 600 nm. Some carbon nanotubes are observed on the Co/CoO@N–C-40 material surface that is catalytically generated by Co/CoO nanoparticles during high-temperature pyrolysis, providing a fast transfer channel for electron transport, and further improving the mass transfer efficiency.21,22 Transmission electron microscopy (TEM) further examined the microstructure of Co/CoO@N–C-40. As shown in Fig. 2d, numerous uniform Co/CoO nanoparticles are embedded in the Co/CoO@N–C-40 substrate. Fig. 2e observes that the nanoparticles are dispersed across the carbon layer, exhibiting an average diameter of 5.8 nm. The strong interaction between these small Co/CoO nanoparticles and the hollow carbon substrate can enhance electron transfer through the synergistic effect.23,24 From the high-resolution transmission electron microscopy (HRTEM) images, it can be seen that there is a heterogeneous interface between the (200) Co facets with a lattice stripe spacing of 0.177 nm and the (200) CoO facets with a lattice stripe spacing of 0.213 nm in a single nanoparticle. The co-existence of the two phases indicates the successful preparation of heterogeneous structures (Fig. 2f). As known that the Schottky barriers formed between Co and CoO facilitate charge separation and also serve as atom traps, capturing O-intermediates to enhance charge transfer of active sites.25,26 The outer layer of nanoparticles has a lattice spacing of 0.34 nm matching the (002) lattice plane of graphitic carbon, implying that the nanoparticles are uniformly encapsulated in the interconnected graphitic carbon shells, facilitating the rapid charge transfer and preventing the nanoparticles from dissolving easily during reaction process.27 Selected area electron diffraction (SAED) mapping shows diffraction rings corresponding to Co and CoO (Fig. S3†). From high-angle annular dark-field scanning TEM (HAADF-STEM) and elements mapping images verify the chemical composition and elemental distribution of Co/CoO@N–C-40, as well as the homogeneous distribution of Co, N, C, and O on the surface of the sample (Fig. 2g).
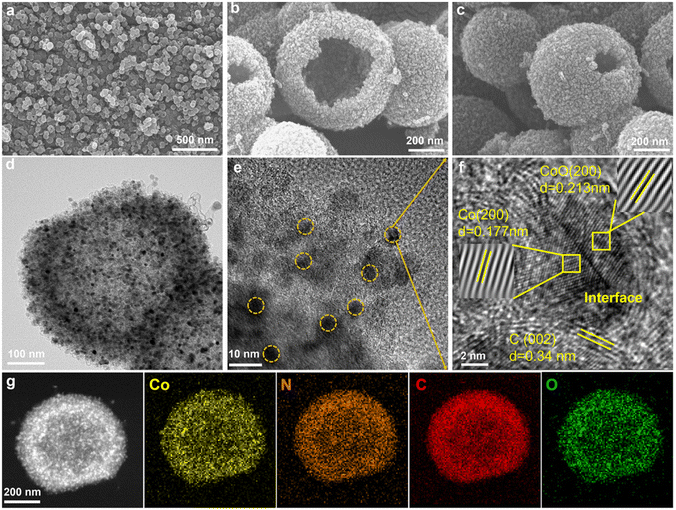 |
| Fig. 2 (a–c) SEM images, (d and e) TEM images, (f) HRTEM image, and (g) HAADF-STEM image and elements mapping of Co, N, C, O for Co/CoO@N–C-40. | |
The crystallinity information of Co@N–C-20 and Co/CoO@N–C-40 and Co@N–C-60 materials was assessed by X-ray diffraction (XRD) technique (Fig. 3a). Co@N–C-20 and Co@N–C-60 exhibit characteristic peaks at about 44.26° and 51.68° attributing to cobalt nanoparticles suggesting that the samples through pyrolysis have few amounts of oxides. The XRD pattern of Co/CoO@N–C-40 shows obvious new peaks at 35.58° and 43.76° indicating the existence of CoO/Co heterostructure.28,29 There is no or less CoO at 20 °C and 60 °C, and we think that the reasons may be attributed to the following: (1) when the synthesis temperature is 20 °C, the reaction conditions are far from the optimum temperature range for the oxidation of Co to CoO. Low temperature limits the activation energy of oxidation reaction or the carbon layer on the surface prevents the formation of CoO. Therefore, Co@N–C-20 is a stacked carbon sphere structure when calcined at high temperatures in an inert atmosphere. (2) For Co/CoO@N–C-40, the mobility of reactants increases and the microcrystals dissolve completely, forming an open hollow structure with a large specific surface area. Co/CoO can be uniformly dispersed on the surface of the carbon layer. (3) When the synthesis temperature at 60 °C, the morphology of Co@N–C-60 collapses due to the increase of temperature and the acceleration of reaction rate, and some of the CoO and Co compact clusters may be reduced by cobalt on the surface of the carbon layer, so the diffraction peak of CoO cannot be detected. The defect structure of the catalyst materials was measured using Raman spectroscopy. The peaks are observed at 1350 and 1590 cm−1 conforming to the disordered carbon defects (D) and ordered graphitic carbon (G).30 A higher ID/IG signifies more defect structure in catalysts. For Co/CoO@N–C-40, the value of ID/IG (1.07) is larger than that of Co@N–C-20 (0.90), demonstrating a greater degree of graphitization and more defect sites in Co/CoO@N–C-40, which promotes electron transfer in electrocatalytic processes (Fig. 3b). The slightly higher disordered carbon defects in Co@N–C-60 (1.12) than in Co/CoO@N–C-40 are due to the collapse of its sample morphology. The catalytic activity is closely related to the SSA of a catalyst. A larger SSA implies more contact area with the reactants, which can enhance abundant active sites available for the catalytic process.31,32 The porosity and SSA of Co@N–C-20 and Co/CoO@N–C-40 and Co@N–C-60 materials were determined using N2 adsorption/desorption curves (Fig. 3c). The Co/CoO@N–C-40 exhibits mesoporous and hollow structures, with a SSA of 543.8 m2 g−1. The isothermal curves of Co@N–C-20 and Co/CoO@N–C-40 and Co@N–C-60 show a mixture of type I and type IV features, revealing that all samples possessed multistage pore structures. The rapid adsorption enhancement phenomenon at low relative pressures suggests that the samples contain abundant microporous structures inside, and the formation of such micropores is mainly attributed to the Zn evaporation process.33,34Fig. 3d illustrates the porosity of Co/CoO@N–C materials, all samples are enriched with a high density of micropores and mesopores, with a relatively minor presence of macropores. Micropores serve as crucial carriers for catalyst active sites, offering additional potential active sites during the catalytic process. The rich mesopore network structure greatly expands the electron transport pathway and significantly shortens the diffusion time and spatial distance of the active substance in the pore.35,36 Especially, the Co/CoO@N–C-40 heterogeneous structure catalysts, due to their high SSA and complex multilevel pore structure, ensure sufficient contact interfaces between the catalytic active sites, reactants, and electrolytes during the ORR/OER reaction, and construct a diverse and highly efficient substance transport pathway through its special pore layout, accelerating the mass transfer rate, thus strongly enhancing the ORR/OER catalytic activity.37,38 The elemental composition, as well as the valence changes of catalyst materials, were investigated by X-ray photoelectron spectroscopy (XPS). The survey spectrum indicated the existence of elements C, N, O, and Co (Fig. S4 and Table S1†). The high-resolution Co 2p spectra present three different kinds of Co, which are Co0 (778.5 eV), Co–O (779.9 eV), and Co–Nx (781.8 eV), with a satellite peak (Sat.) observed at 785.2 eV (Fig. 3e).39,40 Moreover, the highest content of Co–Nx in Co/CoO@N–C-40 can be observed from Fig. S5,† and Co–Nx is often involved in catalytic reactions as active sites.29 The high-resolution C 1s spectra show peaks at 284.8 eV (C–C), 286.2 eV (C–N) as well as 288.2 eV (O
C–O) (Fig. 3f). The formation of C–N bonds due to their inherent electronegativity difference resulted in an asymmetric bond structure, which can induce the generation of an asymmetric spin/charge density distribution in its peripheral region, enhancing the adsorption capacity of the catalyst for the reactive species, and thus comprehensively enhanced the performance of the catalysts.41,42 The O 1s spectrum reveals Co–O bonds (530.9 eV), oxygen vacancies (531.98 eV), and adsorbed water (533.3 eV), respectively (Fig. 3g). Notably, Co/CoO@N–C-40 has the most oxygen vacancies and oxygen defects from Fig. S6.† Generally, oxygen vacancies contribute to the generation of catalytically active sites and increase the electronic conductivity of the sample, which in turn effectively enhances the electrochemical reaction activity of the catalyst.43 The electron paramagnetic resonance (EPR) spectrum was performed to demonstrate the presence of oxygen vacancies. Fig. S7† shows that the peak intensity of Co/CoO@N–C-40 is stronger compared to other samples, indicating the presence of more oxygen vacancies. The abundance of oxygen vacancies acts synergistically with the nearby active metal sites to enhance the efficiency of the catalytic reaction. The introduction of oxygen vacancies not only optimizes the coordination environment of the metal center and improves its conductivity, but also accelerates the adsorption of OER intermediates, thus improving OER performance.44,45 The N 1s spectrum can be deconvoluted into five nitrogen species including pyridine nitrogen at 398.4 eV, Co–Nx at 399.1 eV, pyrrole nitrogen at 400.2 eV, graphitic nitrogen at 401.2 eV, and oxidized nitrogen at 404.0 eV (Fig. 3h).46 The pyridine nitrogen can activate the neighboring C atoms by synergizing with other components and giving them positive charges to form defects, which in turn increases the active sites and strengthens the catalytic performance. Co–Nx can directly participate in the reaction as the active center of the catalyst, while its strong coordination bonds can also stabilize the structure of the catalyst and effectively prevent the Co atoms from dissolving or being lost in the reaction.47 Graphite N improves electronic conductivity and accelerates electron transfer. Among them, the active species of pyridine nitrogen and Co–Nx in Co/CoO@N–C-40 are the highest, which also provides support for the exceptional catalytic ability of Co/CoO@N–C-40 from the side (Fig. 3i).
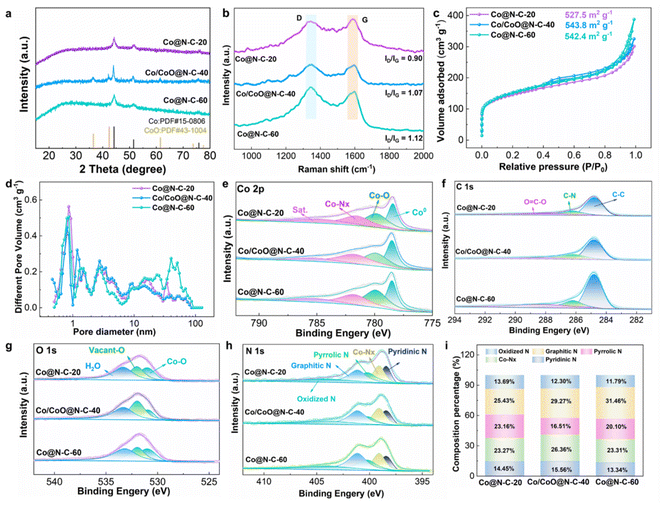 |
| Fig. 3 (a) XRD patterns, (b) Raman spectroscopy, (c) N2 adsorption/desorption curves, (d) corresponding pore size distributions, high-resolution XPS spectra of (e) Co 2p, (f) C 1s, (g) O 1s, and (h) N 1s for Co@N–C-20, Co/CoO@N–C-40, and Co@N–C-60. (i) Content of N species for Co/CoO@N–C-40. | |
3.2. Electrocatalytic performances of electrocatalyst for ORR
The fabricated catalyst materials were tested in 0.1 M KOH solution (saturated O2) to test the ORR activity. The cyclic voltammetry (CV) curve exhibits that Co/CoO@N–C-40 has distinguished a redox peak (Fig. 4a). The ORR activity of all catalysts was evaluated by linear sweep voltammetry (LSV). Under the same circumstances, the ORR activity of the Pt/C was examined for comparison. Co/CoO@N–C-40 reveals a half-wave potential (E1/2) of 0.854 V and the limited current density (JL) of 5.26 mA cm−2, which are preferred to Co@N–C-20, Co@N–C-60 and Pt/C (Fig. 4b and c). The outstanding ORR activity of Co/CoO@N–C-40 is due to the abundance of defects created at the hetero-interface of Co/CoO and the exposure of hetero-interfacial defects enhances the reaction activity.19 In addition, the carbon nanotubes on the surface of the carbon spheres provide fast transfer channels for electron transport, which further improves the mass transfer efficiency. Tafel slopes were determined from the LSV curves of catalysts in order to investigate the fast kinetics during the ORR reaction process (Fig. 4d). Co/CoO@N–C-40 shows a smaller Tafel slope of 73.44 mV dec−1 than Co@N–C-20 (80.12 mV dec−1), Co@N–C-60 (76.44 mV dec−1), and Pt/C (105.7 mV dec−1), suggesting that the unique open hollow carbon sphere structure and the heterogeneous interfaces can accelerate the reaction kinetic process. In Fig. 4e, rotating disc electrode (RDE) tests were carried out at different rotational speeds (ranging from 400 to 2500 rpm) to further explore the reaction pathway and the number of electrons transferred (n). The Koutechy–Levich (K–L) equation was used to calculate the n of 3.99 for Co/CoO@N–C-40, indicating the direct 4 electrons (4e−) pathway to the ORR. Further rotating ring disc electrode (RRDE) tests were conducted to measure the n and the H2O2 yield in the ORR reaction on the catalyst, as shown in Fig. 4f. The results of n for all samples between 3.95 and 3.99 within the voltage range of 0.2 to 0.8 V are consistent with the K–L curve results described above. Moreover, the Co/CoO@N–C-40 also exhibited a minimal H2O2 yield of 1.12%, this result proved that the Co/CoO@N–C-40 mainly followed the direct 4e− transfer pathway during the ORR reaction. Stability is a crucial factor in assessing the ORR character of a catalyst. The E1/2 of Co/CoO@N–C-40 is only slightly attenuated after 5000 cycles (negative shift of 7 mV), superior to Pt/C (negative shift of 37 mV) (Fig. S8†), demonstrating that Co/CoO@N–C-40 has outstanding stability. Importantly, there is also no significant change in Co/CoO@N–C-40 morphology after long-term cycling, proving its durable stability (Fig. S9†).
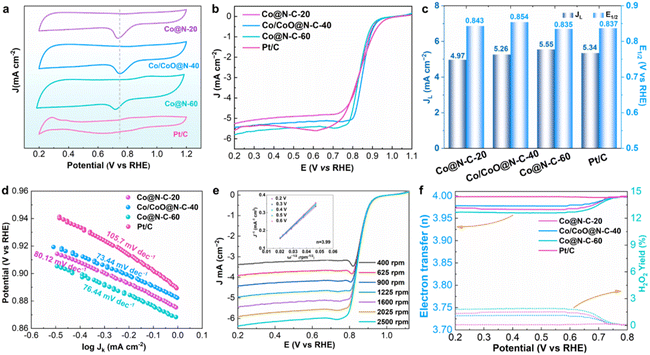 |
| Fig. 4 (a) CV curves in an O2-saturated 0.1 M KOH solution. (b) LSV curves for ORR. (c) Comparison of E1/2 and JL. (d)Tafel plots. (e) LSV curves for Co/CoO@N–C-40 at different rotation rates (400–2500 rpm) and calculated K–L plots (insets) at different potentials (0.2–0.6 V). (f) Electron transfer and H2O2 yield. | |
3.3. Electrocatalytic performances of electrocatalyst for OER
In addition to exhibiting excellent ORR activity, the Co/CoO@N–C-40 possesses high-performance OER activity as well. Fig. 5a demonstrates the LSV curves of OER for Co@N–C-20, Co/CoO@N–C-40, Co@N–C-60, and the reference catalyst RuO2 in 1.0 M KOH solution. Co/CoO@N–C-40 achieves a low overpotential of 349 mV at 10 mA cm−2, exceeding Co@N–C-20 (370 mV), and Co@N–C-60 (400 mV) (Fig. 5b). Notably, Co/CoO@N–C-40 is slightly less effective than RuO2 (325 mV). This suggests that the formation of heterojunctions in Co/CoO@N–C-40 enhances both the chemical adhesion and electrical coupling between Co/CoO and hollow carbon substrate, potentially modulating the electronic structure and remarkably boosting OER activity.48,49 Smaller Tafel slopes generally indicate better reaction kinetics for OER. In Fig. 5c, Co/CoO@N–C-40 exhibits a small Tafel slope of 137 mV dec−1, which outperforms Co@N–C-20 (193 mV dec−1), Co@N–C-60 (169 mV dec−1), and RuO2 (187 mV dec−1), suggesting that at lower potentials, Co/CoO@N–C-40 promotes rapid charge transfer across the electrocatalytic interface. Moreover, Co/CoO@N–C-40 can create more interfaces in Co/CoO heterojunctions, facilitating the transition from Co-related reactive to reactive species. The electrochemically active surface area (ECSA) of the electrocatalyst was evaluated by the electrochemical double layer capacitance (Cdl). The value of Co/CoO@N–C-40 was calculated to be 21.3 mF cm−2, which was larger than that of its counterparts, Co@N–C-20 (17.5 mF cm−2) and Co@N–C-60 (7.95 mF cm−2) (Fig. 5d and S10†). Co/CoO@N–C-40 heterogeneous interface construction and hollow structure with easily approachable active sites promote high ECSA.50 The Co/CoO@N–C-40 exhibits a charge transfer resistance of 10.56 Ω from the electrochemical impedance spectroscopy (EIS) plots, which is substantially lower than that of Co@N–C-20 and Co@N–C-60 (Fig. 5e and S11†). Motivated by previous reports, heterogeneous interfaces produced from Co/CoO can alter the electronic structure of Co atoms, boost the density and conductivity of the active sites, and provide moderate adsorption strength for O-intermediates.51 In addition, Co/CoO@N–C-40 open hollow carbon nanosphere structure as well as surface-attached carbon nanotubes synergistically enhance the charge transfer efficiency and optimize the kinetic behavior of the mass transfer. Co/CoO@N–C-40 also shows long-lasting activity for OER. After 5000 CV cycles, the Co/CoO@N–C-40 overpotential only shifted negatively by 11 mV. After 15 h of continuous operation at 10 mA cm−2, the Co/CoO@N–C-40 current density can retain 87.2% of its initial value (Fig. 5f and inset). These results suggest that the hollow structure of Co/CoO@N–C-40 promotes the formation of heterojunctions, strengthening the structural and chemical stability, and thereby stabilizing the OER activity of Co/CoO@N–C-40.
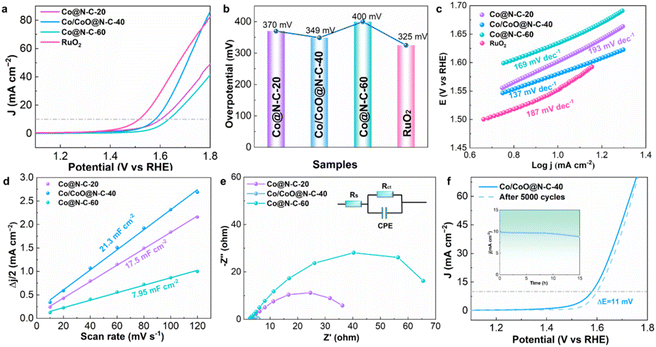 |
| Fig. 5 (a) LSV curves of OER. (b) Comparison of overpotentials at 10 mA cm−2. (c) Tafel plots. (d) Plots for calculating Cdl of Co/CoO@N–C catalysts for the OER process. (e) Nyquist curves. (f) LSV curves of Co/CoO@N–C-40 for OER before and after 5000 CV cycles in 1.0 M KOH. (The inset shows the chronoamperometric (i–t) responses of Co/CoO@N–C-40.) | |
3.4 Activity behavior of the electrocatalyst
Selective poisoning and acid treatment experiments were performed to further elucidate the catalytic sites of Co/CoO@N–C-40 for ORR and OER. It is well known that thiocyanate ions (SCN−) can easily coordinate with metal ions and disrupt and inhibit the reactions catalyzed by metal species.52 The acid etching can effectively remove metal nanoparticles from the catalyst.53 The performance of Co/CoO@N–C-40 catalysts shows a significant reduction after acid treatment compared to the poisoning of the SCN− sample (Fig. 6a and b), suggesting that ORR activity is primarily affected by the N-doped carbon frameworks. And the electrocatalytic activity of the SCN− and acid-treated Co/CoO@-N–C-40 catalysts display substantial degradation in the OER (Fig. 6c and d), suggesting that Co/CoO species embedded in the carbon frameworks play the predominate role in the OER process. These results confirm that the metal sites contributed to both ORR and OER reactions but predominately to OER.54 Thus the superior ORR/OER behavior of Co/CoO@N–C-40 can be attributed to the synergistic interactions between multiple active components and unique structural features: (1) the unique layered porous and hollow structure of the MOF-derived 3D carbon spheres improves the utilization of catalytically active sites, promotes rapid electron transfer and enhances the transport of the electrolyte solution and oxygen molecules (2) the synergistic interactions between multiple active sites (e.g. carbon encapsulated metal nanoparticles, Co–Nx, and N-doped carbon) increase the reaction sites of oxygen molecules and promote the adsorption of reaction intermediates (OH*, O*, OOH*), enhancing the ORR/OER catalytic activity. (3) Strong electronic coupling between the different components of the Co/CoO heterojunction multiphase structure occurs during the reaction process, which optimizes the electronic structure, lowers the reaction energy barriers, improves the binding capacity of the catalyst for interaction with reaction intermediates, and promotes the chemisorption of highly reactive substances onto the catalyst surface, leading to a significant increase in the bifunctional catalytic activity.55 (4) The introduction of a large number of oxygen vacancies can further increase the number of active sites and density of states (DOS) near the Fermi level, reduce the charge transfer resistance, and accelerate the electron transfer, thus enhancing the reaction kinetic process.56,57
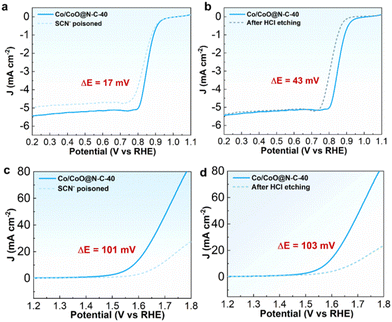 |
| Fig. 6 (a and b) ORR and (c and d) OER catalytic activity of Co/CoO@N–C-40 affected by SCN− poisoning (10 mM) and acid leaching in 3 M HCl, respectively. | |
3.5. Electrochemical performance of electrocatalyst in ZABs
To assess the bifunctional activity of catalysts in ZABs, the ΔE between the potential of OER at 10 mA cm−2 and the E1/2 of the ORR was calculated. The smaller values of ΔE (ΔE = Ej=10 − E1/2) are typically attributed to more superior bifunctional oxygen electrocatalytic activity.11,35 In comparison to the Co@N–C-20 (ΔE = 0.757 V) and Co@N–C-60 (ΔE = 0.795 V), Co/CoO@N–C-40 achieves a low ΔE of 0.725 V, implying that the open hollow carbon sphere structure of Co/CoO@N–C-40 can efficiently expose more active centers (Fig. S12†). Carbon nanotubes attached to the surface of the carbon sphere can further improve the charge transfer efficiency, thus significantly enhancing the ORR activity as well as the OER activity of the catalyst.3,22 Co/CoO@N–C-40 demonstrates exceptional ORR and OER activity, which makes it air cathodes in rechargeable ZABs to explore its feasibility in practical applications (Fig. 7a). The ZAB assembled with Co/CoO@N–C-40 delivers an impressive OCV of 1.468 V surpassing Pt/C + RuO2 (1.443 V) (Fig. 7b). As illustrated in Fig. 7c, the Co/CoO@N–C-40-based battery reaches a high-power density of 186 mW cm−2, outperforming Pt/C + RuO2-based ZAB (104 mW cm−2). The discharge curves present that the Co/CoO@N–C-40-based batteries outperform the Pt/C + RuO2 batteries in terms of higher discharge plateau and excellent rate performance at different current densities (from 1 to 50 mA cm−2) (Fig. 7d). The voltage plateau of Co/CoO@N–C-40-based ZAB remains stable and undecayed even at high current densities, indicating is promising for high-current output application scenarios. Moreover, ZABs assembled with Co/CoO@N–C-40 were first discharged to 10 mA cm−2 at 1.3 V and finally returned to 10 mA cm−2 at 1.297 V with negligible decay, demonstrating their excellent reversibility and recoverability. Notably, Co/CoO@N–C-40-based ZABs also delivered longer discharge times at different current densities 10 mA cm−2, 25 mA cm−2, and 50 mA cm−2 (Fig. S13–S15†), with discharge time of 164 h (1.29 V), 54.6 h (1.24 V), and 26.8 h (1.15 V), respectively, which are superior to the Pt/C + RuO2-based ZABs (151 h, 1.21 V; 48.6 h, 1.11 V; and 20.3 h, 0.96 V). The mass of anode zinc flake loss at a constant discharge current density of 10 mA cm−2 was used to evaluate the discharge specific capacity of ZABs (Fig. S16†). The ZABs constructed using the Co/CoO@N–C-40 endowed a high discharge capacity of 808 mA h g−1, compared to 636 mA h g−1 for ZABs assembled with Pt/C + RuO2. Co/CoO@N–C-40 with heterogeneous interface structure enhances the numerous active sites, thus significantly increasing the energy density of ZABs. Additionally, the hollow structure of Co/CoO@N–C-40 facilitates the regulation of the stress from volume changes in the charging and discharging process, which makes the electrode material polarize less quickly and cycle more effectively.6 Attractively, the Co/CoO@N–C-40 as air cathodes assembled with ZABs exhibit impressive charging and discharging ability and a remarkably longer lifetime of 750 h (Fig. 7e). In contrast, ZABs constructed with Pt/C + RuO2 showed a more pronounced degradation of cycling performance less than 200 h. Furthermore, Co/CoO@N–C-40-based ZABs realize stable and consistent round-trip efficiencies (initial: 55.14%; final: 53.46%) for up to 555 h, revealing its excellent charging and discharging capability (Fig. 7f). The key parameters of the recently reported ZABs are presented in Fig. 7g and Table S2,† and the observations of distinct advantages of Co/CoO@N–C-40-based ZABs, especially in terms of cycle stability and specific capacity, are evident from the comprehensive analysis. The main reason for the excellent cycling performance of Co/CoO@N–C-40-based batteries under long-term use is that the internal nanoparticles are uniformly embedded and encapsulated in the intertwined graphitic carbon-shell structure. This design improves the charge transfer rate and material transfer efficiency while preventing nanoparticle dissolution during the catalytic process, ensuring the overall structural stability and durability of the catalyst. The competitive ZABs performance suggests that Co/CoO@N–C-40 could serve as a promising substitute for precious metal-based electrocatalysts.
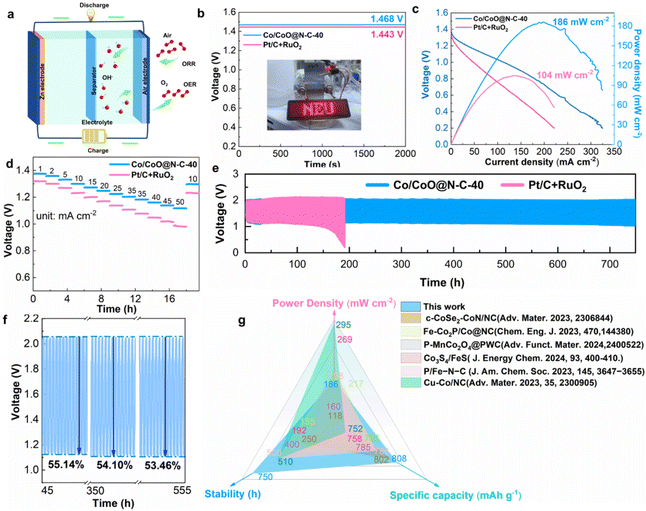 |
| Fig. 7 (a) Schematic configuration of the homemade ZAB. (b) Open-circuit plots of ZABs assembled with catalysts (Co/CoO@N–C-40 and Pt/C + RuO2). (c) Discharging curves and corresponding power density curves. (d) Rate performance at various current densities. (e) Cycling performance of ZABs at 10 mA cm−2. (f) The round-trip efficiency of ZAB at 50 h, 355 h, and 555 h. (g) Performance comparison of Co/CoO@N–C-40 and other air electrodes reported recently. | |
4. Conclusion
In conclusion, CoO/Co heterogeneous interface electrocatalysts were synthesized using a simple and efficient method to construct bifunctional oxygen electrocatalysts with hollow carbon sphere structures. The rational design of Co/CoO@N–C-40 improves the charge transfer efficiency and electrocatalytic activity towards ORR and OER. Due to the abundance of oxygen vacancies, the Co/CoO@N–C-40 catalyst exhibited excellent electrochemical performance with a low overpotential of 340 mV and a high half-wave potential of 0.854 V. The outstanding OER and ORR bifunctional catalytic properties of Co–N–C-40 can be attributed to the ultra-high specific surface area (543.8 m2 g−1), inhomogeneous interfacial structure, a large number of oxygen vacancies, and synergistic coupling effects between active substances such as pyridine-N, graphite-N, and CoO/Co species. In addition, the ZAB constructed with Co/CoO@N–C-40 as an air cathode exhibits an excellent power density of 186 mW cm−2 and excellent long-term durability (750 h) at a current density of 10 mA cm−2. This work offers a new way for the preparation of heterostructure catalysts to enrich bifunctional oxygen catalysts with ORR/OER for clean energy storage and conversion devices.
Author contributions
Tao-Tao Li: writing, data curation, investigation, conceptualization. Yu-Rui Ji: data curation, investigation. Yi-Meng Wu: visualization, review. Peng-Fei Wang: review. Zonglin Liu: review, writing. Jie Shu: review. Ting-Feng Yi: investigation, review, supervision, funding acquisition. All authors have given approval to the final version of the manuscript.
Data availability
The data supporting this article have been included as part of the ESI.†
Conflicts of interest
The authors declare no competing financial interest.
Acknowledgements
This work was supported by the Hebei Provincial Natural Science Foundation (no. E2024501010), the National Natural Science Foundation of China (no. 52374301), the Shijiazhuang Basic Research Project (no. 241790667A), the Fundamental Research Funds for the Central Universities (no. N2423054), and the Performance Subsidy Fund for Key Laboratory of Dielectric and Electrolyte Functional Material Hebei Province (no. 22567627H).
References
- Q. Wang, S. Kaushik, X. Xiao and Q. Xu, Sustainable zinc-air battery chemistry: Advances, challenges and prospects, Chem. Soc. Rev., 2023, 52, 6139–6190 RSC.
- Y. Yu, P. Rao, S. Feng, M. Chen, P. Deng, J. Li, Z. Miao, Z. Kang, Y. Shen and X. Tian, Atomic Co clusters for efficient oxygen reduction reaction, Acta Phys.-Chim. Sin., 2023, 39, 2210039 Search PubMed.
- Q. Liu, R. Liu, C. He, C. Xia, W. Guo, Z.-L. Xu and B. Y. Xia, Advanced polymer-based electrolytes in zinc–air batteries, eScience, 2022, 2, 453–466 CrossRef.
- Q. Wu, T. Xie, L. Zhang, H. Gao, J. Jiang, T. Zhao and G. Xu, CoFe formic-acid framework derived S, N co-doped carbon nanotube composites decorated with a bimetallic sulfide as a bifunctional electrocatalyst for rechargeable zinc–air batteries, Inorg. Chem. Front., 2024, 11, 1852–1861 RSC.
- P. Zhang, K. Chen, J. Li, M. Wang, M. Li, Y. Liu and Y. Pan, Bifunctional single-atom catalysts for rechargeable zinc–air batteries: From dynamic mechanism to rational design, Adv. Mater., 2023, 35, 2303243 CrossRef CAS PubMed.
- J.-X. An, Y. Meng, L. Fang, Z. Lyu, S. Tang, T. Li, C. Liu, H. Shin, J. Lee, S. Ding, I. D. Kim and J. C. Li, Surface engineering strategy to synthesize bicomponent carbons for rechargeable zinc-air batteries, Energy Storage Mater., 2024, 70, 103520 CrossRef.
- M. Zhang, H. Li, J. Chen, F.-X. Ma, L. Zhen, Z. Wen and C.-Y. Xu, High-loading co single atoms and clusters active sites toward enhanced electrocatalysis of oxygen reduction reaction for high-performance Zn–air battery, Adv. Funct. Mater., 2023, 33, 2209726 CrossRef CAS.
- X. Zhou, M. Wu, K. Chen, H. Wang, Z. Wen and S. Ci, γ-Fe2O3 decorating N, S co-doped carbon nanosheets as a cathode electrocatalyst for different-scenario fuel cells, Inorg. Chem. Front., 2024, 11, 4625–4637 RSC.
- J. H. Park, C. H. Lee, J.-M. Ju, J.-H. Lee, J. Seol, S. U. Lee and J.-H. Kim, Bifunctional covalent organic framework-derived electrocatalysts with modulated p-band centers for rechargeable Zn–air batteries, Adv. Funct. Mater., 2021, 31, 2101727 CrossRef CAS.
- Y. Tan, Y. Wang, A. Li, X. Jiang, Y. Zhang and C. Cheng, Superhydrophobic Co/Fe sulfide nanoparticles and single-atoms doped carbon nanosheets composite air cathode for high-performance zinc-air batteries, J. Energy Chem., 2024, 96, 568–577 CrossRef CAS.
- Y. Liu, S. Liu, P. Zhang, J. Zhou, H. Liu, S. Li, X. Li, X. Wang, D. Han, Y. Chen, Y. Wang, J. Jiang and B. Li, Electronic structure regulation of MnCo2O4 via surface-phosphorization coupling to monolithic carbon for oxygen electrocatalysis in Zn–air batteries, Adv. Funct. Mater., 2024, 2400522 CrossRef CAS.
- L. Li, S. Jia, Y. Shi, C. Wang, H. Qiu, Y. Ji, M. Cao and D. Zhang, Advanced aqueous zinc-ion batteries based on an anode constructed from zinc powder: A review, Inorg. Chem. Front., 2024, 11, 4485–4498 RSC.
- H. Li, W. Wang, S. Xue, J. He, C. Liu, G. Gao, S. Di, S. Wang, J. Wang, Z. Yu and L. Li, Superstructure-assisted single-atom
catalysis on tungsten carbides for bifunctional oxygen reactions, J. Am. Chem. Soc., 2024, 146, 9124–9133 CrossRef CAS PubMed.
- C. Lan, Y. Chu, S. Wang, C. Liu, J. Ge and W. Xing, Research progress of proton-exchange membrane fuel cell cathode nonnoble metal M-Nx/C-type oxygen reduction catalysts, Acta Phys.-Chim. Sin., 2023, 39, 2210036 Search PubMed.
- Y. Niu, X. Teng, S. Gong, X. Liu, M. Xu and Z. Chen, Boosting oxygen electrocatalysis for flexible zinc-air batteries by interfacing iron group metals and manganese oxide in porous carbon nanowires, Energy Storage Mater., 2021, 43, 42–52 CrossRef.
- Q. Xu, J. Zhang, H. Zhang, L. Zhang, L. Chen, Y. Hu, H. Jiang and C. Li, Atomic heterointerface engineering overcomes the activity limitation of electrocatalysts and promises highly-efficient alkaline water splitting, Energy Environ. Sci., 2021, 14, 5228–5259 RSC.
- Y. Li, L. Zhang, Y. Han, W. Ji, Z. Liu, B. Wang, S. Zhao, X. Wu, L. Zhang and R. Zhang, Interface engineering of bifunctional oxygen electrocatalysts for rechargeable Zn–air batteries, Mater. Chem. Front., 2023, 7, 4281–4303 RSC.
- Q. Lu, H. Wu, X. Zheng, Y. Cao, J. Li, Y. Wang, H. Wang, C. Zhi, Y. Deng, X. Han and W. Hu, Controllable constructing Janus homologous heterostructures of transition metal alloys/sulfides for efficient oxygen electrocatalysis, Adv. Energy Mater., 2022, 12, 2202215 CrossRef CAS.
- X. Xu, X. Wang, S. Huo, X. Liu, X. Ma, M. Liu and J. Zou, Modulation of phase transition in cobalt selenide with simultaneous construction of heterojunctions for highly-efficient oxygen electrocatalysis in zinc-air battery, Adv. Mater., 2024, 36, 2306844 CrossRef CAS.
- F. Fan, Q. Huang, K. K. Rani, X. Peng, X. Liu, L. Wang, Z. Yang, D. Huang, R. Devasenathipathy, D.H. Chen, Y. Fan and W. Chen, Interface and doping engineering of co-based electrocatalysts for enhanced oxygen reduction and evolution reactions, Chem. Eng. J., 2023, 470, 144380 CrossRef CAS.
- S. Chen, L. Ma, S. Wu, S. Wang, Z. Li, A. A. Emmanuel, M. R. Huqe, C. Zhi and J. A. Zapien, Uniform virus-like Co–N–Cs electrocatalyst derived from Prussian blue analog for stretchable fiber-shaped Zn–air batteries, Adv. Funct. Mater., 2020, 30, 1908945 CrossRef CAS.
- H. Chang, L. Zhao, S. Zhao, Z.-L. Liu, P.-F. Wang, Y. Xie and T.-F. Yi, Tuning interface mechanism of FeCo alloy embedded N, S-codoped carbon substrate for rechargeable Zn-air battery, J. Energy Chem., 2024, 93, 400–410 CrossRef CAS.
- W. Li, J. Liu, P. Guo, H. Li, B. Fei, Y. Guo, H. Pan, D. Sun, F. Fang and R. Wu, Co/CoP heterojunction on hierarchically ordered porous carbon as a highly efficient electrocatalyst for hydrogen and oxygen evolution, Adv. Energy Mater., 2021, 11, 2102134 CrossRef CAS.
- Y. Guo, F. Liu, L. Feng, X. Wang, X. Zhang and J. Liang, Single co atoms anchored on nitrogen-doped hierarchically ordered porous carbon for selective hydrogenation of quinolines and efficient oxygen reduction, Chem. Eng. J., 2022, 429, 132150 CrossRef CAS.
- Y. Jiang, Y. P. Deng, J. Fu, D. U. Lee, R. Liang, Z. P. Can, Y. Liu, Z. Bai, S. Hwang, L. Yang, D. Su, W. Chu and Z. Chen, Interpenetrating triphase cobalt-based nanocomposites as efficient bifunctional oxygen electrocatalysts for long-lasting rechargeable Zn–air batteries, Adv. Energy Mater., 2018, 8, 1702900 CrossRef.
- A. Aijaz, J. Masa, C. Rösler, W. Xia, P. Weide, A. J. R. Botz, R. A. Fischer, W. Schuhmann and M. Muhler, Co@Co3O4 encapsulated in carbon nanotube-grafted nitrogen-doped carbon polyhedra as an advanced bifunctional oxygen electrode, Angew. Chem., Int. Ed., 2016, 55, 4087–4091 CrossRef CAS PubMed.
- M. Luo, W. Sun, B. B. Xu, H. Pan and Y. Jiang, Interface engineering of air electrocatalysts for rechargeable zinc–air batteries, Adv. Energy Mater., 2021, 11, 2002762 CrossRef CAS.
- X. Wu, Z. Zhang, C. He, Y. Shen, X. Wu, H. Wang, Z. Ma and Q. Li, Mixed-valence cobalt oxides bifunctional electrocatalyst with rich oxygen vacancies for aqueous metal-air batteries, Chem. Eng. J., 2023, 453, 139831 CrossRef CAS.
- T. Zhou, W. Xu, N. Zhang, Z. Du, C. Zhong, W. Yan, H. Ju, W. Chu, H. Jiang, C. Wu and Y. Xie, Ultrathin cobalt oxide layers as electrocatalysts for high-performance flexible Zn–air batteries, Adv. Mater., 2019, 31, 1807468 CrossRef.
- J. Liu, X. Meng, J. Xie, B. Liu, B. Tang, R. Wang, C. Wang, P. Gu, Y. Song, S. Huo and J. Zou, Dual active sites engineering on sea urchin-like conis hollow nanosphere for stabilizing oxygen electrocatalysis via a template-free vulcanization strategy, Adv. Funct. Mater., 2023, 33, 2300579 CrossRef CAS.
- X. Zhao, M. Chen, Z. Bi, H. Zhang, G. Hu and Y. Zhou, Double-confinement construction of atomically-dispersed-Fe bifunctional oxygen electrocatalyst for high-performance zinc-air battery, Small, 2023, 19, 2304854 CrossRef CAS.
- J. Qiao, Y. You, L. Kong, W. Feng, H. Zhang, H. Huang, C. Li, W. He and Z. Sun, Precisely constructing orbital-coupled Fe-Co dual-atom sites for high-energy-efficiency Zn-air/iodide hybrid batteries, Adv. Mater., 2024, 2405533 CrossRef CAS PubMed.
- Z. Li, S. Ji, C. Xu, L. Leng, H. Liu, J. H. Horton, L. Du, J. Gao, C. He, X. Qi, Q. Xu and J. Zhu, Engineering the electronic structure of single-atom iron sites with boosted oxygen bifunctional activity for zinc-air batteries, Adv. Mater., 2023, 35, 2209644 CrossRef CAS.
- H. Zhang, H.-C. Chen, S. Feizpoor, L. Li, X. Zhang, X. Xu, Z. Zhuang, Z. Li, W. Hu, R. Snyders, D. Wang and C. Wang, Tailoring oxygen reduction reaction kinetics of Fe−N−C catalyst via spin manipulation for efficient zinc-air batteries, Adv. Mater., 2024, 36, 2400523 CrossRef CAS PubMed.
- Y. Zhou, R. Lu, X. Tao, Z. Qiu, G. Chen, J. Yang, Y. Zhao, X. Feng and K. Müllen, Boosting oxygen electrocatalytic activity of Fe–N–C catalysts by phosphorus incorporation, J. Am. Chem. Soc., 2023, 145, 3647–3655 CrossRef CAS PubMed.
- T. Li, L. Zhang, L. Zhang, J. Ke, T. Du, L. Zhang, Y. Cao, C. Yan and T. Qian, Tailoring the chemisorption manner of Fe d-band center with La2O3 for enhanced oxygen reduction in anion exchange membrane fuel cells, Adv. Funct. Mater., 2024, 34, 2309886 CrossRef CAS.
- J. Cai, Y. Xu, Y. Sun, H. Zhao, D. Ye, Y. Tang, C. Sun, L. Liu and J. Zhang, Regulating the coordination environment of Fe/Co-N/S-C to enhance ORR and OER bifunctional performance, Inorg. Chem. Front., 2023, 10, 1826–1837 RSC.
- L. Ran, Y. Xu, X. Zhu, S. Chen and X. Qiu, Mn single-atom tuning fe-n-c catalyst enables highly efficient and durable oxygen electrocatalysis and zinc-air batteries, ACS Nano, 2024, 18, 750–760 CrossRef CAS.
- Q. Lu, H. Wu, X. Zheng, Y. Chen, A. L. Rogach, X. Han, Y. Deng and W. Hu, Encapsulating cobalt nanoparticles in interconnected N-doped hollow carbon nanofibers with enriched Co/CoO@N-C moiety for enhanced oxygen electrocatalysis in Zn-air batteries, Adv. Sci., 2021, 8, 2101438 CrossRef CAS.
- X. Xie, Z. Zhai, W. Cao, J. Dong, Y. Li, Q. Hou, G. Du, J. Wang, L. Tian, J. Zhang, T. Zhang and L. Shang, Bifunctional ligand Co metal-organic framework derived heterostructured Co-based nanocomposites as oxygen electrocatalysts toward rechargeable zinc-air batteries, J. Colloid Interface Sci., 2024, 664, 319–328 CrossRef CAS PubMed.
- P. Yu, L. Wang, F. Sun, Y. Xie, X. Liu, J. Ma, X. Wang, C. Tian, J. Li and H. Fu, Co nanoislands rooted on Co-N-C nanosheets as efficient oxygen electrocatalyst for Zn–air batteries, Adv. Mater., 2019, 31, 1901666 CrossRef.
- P. Li, J. Wen, Y. Xiang, M. Li, Y. Zhao, S. Wang, J. Dou, Y. Li, H. Ma and L. Xu, Hierarchical mesoporous N-doped carbon as an efficient ORR/OER bifunctional electrocatalyst for rechargeable zinc–air battery, Inorg. Chem. Front., 2024, 11, 5345–5358 RSC.
- Y. Zhao, H.-C. Chen, X. Ma, J. Li, Q. Yuan, P. Zhang, M. Wang, J. Li, M. Li, S. Wang, H. Guo, R. Hu, K.-H. Tu, W. Zhu, X. Li, X. Yang and Y. Pan, Vacancy defects inductive effect of asymmetrically coordinated single-atom Fe-N3S1 active sites for robust electrocatalytic oxygen reduction with high turnover frequency and mass activity, Adv. Mater., 2024, 36, 2308243 CrossRef CAS PubMed.
- B. Zhang, J. Shan, W. Wang, P. Tsiakaras and Y. Li, Oxygen vacancy and core-shell heterojunction engineering of anemone-like CoP@CoOOH bifunctional electrocatalyst for efficient overall water splitting, Small, 2022, 18, 2106012 CrossRef CAS PubMed.
- F. Kong, Y. Huang, X. Yu, M. Li, K. Song, Q. Guo, X. Cui and J. Shi, Oxygen vacancy-mediated synthesis of inter-atomically ordered ultrafine Pt-alloy nanoparticles for enhanced fuel cell performance, J. Am. Chem. Soc., 2024, 146, 30078–30090 CrossRef CAS.
- M. Yang, S. Ding, X. Shu, W. Pan and J. Zhang, Hollow CoOx nanoparticle-embedded n-doped porous carbon as an efficient oxygen electrocatalyst for rechargeable zinc–air batteries, Mater. Chem. Front., 2022, 6, 3706–3715 RSC.
- K. Ding, J. Hu, J. Luo, L. Zhao, W. Jin, Y. Liu, Z. Wu, G. Zou, H. Hou and X. Ji, Robust electronic correlation of Co-CoN4 hybrid active sites for durable rechargeable Zn-air batteries, Adv. Funct. Mater., 2022, 32, 2207331 CrossRef CAS.
- J.-C. Li, Y. Meng, L. Zhang, G. Li, Z. Shi, P. X. Hou, C. Liu, H.-M. Cheng and M. Shao, Dual-phasic carbon with Co single atoms and nanoparticles as a bifunctional oxygen electrocatalyst for rechargeable Zn–air batteries, Adv. Funct. Mater., 2021, 31, 2103360 CrossRef CAS.
- H. Yang, S. Gao, D. Rao and X. Yan, Designing superior bifunctional electrocatalyst with high-purity pyrrole-type con4 and adjacent metallic cobalt sites for rechargeable Zn-air batteries, Energy Storage Mater., 2022, 46, 553–562 CrossRef.
- Z. Pei, H. Zhang, Z. P. Wu, X. F. Lu, D. Luan and X. W. Lou, Atomically dispersed Ni activates adjacent Ce sites for enhanced electrocatalytic oxygen evolution activity, Sci. Adv., 2023, 9, eadh1320 CrossRef CAS.
- Y. C. Zhang, C. Han, J. Gao, L. Pan, J. Wu, X.-D. Zhu and J. J. Zou, NiCo-based electrocatalysts for the alkaline oxygen evolution reaction: A review, ACS Catal., 2021, 11, 12485–12509 CrossRef CAS.
- X. Liu, F. Zhao, L. Jiao, T. Fang, Z. Zhao, X. Xiao, D. Li, K. Yi, R. Wang and X. Jia, Atomically dispersed Fe/N4 and Ni/N4 sites on separate-sides of porous carbon nanosheets with Janus structure for selective oxygen electrocatalysis, Small, 2023, 19, 2300289 CrossRef CAS PubMed.
- M. Wang, Z. Chen, Y. Song, Z. Hu, H. Song, S. Dong and D. Yuan, Architecting N-doped carbon nanotube-rich carbon nanofibers with biomimetic vine-leaf-whisker structure as robust bifunctional electrocatalysts for rechargeable Zn–air batteries, Inorg. Chem., 2024, 63, 4373–4384 CrossRef CAS PubMed.
- Z. Xu, J. Chen, T. Zhang, H. Lu, L. Yan, J. Ning and Y. Hu, Enhancing efficiency and durability of alkaline Zn-Co/air hybrid batteries with self-reconstructed Co/Co2P heterojunctions, Adv. Energy Mater., 2024, 2402839 CrossRef.
- L. Ye, W. Chen, Z. J. Jiang and Z. Jiang, Co/CoO heterojunction rich in oxygen vacancies introduced by O2 plasma embedded in mesoporous walls of carbon nanoboxes covered with carbon nanotubes for rechargeable zinc–air battery, Carbon Energy, 2024, 6, e457 CrossRef CAS.
- T. Hu, Z. Jiang, Z. Fu and Z. J. Jiang, A NiFe/NiSe2 heterojunction bifunctional catalyst rich in oxygen vacancies introduced using dielectric barrier discharge plasma for liquid and flexible all-solid-state rechargeable Zn–air batteries, J. Mater. Chem. A, 2022, 10, 8739–8750 RSC.
- W. Wu, R. Chen, S. Chen, Z. Wang and N. Cheng, Optimizing d-orbital electronic configuration via metal–metal oxide core-shell charge donation for boosting reversible oxygen electrocatalysis, Small, 2023, 19, 2300621 CrossRef CAS PubMed.
|
This journal is © the Partner Organisations 2025 |
Click here to see how this site uses Cookies. View our privacy policy here.