DOI:
10.1039/D4QI02381A
(Research Article)
Inorg. Chem. Front., 2025,
12, 301-310
A dual-purpose copper(I) coordination polymer for the construction of self-driven photoinduced C–H arylation systems†
Received
20th September 2024
, Accepted 20th November 2024
First published on 21st November 2024
Abstract
The simultaneous exploitation of the dual or multiple physical and chemical properties of a material is a promising strategy for developing high-tech intelligent complex systems. In this study, a copper(I) coordination polymer (CuI-CP, 2) was synthesized and utilized as a dual-purpose material to construct a self-powered photocatalytic system capable of significantly improving the power generation capabilities of triboelectric generators (TEGs) as a triboelectric layer and efficiently catalyzing the C–H arylation reaction as a photocatalyst. Compound 2 was achieved via a solvothermal method in the presence of ammonia and ethylenediamine. In contrast, only mixed-valence copper salts ([CuII(H2O)5][CuI3(CN)5]·H2O, 1) were obtained without ammonia and ethylenediamine. Comparative analysis revealed that the TEG based on 2 (2-TEG) showcased superior output performance compared to 1-TEG owing to the exceptional electron-donating ability of 2. Furthermore, under light-emitting diode (LED) irradiation powered by 2-TEG, 2 demonstrated remarkable catalytic activity and selectivity in the photoinduced C–H arylation of benzothiazole, far exceeding the performance of 1. This research highlights the potential of bifunctional material 2 with a distinctive structure, renowned for its outstanding energy harvesting and conversion capabilities as well as excellent photocatalytic performance, thereby facilitating the design objectives of multitasking in self-driven complex systems.
1. Introduction
Photocatalytic organic conversion has emerged as an environmentally benign alternative for producing value-added compounds.1–3 Achieving photocatalysts with high charge separation and fast charge-transfer kinetics is the main challenge of this process. Efforts are currently underway to enhance the performance of photocatalysts by designing new materials and modifying pristine catalysts.4–7 Metal–organic frameworks (MOFs) or coordination polymers (CPs), as a subclass of porous crystalline materials, hold significant promise in heterogeneous photocatalysis because of their high surface areas, adjustable and confined pore sizes, and tunable functionalities.1,8–14 Their tunable features enable the tailoring of MOFs or CPs based on specific requirements to accommodate different types of organic conversion reactions and environmental conditions. The high surface areas contribute to a sufficient number of active sites for contact with the reactants. Their confined porous structures provide transport pathways not only for the diffusion of selective substrates and products but also for the migration of photoinduced electrons to promote the separation of charge carriers.15,16 Therefore, MOFs or CPs are expected to be efficient photocatalysts through reasonable design at the molecular level, ensuring remarkable catalytic activity and selectivity.
On the other hand, photocatalysts generate electron–hole pairs upon exposure to light in order to facilitate chemical reactions. Typically, light is derived from solar or chemical energy, which is constrained by weather and environmental conditions.17–20 To address this limitation, a self-powered photocatalytic system that enables full utilization of the catalytic capabilities of photocatalysts without requiring an external energy input is needed.21–25 In this system, triboelectric generators (TEGs) based on the combined effects of triboelectrification and electrostatic induction, which can convert ambient mechanical energy into electrical energy, stand out as a promising option among a variety of self-powered devices owing to their universality in material selection, simplicity of design, and cost-effectiveness.26–30 Virtually, many materials can be used as friction materials for the fabrication of TEGs.31–35 Notably, MOFs or CPs exhibit exceptional characteristics for TEG applications owing to their aforementioned unique features.36–43 By carefully selecting metal centers and organic ligands or controlling the synthesis conditions, it becomes feasible to precisely fabricate diverse structures of MOFs or CPs with tailored characteristics suitable for high-output TEGs to meet the requirements of various photocatalytic organic conversion reactions.
Considering the unique advantages of MOFs or CPs in the construction of TEG devices and the photocatalysis field at the same time, it is conceivable to engineer a dual-purpose MOF or CP material with well-defined multitasking capabilities for high-performance MOF-based TEGs driving photoinduced MOF/CP-catalyzed organic conversion reactions. This approach, which incorporates two different properties of a material into a complex system to achieve multiple tasks, simplifies the preparation process and saves costs compared with using two materials with specific properties. It aligns with the objective of materials science to achieve maximum versatility using minimal resources, which is conducive to the development of high-tech intelligent systems. Herein, we present a self-powered photocatalytic system based on a bifunctional copper(I) CP (CuI-CP, 2), synthesized via a solvothermal method in the presence of ammonia and ethylenediamine. The material exhibited superior triboelectric properties as a friction layer in TEG assemblies and remarkable photocatalytic activity as a photocatalyst in photoinduced C–H arylation.
2. Experimental procedure
2.1. Materials
1,2,4,5-Tetra(2H-tetrazole-5-yl)-benzene (H4TTB) was synthesized according to a modified procedure from the literature.44 Copper(I) cyanide (CuCN, AR) was obtained from Sinopharm Chemical Reagent Co., Ltd (Shanghai, China). Benzothiazole (3, 98%) was provided by Saen Chemical Technology (Shanghai) Co., Ltd (Shanghai, China). 4-Iodotoluene (4a, 98%), 3-iodotoluene (4b, 98%), 2-iodotoluene (4c, 98%), iodobenzene (4d, 98%), 1-chloro-4-iodobenzene (4e, 98%), 2-iodonaphthalene (4f, 98%), m-iodobenzotrifluoride (4g, 98%), 4-iodobenzotrifluoride (4h, 98%), lithium tert-butoxide (LiOtBu, 99%), and N,N-dimethylglycine (Me2NCH2CO2H, 98%) were purchased from Anhui ZeSheng Technology Co., Ltd (Anhui, China). All reagents and solvents were commercially available and used as received without further purification.
2.2. Characterization
Complexes 1 and 2 were fully characterized by using various techniques, such as single-crystal X-ray diffraction (SCXRD), field-emission scanning electron microscopy (FE-SEM), X-ray photoelectron spectroscopy (XPS), and atomic force microscopy (AFM) (ESI†).
2.3. Syntheses
Synthesis of [CuII(H2O)5][CuI3(CN)5]·H2O (1).
H4TTB (0.017 g, 0.05 mmol) and CuCN (0.018 g, 0.2 mmol) were poured into a 15 mL glass vial containing a mixed solvent of 4 mL of EtOH and 4 mL of DMF, which was sonicated for 30 minutes and then heated at 80 °C for 3 days. Upon cooling, blue crystals were collected with a yield of 35% (based on Cu) after washing twice with distilled water and drying under vacuum. Anal. calcd for C10H24Cu8N10O12: C, 12.20%; H, 2.46%; N, 14.22%. Found: C, 12.15%; H, 2.43%; N, 14.17%. IR (KBr, cm−1): 3406 (w), 2803 (vw), 2713 (vw), 2363 (vw), 1631 (s), 1382 (w), 1349 (m), 1129 (m), 775 (vw), 619 (vw), 443 (vw).
Synthesis of (HEDA)[CuI4(TTB)(EDA)2(CN)] (CuI-CP, 2).
There are two routes to synthesize 2. The first method: a mixture of H4TTB (0.017 g, 0.05 mmol), CuCN (0.018 g, 0.2 mmol), EtOH (2 mL), DMF (2 mL), NH3·H2O (2 mL), and ethylenediamine (EDA, 2 mL) was placed in a 25 mL Teflon-lined stainless steel autoclave and vigorously stirred for 1 h. The container was sealed and kept at 160 °C for 3 days. After cooling to room temperature, light yellow crystals were obtained by centrifugation with a yield of 46% (based on Cu) after washing three times with EtOH and drying under vacuum. The second method: 4 mL of ammonia and 4 mL of ethylenediamine were added into a glass vial containing 1 along with its mother liquor, which was maintained at 80 °C for 2 days to obtain light yellow crystals with a yield of 50% (based on Cu). Anal. calcd for C17H27Cu4N23: C, 25.28%; H, 3.37%; N, 39.88%. Found: C, 25.22%; H, 3.34%; N, 39.80%. IR (KBr, cm−1): 3268 (vw), 2943 (vw), 2867 (vw), 2109 (m), 1653 (w), 1588 (m), 1415 (s), 1362 (m), 1139 (w), 1014 (s), 923 (m), 762 (w), 589 (s).
2.4. Assembly of TEGs based on 1 and 2
In a 50 mL round-bottom flask, PVDF (3.75 g), DMAC (N,N-dimethylacetamide, 8.5 g), and acetone (12.75 g) were stirred at 60 °C for 30 minutes. The mixed solution was then cooled naturally to achieve uniform dissolution. Subsequently, the homogeneous solution was spin-coated onto a Kapton (polyimide) film to produce a PVDF membrane, which was further adhered to the adhesive side of Cu tape to fabricate a negative electrode. After grinding crystals 1 or 2 into fine powders, they were uniformly fixed onto the adhesive side of Cu tape, with excess powder being blown away using an air gun.
Finally, copper wires were firmly attached onto the other side of each copper tape to ensure good electrical conductivity. The spin-coated PVDF film and 1 or 2 were used as the negative and positive friction materials, respectively. They were combined to fabricate 1/2-TEG devices, working in vertical contact-separation mode with an effective contact area of 5 × 5 cm2.
2.5. Procedure for the photoinduced copper-catalyzed C–H arylation reaction using the 2-TEG device
A mixture solution of benzothiazole (3, 0.5 mmol), iodoarene (4a–h, 2.5 mmol), catalyst (CuI/1/2, 15 mol%), N,N-dimethylglycine (30 mol%), LiOtBu (1.5 mmol), and Et2O (3 mL) under a N2 atmosphere was sealed tightly and stirred under irradiation of 14 blue LEDs (1 W) driven by the 2-TEG device. The reaction temperature was maintained at 23–25 °C. After approximately 12 hours, the reaction mixture was cooled, filtered, washed with Et2O, and extracted with ethyl acetate and water. The organic phase was separated and concentrated under reduced pressure. The residue was purified by column chromatography on silica gel to obtain pure products 5a–h.
3. Results and discussion
3.1 Structures and characterization of 1 and 2
The direct reaction of CuCN with the H4TTB (H4TTB = 1,2,4,5-tetra(2H-tetrazole-5-yl)-benzene) ligand in a mixed solution of DMF/EtOH produced blue single crystals 1 (Fig. 1a). Unexpectedly, 1 is a mixed-valence copper salt without the participation of the organic ligand H4TTB. Single-crystal X-ray diffraction (SCXRD) analysis unambiguously showed that 1 possesses a three-dimensional (3D) anionic framework {[CuI3(CN)5]2−}n composed of cyanogen and CuI ions with [CuII(H2O)5]2+ countercations and free water molecules as guest molecules in the cavities (Fig. 1b and c). The binding energies of 1 for Cu 2p3/2 and 2p1/2 at 932.35, 934.84, 952.49, and 954.94 eV, as well as the shake-up satellite signals at 941.61, 943.18, and 944.29 eV in the X-ray photoelectron spectroscopy (XPS) spectra (Fig. S1† and Fig. 2a), reveal the coexistence of CuI and CuII centers.
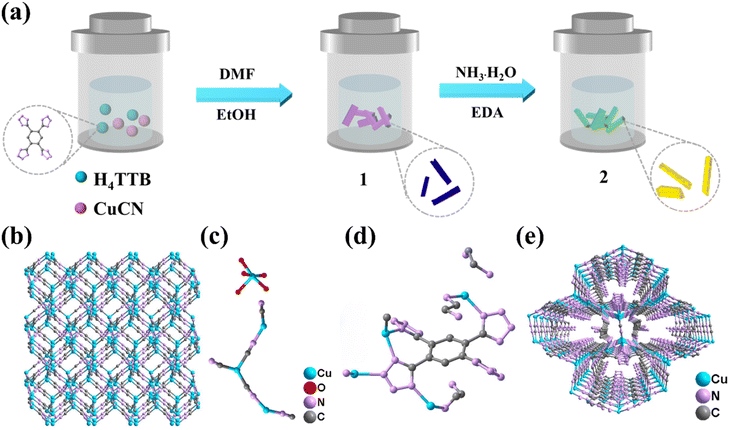 |
| Fig. 1 (a) Schematic diagram of the synthesis procedure of compounds 1 and 2. (b) The 3D anionic framework of 1. (c) The asymmetric unit of 1. (d) The linkage between Cu+ and the TTB4− ligand in 2. (e) The 3D framework of 2. All hydrogen atoms, free solvent molecules, and countercations are omitted for clarity. | |
 |
| Fig. 2 (a) XPS spectra of 1. (b) XPS spectra of 2. (c) HOMO–LUMO energy levels of 1 and 2. (d) Kelvin probe force microscopy surface potentials of H4TTB, 1 and 2. | |
Surprisingly, the addition of ammonia and ethylenediamine into the mixture of 1 together with their mother solution resulted in the rapid dissolution of 1, and light-yellow blocky crystals of 2 were obtained after being maintained at 80 °C for 48 hours (Fig. 1a). In addition to this approach, the one-pot method was also successfully used to synthesize 2. The formula of 2 was determined as {(HEDA)[CuI4(TTB)(EDA)2(CN)]}n by combining various characterization results (including SCXRD and fourier transform infrared spectroscopy (FT-IR) (Fig. S2†), thermogravimetric analysis (TGA) (Fig. S3†), elemental analysis (EA), XPS (Fig. S4†), etc.) with charge-balance consideration. The phase purity of the bulk crystals was verified by powder X-ray diffraction (PXRD) experiments (Fig. S5†). Unlike mixed-valence CuI/CuII salts 1, there were four crystallographically independent Cu+ ions, one fully deprotonated TTB4− ligand, two coordinated ethylenediamine molecules, one coordinated CN− ion, and one free protonated ethylenediamine in the asymmetric unit of 2 (Fig. 1d). Each Cu+ ion was in a distorted four-coordinated tetrahedral coordination geometry completed by three N atoms from the tetrazole rings of the TTB4− ligands and one N/C atom from the coordinated ethylenediamine molecule or the CN− anion. Two of the four tetrazole rings in the TTB4− ligand were two-coordinated with two CuI ions, and the other two were four-coordinated to connect four CuI ions. Among them, two CuI ions were shared by two tetrazole rings in close proximity. Therefore, each TTB4− ligand linked ten CuI ions through twelve N atoms in four tetrazole rings to form a two-dimensional (2D) layered structure, which was further expanded to a 3D framework by the coordination of CN− anions with CuI ions between the two adjacent layers (Fig. 1e). The peaks at 932.58 and 952.54 eV in the XPS spectrum (Fig. S4† and Fig. 2b), corresponding to Cu 2p3/2 and 2p1/2, respectively, confirmed that copper ions existed in the unique form of Cu+.
The energy bandgap values obtained for 1 and 2 from UV-visible diffuse reflectance spectroscopy (UV−Vis DRS) by Tauc plot analysis were 3.21 and 2.62 eV, respectively (Fig. S6 and S7†).45 Compared to 1, the smaller bandgap of 2 manifested that less energy was needed for the initiation of photocatalytic processes, enabling it to be a more efficient photocatalyst. Mott–Schottky measurements were performed to illustrate the n-type semiconductor nature of 2 (Fig. S8†). However, the energy of the highest occupied molecular orbital (HOMO) for 2 was 2.41 V, which was higher than that for 1 (3.01 V), demonstrating that the valence-band electrons in 2 were much more active than those in 1 (Fig. 2c).
The instantaneous photocurrent response under visible light irradiation was higher over 2 than that of 1 (Fig. S9†), suggesting that 2 promotes electron–hole separation more effectively, which results in the generation of more free photogenerated carriers.46 Furthermore, the transport ability of the photogenerated charge carriers in the two samples was evaluated using electrochemical impedance spectroscopy (EIS). It can be seen clearly from the typical Nyquist plots that both 1 and 2 display one dominant semicircle, and the diameter of 2 is much smaller than that of 1, revealing a smaller charge-transfer resistance for 2 than for 1 (Fig. S10†).47 This implies that more photogenerated electrons are transferred to the surface within a certain amount of time. Efficient charge separation and improved charge transport were achieved simultaneously within 2, thereby enhancing its overall photocatalytic activity.
3.2 The output performance of TEGs based on 1 and 2
Two materials with different electron affinities produce a potential difference during the process of contact and separation, such that electrons flow back and forth between the electrodes and, in turn, generate the corresponding electrical signals. However, the composition (types and distribution density of elements and groups) and structure of the material have a significant impact on the electron affinity.48 Therefore, bulk crystals 1 and 2 were ground and coated on Cu tape, and their surface potentials were characterized by Kelvin probe force microscopy (KPFM) to confirm the difference in electron affinity (Fig. 2d and S11†). The results showed that 2 induced a higher average surface potential than both 1 (0.117 V for 1 and 0.410 V for 2) and the organic ligand H4TTB, demonstrating that 2 has a better electron-donating ability and is more suitable as a triboelectric positive layer to improve the TEG output performance.
To explore the effect of the structure and composition of the CPs on the performance of the assembled TEGs, 1/2-TEGs designed in vertical contact-separation working mode were fabricated using 1/2 powders and a polyvinylidene fluoride (PVDF) film as positive and negative triboelectric materials, respectively (Fig. 3a). Cu tapes served as electrodes, and the Kapton film was used as the substrate and triboelectrification charge storage layer.49 The working mechanism is illustrated in Fig. 3b. It should be noted that the TEG performance can be affected by factors such as surface roughness and thickness. To reduce the impact of these factors, similar particle sizes of 1 and 2 (less than 4 μm) were obtained by controlling the grinding time (Fig. S12†). In addition, the same dose of powders was used to prepare the friction layers, and the samples were compacted with a glass rod such that they were evenly and firmly coated on the Cu tape. Finally, frictional electrodes with similar thicknesses and surface roughness were obtained for further research (Fig. S13 and S14†).
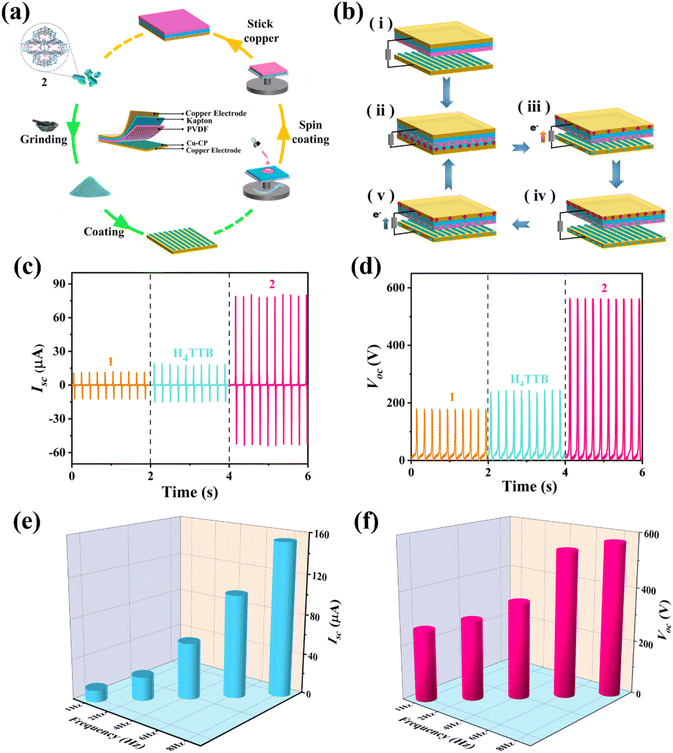 |
| Fig. 3 (a) The schematic diagram of the fabrication process for the 2-based TEG. (b) The working principle of the 2-based TEG in vertical contact-separation mode. (c) Isc and (d) Voc of 1-TEG, H4TTB-TEG, and 2-TEG at 5 Hz. (e) Isc and (f) Voc of the TEGs based on 2 under different operating frequencies. | |
Driven by a linear motor to simulate various mechanical energies in the real environment, the 1 or 2 layers periodically contacted and separated from the PVDF film. Through the coupling effect of triboelectrification and electrostatic induction, electrical signals can be generated and collected by connecting an external circuit. As shown in Fig. 3c and d, the TEG based on 2 outputted much stronger current and voltage signals than those based on 1. The short-circuit current (Isc), the open-circuit voltage (Voc) and the transferred charges (Qsc) of 2-TEG reached up to 80.7 μA, 563.6 V and 360 nC under a force of 3.5 N at an operating frequency of 5 Hz, respectively, resulting in a 530.5%, 212.1% and 414.3% increase, respectively, compared to those of 1-TEG (12.8 μA, 180.6 V and 70 nC, respectively) under the same conditions (Fig. S15†). These results indicated the strong electron-donating ability of 2 as a triboelectric layer, which was consistent with our hypothesis.
The variation in the TEG output was tested in terms of the operating frequencies in the range of 1–8 Hz. Obviously, both the Isc and Voc of 2-TEG displayed distinct enhancements as the frequency increased (Fig. 3e and f). This phenomenon is consistent with the reported studies on nano-TEGs by employing the sine wave.50–52 The optimal output showed an Isc of 153.7 μA, a Voc of 569.8 V, and a charge density of 144.6 μC m−2 at a frequency of 8 Hz. The change in current was measured under various external resistances (Fig. S16†). As the connected external resistance increased, the current of 2-TEG decreased. The instantaneous power density (P) was calculated using the formula P = I2R/A (where I represents the output current under each connected resistance, R denotes the value of the resistance, and A signifies the area of the friction surface),53 and the maximum peak was 3120.5 mW m−2 under an external load of 50 MΩ. The 2-TEG device shows a higher power density as compared to the CP/MOF based nano-TEGs reported in the literature (Table S3†).37,54–56
To examine the durability and sustainability of the assembled 2-TEG, long-term durability experiments were conducted at a frequency of 5 Hz in vertical contact-separation mode (Fig. S17 and S18†). 2-TEG demonstrated distinguished stability, withstanding up to 50
000 periodic cycles without any decrease in performance. Furthermore, the field-emission scanning electron microscopy (FE-SEM) images and energy dispersive spectroscopy (EDS) mapping of friction layer 2 coated on the Cu tape remained unchanged before and after the test, confirming the stability of the friction electrode (Fig. S19 and S20†).
3.3 Self-driven photoinduced C–H arylation systems
From the structural and electrical characterization studies discussed above, it can be inferred that the as-prepared 2 is not only a triboelectric material for assembling a high-output performance TEG, but also a potential excellent photocatalyst for catalyzing photoinduced reactions. Aryl compounds containing heteroaryl moieties exhibit distinctive chemical and physical properties, finding widespread applications in medicine, materials science, and other disciplines. Photoinduced copper-catalyzed C–H activation has been established as a highly efficient method for cross-coupling reactions between various heteroarenes and aryl halides under exceptionally mild reaction conditions at ambient temperature.57,58 Subsequently, 2-TEG as a power source was directly connected to 14 blue commercial light-emitting diodes (LEDs, 460–465 nm) without any storage or power units (Fig. S21 and Video S1†), and the light emitted was used to induce copper-catalyzed C–H functionalization under a N2 atmosphere at room temperature (Fig. 4a). Our studies were initiated by exploring the optimal reaction conditions with benzothiazole (3) and 4-iodotoluene (4a) as model substrates using the photoinduced copper(I) catalysts (Table S4†). Among the conditions screened, 2 was much more effective for this reaction than the as-obtained mixed-valence copper salt 1 and copper iodide (CuI), both with and without the aid of an organic ligand (N,N-dimethylglycine) (Table S4, entries 1–4†). Preliminary experiments on the effect of catalyst loading on the catalytic activity showed that 5a was obtained in high yield within 10 h in the presence of 15 mol% catalyst (Table S4, entries 5–13†). Controlled experiments revealed the need for copper catalysts (Table S4, entry 14†) and unambiguously revealed the photocatalytic characteristics of the C–H activation process (Table S4, entry 15†).
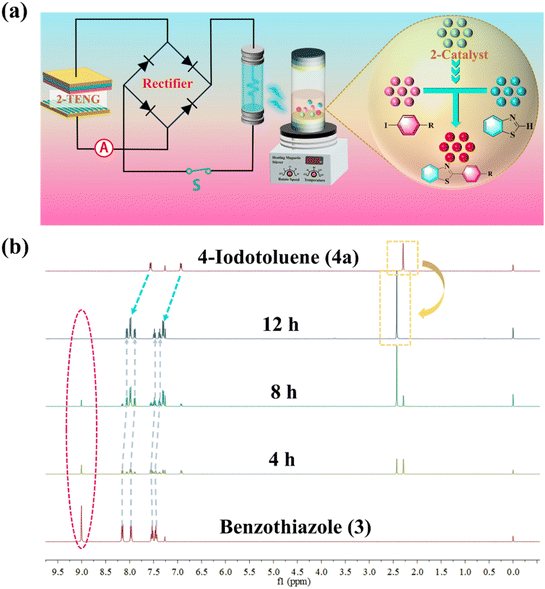 |
| Fig. 4 (a) Transformation of 3 to 4 under a visible light source driven by 2-TEG. (b) 1H NMR spectra of 3 to 5a after visible light irradiation for 0, 4, 8, and 12 h, respectively. | |
With the optimal reaction conditions in hand, the self-powered photocatalytic azole arylation process was monitored using 1H NMR spectroscopy. It was observed that the hydrogen signal for the thiazole group (δ = 9.0 ppm) in 3 gradually weakened and eventually disappeared over time, indicating the smooth progress of the photocatalytic site-selective C–H arylation (Fig. 4b). Additionally, the phenyl resonances in 3 experienced a slight upfield shift, and all the signals associated with 4a moved downfield. Substrates (3 and 4a) and product 5a coexisted for less than 10 hours under light irradiation; however, beyond this duration, the characteristic peaks of the substrates vanished immediately, confirming the completion of the C–H functionalization reaction of 3.
Finally, efforts were devoted to probing the generality of the self-powered photoinduced C–H arylation of 3 by extending the substrate scope with respect to the variety of substituted aryl iodides. Table 1 shows that catalyst 2 effectively executed the C–H arylation process with aryl iodides (4a–4h) and generated the corresponding arylated benzothiazoles (5a–5h) in fair to moderate yields (70–90%). Aryl iodides with methyl substituents as electron-donating groups were well tolerated at all substituted positions (para- (4a), meta- (4b) and ortho- (4c)), as well as without substituents (4d) (Table 1, entries 1–12). Interestingly, electron-poor aryl iodides, such as 4e–4h bearing chloro-, iodo- and trifluoromethyl groups, respectively (Table 1, entries 13–24), also performed well. These results demonstrated the generality of the reactions. After the catalysis was completed, catalyst 2 was filtered out for further XPS characterization (Fig. S22†), which indicated that Cu still existed in the form of a single valence state, thereby confirming its stability during the catalytic process.
Table 1 Self-powered photocatalytic C–H arylation systems driven by the 2-TEG devicea
Conclusions
In summary, a self-powered photocatalytic system was assembled for C–H arylation by integrating a CP-based TEG with a CP-catalyzed reaction chamber. CuI-CP (2) was synthesized using both stepwise and direct synthesis methods in the presence of ammonia and ethylenediamine. It exhibited a comparatively narrow bandgap and a high surface potential, making it an excellent triboelectric positive material for fabricating high-output TEGs. Electrical characterization of the CP-based TEGs demonstrated an Isc of 80.7 μA and a Voc of 563.6 V at 5 Hz, surpassing all other TEGs based on the organic ligand H4TTB and intermediate products (mixed-valence CuI/CuII salts 1). In addition, 2 served as a highly efficient catalyst for the photocatalytic reaction, as evidenced by the photocurrent response and EIS results. Consequently, the fabricated 2-TEG successfully drove the C–H arylation of benzothiazole under the catalysis of 2 by illuminating lamp beads. This study emphasizes both the triboelectric and catalytic properties of CPs and leverages their bifunctionality to construct complex self-driven systems. These findings not only provide insights into designing complex systems with multiple tasks, but also will arouse enthusiasm for designing bi-/multifunctional CPs for self-powered systems.
Author contributions
Yue Zhang: investigation, methodology, writing – original draft, and software. Ying-Ying Zhang: conceptualization, supervision, writing – original draft, and software. Shuo Li: methodology, investigation, and software. Fei Wang: methodology and data curation. Yuanmeng Tao: methodology and validation. Jiaxing Cui: software. Chao Huang: writing – review & editing, supervision, funding acquisition, and resources. Liwei Mi: supervision and resources.
Data availability
The data supporting this article have been included as part of the ESI.†
Conflicts of interest
There are no conflicts to declare.
Acknowledgements
This work was supported by the National Natural Science Foundation of China (No. 21601212 and 21701201), the Program for Science & Technology Innovation Talents in Universities of Henan Province (22HASTIT030), the Natural Science Foundation of Henan (222300420593), the Program for Science & Technology Innovation Talents in Zhongyuan University of Technology (K2023YXRC02), the Strength Enhancement Plan of Superior Disciplines and the Graduate Education Quality Improvement Project of Zhongyuan University of Technology (GG202213 and JG202321).
References
- J. Chen, R. Abazari, K. A. Adegoke, N. W. Maxakato, O. S. Bello, M. Tahir, S. Tasleem, S. Sanati, A. M. Kirillov and Y. Zhou, Metal-organic frameworks and derived materials as photocatalysts for water splitting and carbon dioxide reduction, Coord. Chem. Rev., 2022, 469, 214664 CrossRef CAS.
- L. Fu, R. Zhang, J. Yang, J. Shi, H.-Y. Jiang and J. Tang, Highly Selective Conversion of CH4 to High Value-Added C1 Oxygenates over Pd Loaded ZnTi-LDH, Adv. Energy Mater., 2023, 13, 2301118 CrossRef CAS.
- T. Han, X. Cao, H.-C. Chen, J. Ma, Y. Yu, Y. Li, W. Xu, K. Sun, A. Huang, Z. Chen, C. Chen, H. Zhang, B. Ye, Q. Peng and Y. Li, Photosynthesis of Benzonitriles on BiOBr Nanosheets Promoted by Vacancy Associates, Angew. Chem., Int. Ed., 2023, 62, e202313325 CrossRef CAS PubMed.
- D. Chen, H. Zhang, Y. Li, Y. Pang, Z. Yin, H. Sun, L.-C. Zhang, S. Wang, M. Saunders, E. Barker and G. Jia, Photocatalysis: Spontaneous Formation of Noble- and Heavy-Metal-Free Alloyed Semiconductor Quantum Rods for Efficient Photocatalysis, Adv. Mater., 2018, 30, 1870296 CrossRef.
- X. Tian, Y. Guo, W. An, Y.-L. Ren, Y. Qin, C. Niu and X. Zheng, Coupling photocatalytic water oxidation with reductive transformations of organic molecules, Nat. Commun., 2022, 13, 6186 CrossRef CAS PubMed.
- B. Cai, P. Huang, Y. Fang and H. Tian, Recyclable and Stable Porphyrin-Based Self-Assemblies by Electrostatic Force for Efficient Photocatalytic Organic Transformation, Adv. Sci., 2024, 11, 2308469 CrossRef CAS PubMed.
- H. Wang, Nanostructure@metal-organic frameworks (MOFs) for catalytic carbon dioxide (CO2) conversion in photocatalysis, electrocatalysis, and thermal catalysis, Nano Res., 2022, 15, 2834–2854 CrossRef CAS.
- L. Jiao, Y. Wang, H.-L. Jiang and Q. Xu, Metal–Organic Frameworks as Platforms for Catalytic Applications, Adv. Mater., 2018, 30, 1703663 CrossRef PubMed.
- R. Li, W. Zhang and K. Zhou, Metal-Organic-Framework-Based Catalysts for Photoreduction of CO2, Adv. Mater., 2018, 30, 1705512 CrossRef PubMed.
- Z. Wang, X. Yue and Q. Xiang, MOFs-based S-scheme heterojunction photocatalysts, Coord. Chem. Rev., 2024, 504, 215674 CrossRef CAS.
- A. Dhakshinamoorthy, Z. Li and H. Garcia, Catalysis and photocatalysis by metal organic frameworks, Chem. Soc. Rev., 2018, 47, 8134–8172 RSC.
- T. Le Huec, A. López-Francés, I. A. Lázaro, S. Navalón, H. G. Baldoví and M. Giménez-Marqués, Heteroepitaxial MOF-on-MOF Photocatalyst for Solar-Driven Water Splitting, ACS Nano, 2024, 18, 20201–20212 CrossRef CAS PubMed.
- T. Zhang and W. Lin, Metal-organic frameworks for artificial photosynthesis and photocatalysis, Chem. Soc. Rev., 2014, 43, 5982–5993 RSC.
- Y. Chen, P. Li, J. Zhou, C. T. Buru, L. Đorđević, P. Li, X. Zhang, M. M. Cetin, J. F. Stoddart, S. I. Stupp, M. R. Wasielewski and O. K. Farha, Integration of Enzymes and Photosensitizers in a Hierarchical Mesoporous Metal-Organic Framework for Light-Driven CO2 Reduction, J. Am. Chem. Soc., 2020, 142, 1768–1773 CrossRef CAS PubMed.
- P. Du, J. Schneider, P. Jarosz and R. Eisenberg, Photocatalytic Generation of Hydrogen from Water Using a Platinum(II) Terpyridyl Acetylide Chromophore, J. Am. Chem. Soc., 2006, 128, 7726–7727 CrossRef CAS PubMed.
- Z. Jin and H. Yang, Exploration of Zr-Metal-Organic Framework as Efficient Photocatalyst for Hydrogen Production, Nanoscale Res. Lett., 2017, 12, 539 CrossRef PubMed.
- Q. Zhou, Y. Guo, Z. Ye, Y. Fu, Y. Guo and Y. Zhu, Carbon nitride photocatalyst with internal electric field induced photogenerated carriers spatial enrichment for enhanced photocatalytic water splitting, Mater. Today, 2022, 58, 100–109 CrossRef CAS.
- K. Mase, M. Yoneda, Y. Yamada and S. Fukuzumi, Efficient Photocatalytic Production of Hydrogen Peroxide from Water and Dioxygen with Bismuth Vanadate and a Cobalt(II) Chlorin Complex, ACS Energy Lett., 2016, 1, 913–919 CrossRef CAS.
- C.-f. Li, W.-g. Pan, Z.-r. Zhang, T. Wu and R.-t. Guo, Recent Progress of Single-Atom Photocatalysts Applied in Energy Conversion and Environmental Protection, Small, 2023, 19, 2300460 CrossRef CAS PubMed.
- G. Z. S. Ling, S.-F. Ng and W.-J. Ong, Tailor-Engineered 2D Cocatalysts: Harnessing Electron–Hole Redox Center of 2D g-C3N4 Photocatalysts toward Solar-to-Chemical Conversion and Environmental Purification, Adv. Funct. Mater., 2022, 32, 2111875 CrossRef CAS.
- C. Huang, Q. Zhang, Y. Zhang, F. Wang, Y.-Y. Zhang, M. Qiu, Y. Zhang and L. Zhai, Self-driven electrochemical system using solvent-regulated structural diversity of cadmium(II) metal–organic frameworks, J. Colloid Interface Sci., 2024, 662, 953–961 CrossRef CAS PubMed.
- Q. Fu, Y. Liu, J. Mo, Y. Lu, C. Cai, Z. Zhao, S. Wang and S. Nie, Improved Capture and Removal Efficiency of Gaseous Acetaldehyde by a Self-Powered Photocatalytic System with an External Electric Field, ACS Nano, 2021, 15, 10577–10586 CrossRef CAS PubMed.
- T. Lu, Y. Zhang, Z. Wang, S. Li, L. Zheng and H. Li, Self-powered organic pollutants degradation in wastewater by photocatalytic ozonation based on triboelectric nanogenerator, Nano Energy, 2024, 127, 109712 CrossRef CAS.
- T. Sun, Q. Zheng, H. Luo, J. Long, L. Zheng and H. Li, Self-powered exhaust gas purification by negative ions and photoelectric catalysis based on triboelectric nanogenerator, Nano Energy, 2023, 115, 108677 CrossRef CAS.
- J. Zhou, N. Zhou, M. Liu, H. Tan, Z. Wang, X. Zhang and Z. Su, NiTiO3/Bi2O3/MoS2 double Z-type heterojunction catalysts realize dual-function applications of photocatalytic fuel cells and lactic acid sensing, Appl. Surf. Sci., 2024, 649, 159095 CrossRef CAS.
- C. Wu, A. C. Wang, W. Ding, H. Guo and Z. L. Wang, Triboelectric Nanogenerator: A Foundation of the Energy for the New Era, Adv. Energy Mater., 2019, 9, 1802906 CrossRef.
- P. Lu, X. Liao, X. Guo, C. Cai, Y. Liu, M. Chi, G. Du, Z. Wei, X. Meng and S. Nie, Gel-Based Triboelectric Nanogenerators for Flexible Sensing: Principles, Properties, and Applications, Nano-Micro Lett., 2024, 16, 206 CrossRef CAS PubMed.
- Z. L. Wang, J. Chen and L. Lin, Progress in triboelectric nanogenerators as a new energy technology and self-powered sensors, Energy Environ. Sci., 2015, 8, 2250–2282 RSC.
- X. Cui, J. Nie and Y. Zhang, Recent advances in high charge density triboelectric nanogenerators, Int. J. Extreme Manuf., 2024, 6, 042001 CrossRef CAS.
- D. W. Kim, J. H. Lee, J. K. Kim and U. Jeong, Material aspects of triboelectric energy generation and sensors, NPG Asia Mater., 2020, 12, 6 CrossRef.
- Z. Zhang, W. Gong, Z. Bai, D. Wang, Y. Xu, Z. Li, J. Guo and L.-S. Turng, Oxygen-Rich Polymers as Highly Effective Positive Tribomaterials for Mechanical Energy Harvesting, ACS Nano, 2019, 13, 12787–12797 CrossRef CAS PubMed.
- H. Zhang, X. Gong and X. Li, Material selection and performance optimization strategies for a wearable friction nanogenerator (W-TENG), J. Mater. Chem. A, 2023, 11, 24454–24481 RSC.
- R. Walden, I. Aazem, A. Babu and S. C. Pillai, Textile-Triboelectric nanogenerators (T-TENGs) for wearable energy harvesting devices, Chem. Eng. J., 2023, 451, 138741 CrossRef CAS.
- S. Liu, W. Tong, C. Gao, Y. Liu, X. Li and Y. Zhang, Environmentally friendly natural materials for triboelectric nanogenerators: a review, J. Mater. Chem. A, 2023, 11, 9270–9299 RSC.
- Z. L. Wang, Triboelectric Nanogenerators as New Energy Technology for Self-Powered Systems and as Active Mechanical and Chemical Sensors, ACS Nano, 2013, 7, 9533–9557 CrossRef CAS PubMed.
- N. P K and A. Chandrasekhar, Marriage between metal-organic frameworks/covalent-organic frameworks and triboelectric nanogenerator for energy harvesting-A review, Mater. Today Energy, 2023, 37, 101393 CrossRef CAS.
- G. Khandelwal, N. P. M. J. Raj and S.-J. Kim, Zeolitic Imidazole Framework: Metal-Organic Framework Subfamily Members for Triboelectric Nanogenerators, Adv. Funct. Mater., 2020, 30, 1910162 CrossRef CAS.
- S. Hajra, M. Sahu, A. M. Padhan, I. S. Lee, D. K. Yi, P. Alagarsamy, S. S. Nanda and H. J. Kim, A Green Metal-Organic Framework-Cyclodextrin MOF: A Novel Multifunctional Material Based Triboelectric Nanogenerator for Highly Efficient Mechanical Energy Harvesting, Adv. Funct. Mater., 2021, 31, 2101829 CrossRef CAS.
- Q. Zhang, C. Huang, Y. Zhang, S. Liu, D. Zhang, P. Li, F. Wang, D. Wang and Y.-Y. Zhang, Self-powered photocatalytic system fabricated by bifunctional coordination polymers, Chem. Eng. J., 2024, 482, 148854 CrossRef CAS.
- R. Wen, R. Feng, B. Zhao, J. Song, L. Fan and J. Zhai, Controllable design of high-efficiency triboelectric materials by functionalized metal-organic frameworks with a large electron-withdrawing functional group, Nano Res., 2022, 15, 9386–9391 CrossRef CAS.
- R. K. Rajaboina, U. K. Khanapuram, V. Vivekananthan, G. Khandelwal, S. Potu, A. Babu, N. Madathil, M. Velpula and P. Kodali, Crystalline Porous Material-Based Nanogenerators: Recent Progress, Applications, Challenges, and Opportunities, Small, 2024, 20, 2306209 CrossRef CAS PubMed.
- Y.-M. Wang, X. Zhang, Y. Ran, C. Liu, D. Wang, G. Mao, X. Jiang, S. Wang, X.-B. Yin and R. Yang, Advances in Metal-Organic Framework-Based Triboelectric Nanogenerators, ACS Mater. Lett., 2024, 6, 3883–3898 CrossRef CAS.
- C. Huang, S. Zhang, S. Liu, Q. Zhang, Y.-Y. Zhang and L. Mi, Triboelectric Nanogenerators Assembled by Cobalt(II) Coordination Polymer Incorporated Composite Films and their Application for Self-Powered Anticorrosion, Chem. – Eur. J., 2023, 29, e202300528 CrossRef CAS PubMed.
- H. Lee, S. H. Jung, W. S. Han, J. H. Moon, S. Kang, J. Y. Lee, J. H. Jung and S. Shinkai, Inside Cover: A Chromo-Fluorogenic Tetrazole-Based CoBr2 Coordination Polymer Gel as a Highly Sensitive and Selective Chemosensor for Volatile Gases Containing Chloride, Chem. – Eur. J., 2011, 17, 2790–2790 CrossRef.
- H.-Q. Xu, J. Hu, D. Wang, Z. Li, Q. Zhang, Y. Luo, S.-H. Yu and H.-L. Jiang, Visible-Light Photoreduction of CO2 in a Metal-Organic Framework: Boosting Electron-Hole Separation via Electron Trap States, J. Am. Chem. Soc., 2015, 137, 13440–13443 CrossRef CAS PubMed.
- Y. An, Y. Liu, P. An, J. Dong, B. Xu, Y. Dai, X. Qin, X. Zhang, M.-H. Whangbo and B. Huang, NiII Coordination to an Al-Based Metal-Organic Framework Made from 2-Aminoterephthalate for Photocatalytic Overall Water Splitting, Angew. Chem., Int. Ed., 2017, 56, 3036–3040 CrossRef CAS PubMed.
- L. Liu, H. Meng, Y. Chai, X. Chen, J. Xu, X. Liu, W. Liu, D. M. Guldi and Y. Zhu, Enhancing Built-in Electric Fields for Efficient Photocatalytic Hydrogen Evolution by Encapsulating C60 Fullerene into Zirconium-Based Metal-Organic Frameworks, Angew. Chem., Int. Ed., 2023, 62, e202217897 CrossRef CAS PubMed.
- X. Li, C. Jiang, Y. Yao, Q. Zhang, S. Dai, Y. Ying and J. Ping, Growth-Controllable Triboelectric Nanogenerator Based on Surface-Attached Metal-Organic Framework Layer on Living Leaf, Small, 2021, 17, 2103430 CrossRef CAS PubMed.
- Y. Feng, Y. Zheng, G. Zhang, D. Wang, F. Zhou and W. Liu, A new protocol toward high output TENG with polyimide as charge storage layer, Nano Energy, 2017, 38, 467–476 CrossRef CAS.
- C. Liu, N. Zhang, J. Li, L. Dong, T. Wang, Z. Wang, G. Wang, X. Zhou and J. Zhang, Harvesting ultralow frequency (< 1 Hz) mechanical energy using triboelectric nanogenerator, Nano Energy, 2019, 65, 104011 CrossRef CAS.
- Y. Dai, X. Zhong, T. Xu, Y. Li, Y. Xiong and S. Zhang, High-Performance Triboelectric Nanogenerator Based on Electrospun Polyvinylidene Fluoride-Graphene Oxide Nanosheet Composite Nanofibers, Energy Technol., 2023, 11, 2300426 CrossRef CAS.
- Z. Shao, J. Chen, K. Gao, Q. Xie, X. Xue, S. Zhou, C. Huang, L. Mi and H. Hou, A Double-Helix Metal-Chain Metal-Organic Framework as a High-Output Triboelectric Nanogenerator Material for Self-Powered Anticorrosion, Angew. Chem., Int. Ed., 2022, 61, e202208994 CrossRef CAS PubMed.
- H. Yoo, M. Mahato, J.-S. Kim, S. Oh, M. Garai, V. H. Nguyen, A. K. Taseer, M.-J. Lee and I.-K. Oh, Janus CoMOF-SEBS Membrane for Bifunctional Dielectric Layer in Triboelectric Nanogenerators, Adv. Sci., 2024, 11, 2307656 CrossRef CAS PubMed.
- P. Zhu, Z. Ullah, S. Zheng, Z. Yang, S. Yu, S. Zhu, L. Liu, A. He, C. Wang and Q. Li, Ultrahigh current output from triboelectric nanogenerators based on UIO-66 materials for electrochemical cathodic protection, Nano Energy, 2023, 108, 108195 CrossRef CAS.
- G. Khandelwal, A. Chandrasekhar, N. P. M. J. Raj and S. J. Kim, Metal–Organic Framework: A Novel Material for Triboelectric Nanogenerator–Based Self–Powered Sensors and Systems, Adv. Funct. Mater., 2019, 9, 1803581 Search PubMed.
- S. Barsiwal, A. Babu, U. K. Khanapuram, S. Potu, N. Madathil, R. K. Rajaboina, S. Mishra, H. Divi, P. Kodali, R. Nagapuri and T. Chinthakuntla, ZIF-67-Metal-Organic-Framework-Based Triboelectric Nanogenerator for Self-Powered Devices, Nanoenergy Adv., 2022, 2, 291–302 CrossRef.
- F. Yang, J. Koeller and L. Ackermann, Photoinduced Copper-Catalyzed C-H Arylation at Room Temperature, Angew. Chem., Int. Ed., 2016, 55, 4759–4762 CrossRef CAS PubMed.
- X. Ma and G. Zhang, Visible Light-Induced Copper-Catalyzed C-H Arylation of Benzoxazoles†, Chin. J. Chem., 2020, 38, 1299–1303 CrossRef CAS.
|
This journal is © the Partner Organisations 2025 |
Click here to see how this site uses Cookies. View our privacy policy here.