DOI:
10.1039/D4QM00609G
(Research Article)
Mater. Chem. Front., 2025,
9, 109-121
MnOx embedded in 3D foam-like polymer composite for high-performance flexible supercapacitors†
Received
18th July 2024
, Accepted 31st October 2024
First published on 31st October 2024
Abstract
3D foam-like composites with a large specific surface area and a well-distributed interconnected pore structure have been recognized as promising materials for energy storage devices. In this study, a novel composite electrode (PEUS-Mn-PS) consisting of a 3D foam-like PEUS matrix embedded with manganese dioxide (MnOx) was prepared using a simple and facile method. The PEUS matrix was fabricated by incorporating poly(3,4-ethylenedioxythiophene):poly(styrenesulfonate) (PEDOT:PSS) and water polyurethane (PU), where a sacrificial template of poly(3,4-ethylenedioxythiophene) (PEDOT)-decorated Ni foam (NF) was utilized. Specifically, surface modification of NF with a thin layer of PEDOT resulted in the formation of a more regular 3D interconnected scaffold of PEU with more hydrophilic surface, facilitating homogeneous formation of the electrode materials and electrolyte infiltration. Benefiting from the high conductivity of PEDOT:PSS, large surface area provided by PEU, and high capacity offered by MnOx, the resulting flexible PEUS-Mn-PS electrode exhibited an exceptional areal specific capacitance of 681.7 mF cm−2 (∼486.9 F g−1) at 1 mF cm−2, much larger than 358.9 mF cm−2 of the PUS-Mn-PS electrode prepared without PEDOT modification and 318.7 mF cm−2 of the NF-Mn electrode synthesized through direct electrodeposition of MnOx on NF. The resulting PEUS-Mn-PS electrode allowed the assembled solid-state symmetric flexible SC to achieve an impressive energy density of 0.043 mW h cm−2 at a power density of 2.24 mW cm−2, while maintaining excellent electrochemical performance even under various bending angles. This work provides a new approach to designing high-performance flexible SC electrode materials using a simple, cost-effective, and environmentally friendly method.
Introduction
Over the past few decades, flexible supercapacitors (SCs) have garnered increasing attention due to the rapid development of flexible and wearable electronics, primarily attributed to their high power density, superior intrinsic safety and long cycling lives.1–3 Nevertheless, the weakness of low energy density still hinders their practical application. Generally, the energy storage capacity of SCs largely depends on the electrochemical performance of their electrodes. An ideal flexible SC electrode should possess the following characteristics: (i) providing a large surface area to accommodate high capacitive materials, (ii) affording abundant channels for fast ion transport, and (iii) having sufficiently high conductivity for rapid electron transfer and favorable mechanical strength to sustain various deforming conditions. For these reasons, to achieve high performance of flexible SCs, it is critical to choose/design the electrode materials with the appropriate structure.4,5
To date, a wide range of materials, including carbon-based materials, conductive polymers, and metal oxides, have been extensively investigated as active materials in SC electrodes.6–9 Among them, MnOx has garnered significant attention due to its high theoretical capacity, natural abundance, and environmental compatibility. However, the poor electronic and ionic conductivity of MnOx results in actual capacitance values that are much lower than their theoretical counterparts.10–12 Incorporating MnOx with highly conductive polymers and/or carbon-based materials can effectively address this issue.11 However, the majority of synthesized MnOx-based hybrid materials exist in powder form, necessitating the incorporation of binders to fix them onto current collectors.13,14 Unfortunately, due to the poor conductivity of binders, their utilization may impede electrode performance. Thus, considerable efforts have been devoted to developing binder-free electrode materials.15
Due to their large specific surface area and well-distributed interconnected pore structure, metallic foams, such as Ni foam (NF) and stainless-steel mesh (SSM), have been employed as electrode scaffolds. Compared with the solid film scaffold, these foams not only enhance the mass loading of electroactive materials but also facilitate efficient charge transport within the electrode.16–18 For example, Karaca et al. fabricated a flexible p(Py-co-Cz)/MnOx composite on a stainless steel mesh (SSM), where the synthesized MnOx nanoparticles were co-electrodeposited with pyrrole (Py) and carbazole (Cz) monomers. The electrode exhibited a high specific capacitance (Cs) of 352 F g−1 at 10 A g−1.19 Zhang et al. electrodeposited MnOx onto the NF decorated with alcohol clothing and reported a Cs of 469 F g−1 at 1 A g−1.20 However, the utilization of metal foams significantly increases the weight of the electrodes while making minimal contributions to their capacity. Recently, the utilization of carbon-based foams, such as graphene foam and CNF foam, has garnered significant attention owing to their lightweight nature, high conductivity, and good capacity.21–23 For instance, Li et al. successfully developed a novel multilevel porous graphite foam (MPG), which was subsequently functionalized with MnOx to enhance the capacity. Based on the entire electrode, the Cs of the fabricated MPGM can still reach 260 F g−1 (1 mV s−1).24 However, the 3D flexible carbon-based foams were prepared either through a hydrothermal self-assembly method followed by freeze drying/lyophilization to obtain aerogels or via direct growth on various pore-structure substrates using chemical vapor deposition, followed by removing substrates. Both methods are complicated and time-consuming. The associated drawbacks, including critical preparation conditions, environmental unfriendliness, and small-scale output, pose obstacles to their further development. Moreover, the limited deformability of metal foams and carbon-based foams severely restrict the flexibility of most reported flexible MnOx-based electrodes.19,25,26 Therefore, it is urgent and essential to develop a 3D flexible scaffold with inherently high-level deformation tolerance using facile, low-cost yet scalable methods.
As an alternative material, polymers can be utilized as the substrate and/or active components of flexible SCs due to their exceptional flexibility, cost-effectiveness, and lightweight nature.27,28 Moreover, the conductivity/capacity of polymers can be enhanced through doping or incorporating additives. Importantly, employing deposited polymers as the current collector not only simplifies the fabrication process but also reduces electrode costs. Consequently, polymers hold tremendous potential as electrode materials for energy storage and conversion devices. However, there is limited literature available on utilizing 3D foam-like polymer matrices, specifically for immobilizing MnOx, in SCs.
Based on the above discussion, we designed a novel flexible 3D porous interconnected sandwich-like electrode composed of MnOx and polymers using a facile solution-based method. The active material of MnOx was embedded in high conductive layers of PEDOT:PSS within the 3D polyurethane (PU) prepared using NF as the sacrificial template. After decorating NF with a thin layer of poly(3,4-ethylenedioxythiophene) (PEDOT), the synthesized porous PEU matrix exhibited a more regular 3D interconnected structure as well as a more hydrophilic surface, facilitating the infiltration of PEDOT:PSS and penetration of electrolyte. As a result, the resulting flexible PEUS-Mn-PS electrode demonstrated an outstanding areal specific capacitance (Ca) of 681.7 mF cm−2 (∼486.9 F g−1) at 1 mF cm−2, which is much larger than 358.9 mF cm−2 for PEU-Mn-PS without PEDOT decoration and 318.7 mF cm−2 for NF-Mn prepared by direct electrodeposition of MnOx on NF. In addition, the flexible solid PEUS-Mn-PS SC was fabricated, which exhibited an excellent areal capacitance of 282 mF cm−2, superior energy density of 0.043 mW h cm−2 at the power density 2.24 mW cm−2, and long cycling performance.
Experiments
Materials
The waterborne polyurethane with a solids content of 40% was purchased from Shanghai Perfemiker Chemical Technology Co. Carbon black (CB), dimethyl sulfoxide (DMSO), isopropyl alcohol (IPA), 3,4-ethylenedioxythiophene (EDOT), sodium dodecyl sulfate (SDS) and manganese sulfate (MnSO4) were purchased from Aladdin, PEDOT:PSS was purchased from Adamas, and ferric iron chloride (FeCl3·6H2O), sodium sulphate (Na2SO4) and sodium chloride (NaCl) were purchased from Sinopharm Chemical Reagent Corporation.
Preparation of 3D PU and PEU matrices
The PU mixed solution was prepared by vigorously mixing 5 g of PU in 2.5 mL of DMSO, along with the addition of 0.3 g of carbon black and 1 mL of PH1000, under constant stirring in an ice bath for 3 hours. Subsequently, the NF was immersed in the PU mixed solution for 5 min, followed by annealing at 95 °C for 20 min to synthesize the NF-PU matrix. After immersing the NF-PU matrix in a 1 M FeCl3 solution for 3 hours to etch away NF, a 3D porous PU foam was achieved. The PEU foam was produced using the same process, except for incorporating PEDOT onto NF via the electrodeposition method.
Fabrication of PUS-Mn-PS and PEUS-Mn-PS electrode
After the deposition of PEDOT:PSS on the PU matrix, the conductive PUS scaffold was achieved. To obtain the PUS-Mn composite, MnOx was electrodeposited into a two-electrode system at a constant current of 0.003 A, where the prepared scaffold served as the working electrode and a Pt sheet was used as the counter electrode. The electrodeposition area was 1 × 1 cm2. The PUS-Mn-PS electrode was prepared by coating another PEDOT:PSS on the PUS-Mn composite. After depositing MnOx for 10 minutes, the PUS-Mn-PS prepared with different thicknesses of NF were denoted as NF-X, where X represents the thickness of NF.
The PEUS-Mn-PS electrode was prepared using the same procedure as for the PUS-Mn-PS electrode. The PEUS-Mn-PS electrodes, prepared with different deposition time of PEDOT and a 10-minute deposition of MnOx, were named PX, where X denotes the PEDOT deposition time. Additionally, the PEUS-Mn-PS electrodes prepared with different deposition time of MnOx were named Mn-X, where X is the deposition time of MnOx.
For comparison, an NF-Mn electrode was fabricated by depositing MnOx directly onto the NF substrate with a deposition time of 15 minutes. With a deposition time of 15 minutes for MnOx, the mass loading of MnOx was approximately ∼1.4 mg for PEUS-Mn-PS and ∼1.2 mg for NF-Mn.
Material characterization and electrochemical measurement
The microscopic morphology of the composite was studied and analyzed using field emission scanning electron microscopy (FESEM, NovaNano450). The crystal structures of these composites were analyzed using an X-ray diffractometer (XRD, D8 ADVANCE). The intrinsic structural information of all samples was examined using laser Raman spectroscopy (Raman, DXR). X-ray photoelectron spectroscopy (XPS) was performed using a Thermo Scientific K-Alpha instrument.
Cyclic voltammetry (CV), galvanostatic charge–discharge (GCD), and electrochemical impedance spectroscopy (EIS) tests were performed in a three-electrode system using 1 M Na2SO4 solution as the electrolyte, where the as-prepared composites were the working electrode, and platinum foil (Pt) and silver chloride (Ag/AgCl) were used as the counter and reference electrodes.
In the three-electrode systems, the areal capacitance Ca (mF cm−2) of composite electrodes calculated from GCD was according to eqn (1):29
|  | (1) |
where,
A (cm
2) is the electrode area, Δ
V (V) is the working potential range,
I (A) is the discharge current and Δ
t (s) is the discharge time.
The energy density (mW h cm−2) and power density (mW cm−2) of the SCs can be calculated according to the following equations:30
|  | (2) |
|  | (3) |
Results and discussion
Structural and morphological characterizations
The fabrication process of the PEUS-Mn-PS and PUS-Mn-PS composites is illustrated in Scheme 1(a), where both materials are prepared using the same procedure except for the template employed. The template for PEUS-Mn-PS was PEDOT-NF which was synthesized through electrodeposition of PEDOT onto NF. Taking the preparation of PEUS-Mn-PS as an example, initially, the PEDOT-NF was immersed in a mixed PU solution and then annealed in air to obtain PU-decorated PEDOT-NF (PEU-NF). After removing NF, a 3D porous interconnected PEU matrix with a structure resembling that of NF was obtained. Subsequently, a highly conductive substrate was prepared by depositing PEDOT:PSS onto the PEU matrix (PEUS), which served as the scaffold for electrodeposition of high-capacitance MnOx. Finally, by incorporating an additional layer of PEDOT:PSS, a sandwich-structured electrode (PEUS-Mn-PS) consisting of current collector (PEDOT:PSS)-active material (MnOx)-current collector (PEDOT:PSS) was formed, as illustrated in Scheme 1(b). In previous studies, the prepared flexible polymer substrates predominantly exhibited a solid film-like structure, which inevitably created dense electrodes with fewer electroactive sites and impeded electrolyte ion permeation and diffusion due to their inherent small surface area and porosity.31,32 However, the PEU matrix in this study exhibits a 3D porous interconnected structure, which not only provides ample surface area for active materials/current collector deposition but also enhances the contact area between the active materials and electrolyte, thereby accelerating the redox reaction within the electrode.
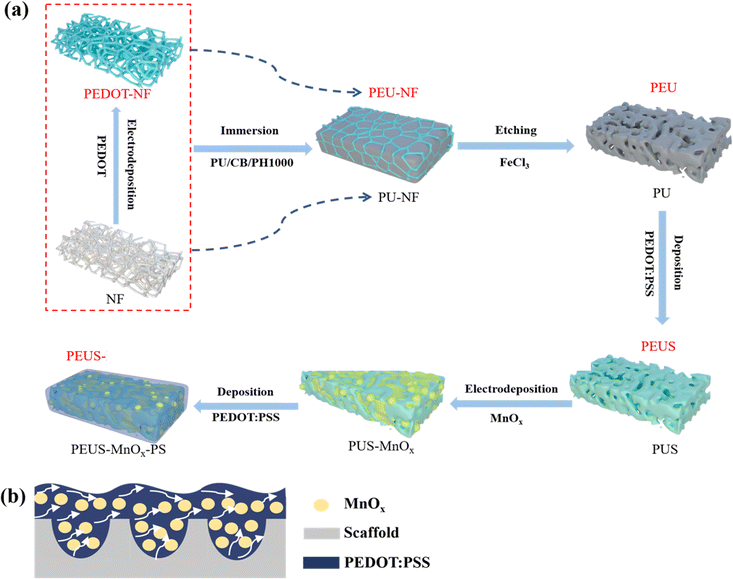 |
| Scheme 1 Schematic diagrams of (a) the fabrication processes for the PEUS-Mn-PS and PUS-Mn-PS composites and (b) the electron/ion transport within the electrodes. | |
The thickness of the NF template could influence the 3D porous structure of the synthesized matrix.26 Fig. S1 (ESI†) presents cross-section SEM images of PU matrices prepared using NF templates with varying thicknesses. When a thinner NF template (0.5 mm) was employed, the resulting PU matrix exhibited a denser film-like structure due to the substantial pore filling (Fig. S1a, ESI†). Fortunately, increasing the thickness of the NF template to 1 mm allowed for most pores within the prepared PU matrix to be preserved (Fig. S1b, ESI†). However, an excessive increase in NF thickness could impede solution penetration into its bottom part due to surface tension, hindering further growth of the PU matrix. For instance, when using an NF template with a thickness of 1.5 mm, pore blockage resultes in the thickness of the PU matrix being only ∼0.85 mm (Fig. S1c, ESI†). Considering that blocked pores adversely affect active material deposition and electrolyte permeation within the PU matrix, we selected an NF template with a thickness of 1 mm for our study.
The SEM image of the pristine NF is presented in Fig. 1(a). It can be observed as a 3D interconnected porous scaffold with smooth pores (∼200–500 μm) throughout the space. After being decorated with a thin layer of PEDOT, the pore surface becomes rougher, and numerous wrinkles can be observed (Fig. 1(b)). A closer view reveals that the wrinkles are composed of PEDOT nanowires (Fig. S2, ESI†). The 3D porous structure of the NF template can be maintained after removing NF. However, as illustrated in Fig. 1(c), damages can be observed within the PU matrix, and certain pores of PU matrix exhibit significantly larger dimensions compared to those of NF. In contrast, the PEU skeleton maintains a hollow internal structure similar to that of pristine NF, although its pore size is reduced to ∼100–200 μm (Fig. 1(d)). Compared with PU, the more regular structure of PEU could be ascribed to the rough surface of PEDOT-NF. As presented in Fig. 2(a), the water contact angle (WCA) for NF is 123.8°, which significantly decreases to 21.2° for PEDOT-NF (Fig. 2(b)), indicating that the foam surface transforms from hydrophobic to hydrophilic. The transformation benefits the thorough infiltration of the PU mixed solution into all pores within the foam. Moreover, due to its rougher surface, PEDOT-NF demonstrates enhanced solution retention after being removed from the PU solution, facilitating the formation of a compact and uninterrupted PU layer encompassing NF. Consequently, upon removal of the NF template, a resulting PEU layer can successfully replicate both the continuous and porous 3D structure provided by the NF template, although with reduced pore size.
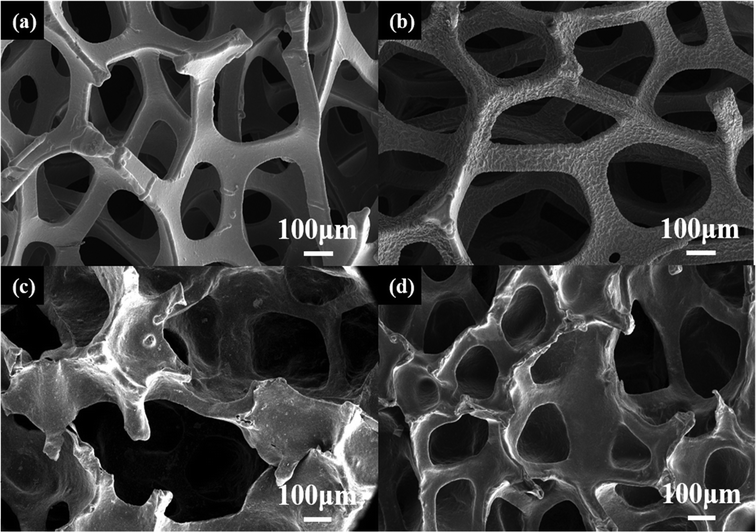 |
| Fig. 1 SEM images of (a) NF, (b) PEDOT-NF, (c) PU and (d) PEU. | |
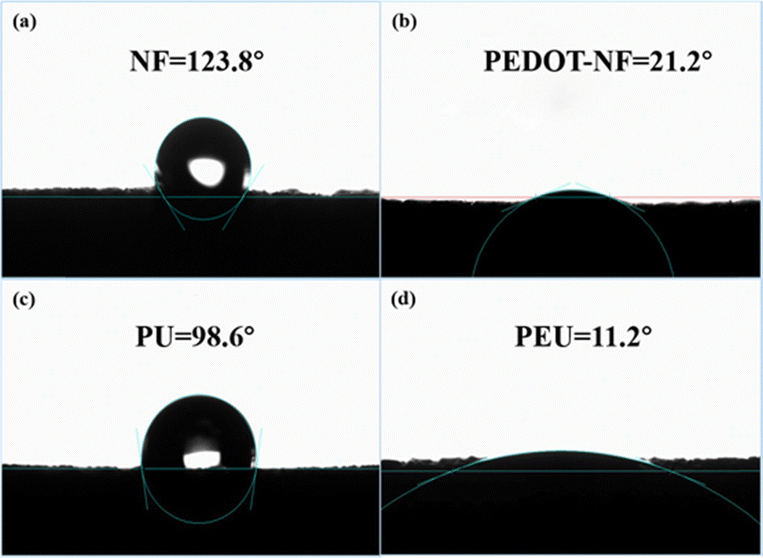 |
| Fig. 2 Water contact angles of (a) NF, (b) PEDOT-NF, (c) PU and (d) PEU. | |
The WCA for PU and PEU is 98.6° and 11.2°, respectively, as demonstrated in Fig. 2(c) and (d). The significantly reduced WCA for the PEU matrix promotes efficient infiltration of the PEDOT:PSS solution into all the pores within it. As illustrated in Fig. 3(a), the distribution of the PEDOT:PSS layer on the PEU matrix is homogeneous, effectively filling or coating the pores within the prepared PEUS matrix without any surface aggregation. A closer examination reveals that the PEDOT:PSS layer is tightly adhered to the PEU matrix (Fig. 3(b)).
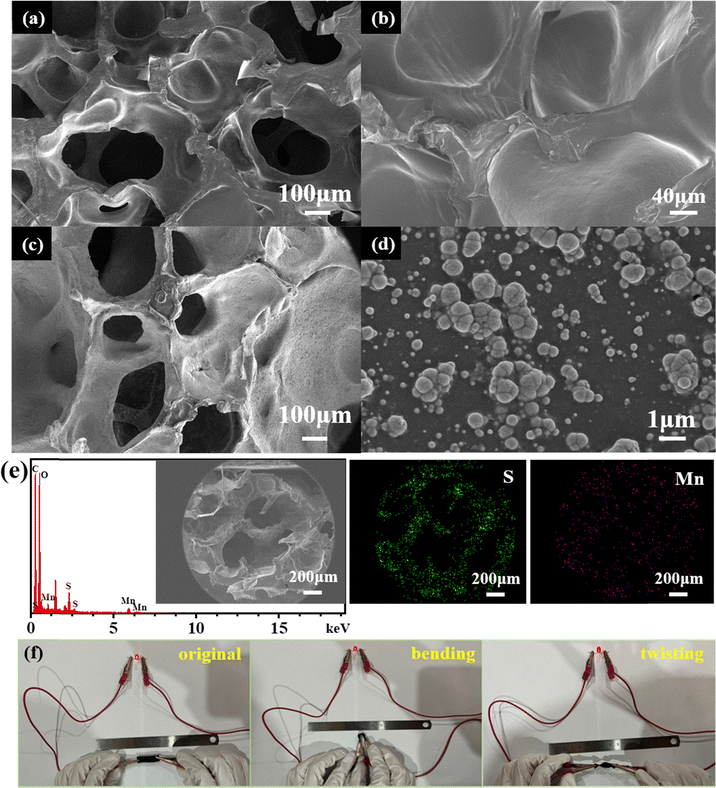 |
| Fig. 3 (a) and (b) SEM images of PEUS at different magnifications; (c) and (d) SEM images of PEUS-Mn at different magnification; (e) EDS spectrum of PEUS-Mn and the corresponding elemental mapping images; (f) optical images of PEUS-Mn-PS electrodes as a circuit conductor in their original, bent, and twisted form. | |
Notably, due to the high conductivity of the PEDOT:PSS layer, MnOx can be prepared on the PEUS scaffold via the electrodeposition method (PEUS-Mn). After being decorated with MnOx, as shown in Fig. 3(c), the macroporous structure within the PEUS-Mn composite is clearly observed, demonstrating that the 3D porous structure of the PEUS scaffold is well-preserved. At a relatively higher magnification, the flower-like MnOx particles are well-distributed on the surface of PEUS (Fig. 3(d)). Fig. 3(e) shows the EDX results of PEUS-Mn. A homogeneous distribution of the Mn element is observed throughout the entire cross-section of the PEUS-Mn composite, suggesting that MnOx is deposited not only on the outer surface but also on the whole surface within the PEUS scaffold. The successful deposition of MnOx throughout the PEUS-Mn composite further confirms that the highly conductive PEDOT:PSS layer can be uniformly coated with all the pores within the PEU matrix. To enhance the conductivity of the electrode, another PEDOT:PSS layer was deposited on the composite, resulting in a sandwich with the active material embedded between two current collectors for the electrode of PEUS-Mn-PS. Due to the high conductivity of the PEDOT:PSS layer as well as the excellent flexibility of PEU, as shown in Fig. 3(f), the resulting free-standing 3D PEUS-Mn-PS electrode obtained through the strategy exhibits excellent conductive and mechanical properties.
The XRD patterns of PEU, PEUS, and PEUS-Mn are depicted in Fig. 4(a). It is evident that all samples exhibit three diffraction peaks at 21.48°, 22.37°, and 23.90°, which can be attributed to the incorporation of CB into the PU matrix.33,34 Upon coating with the PEDOT:PSS layer, the intensity of these peaks decreases in PEUS and reaches its lowest value in PEUS-Mn due to an additional MnOx coating. Obviously, the peaks intensity for MnOx is relatively low, indicating that the synthesized MnOx has a low degree of crystallinity. The main diffraction peaks at 37° and 66° are attributed to the (006) and (119) of MnO2 (JCPDF 30-0820).35,36 As reported, the electrode material with low crystallinity exhibits enhanced ionic conductivity and reduced diffusion paths, improving the capacity of the electrode.37,38 The Raman spectra of the samples are shown in Fig. 4(b). The characteristic Raman bands at 1340 cm−1 and 1580 cm−1 in PEU are caused by vibration of the graphite lattice plane resulting from the addition of CB. Similarly, the density of the bonds is also reduced upon coating with the PEDOT:PSS layer. The presence of the bond at 1428 cm−1 in PEUS is caused by the stretching vibration of Cα
Cβ in PEDOT:PSS. For PEUS-Mn, the smaller supplemental peak observed at 648 cm−1 is associated with MnO2 crystals.39,40
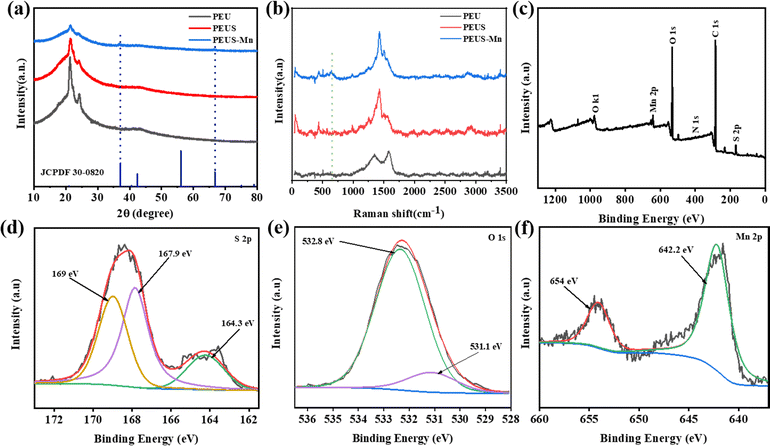 |
| Fig. 4 XRD patterns (a) and Raman spectra (b) for PEU, PEUS and PEUS-MnOx. X-ray photoelectron spectroscopy (XPS) of (c) survey; (d) S 2p; (e) O 1s; and (f) Mn 2p for PEUS-MnOx. | |
XPS analysis was used to provide details of the elemental composition and valence state of PEUS-Mn. As shown in Fig. 4(c), the presence of Mn 2p, S 2p, C 1s, N 1s, and O 1s in the full spectrum of PEUS-Mn reveals the existence of Mn, S, C, N, and O elements in the composite. In the high-resolution S 2p spectrum (Fig. 4(d)), three main peaks at 164, 167.9, and 169 eV can be ascribed to the presence of sulfur in the PEDOT/PEDOT:PSS structure.41 The O 1s peak can be deconvoluted into two peaks (Fig. 4(e)), where the peaks located at 531.1 and 532.8 eV are attributed to C
O/Mn–O–Mn bonds and C–OH/C–O–C bonds, respectively.42 In the Mn 2p spectrum (Fig. 4(f)), two peaks centered at 642.2 and 654 eV correspond to the spin-orbit doublets of Mn 2p3/2 and Mn 2p1/2, respectively. The observed binding energy separation of 11.8 eV agrees with the reported data for MnO2.43,44
Based on the discussion above, we have successfully fabricated a 3D porous interconnected PEU matrix with a large specific surface area, which is conducive to construct highly conductive and high-capacity flexible electrodes. Using the PEU as the substrate, MnOx was electrodeposited between the PEDOT:PSS layers. The resulting PEUS-Mn-PS integrates the functionalities of the current collector and active species, allowing it to be directly used as an electrode for flexible SCs. Furthermore, the facile and cost-effective solution method employed for synthesizing PEUS-Mn-PS facilitates easy reproducibility and scalability of the electrode.
Electrochemical properties of the electrodes
Electrochemical performances of the fabricated electrodes were evaluated in a three-electrode system using 1 M Na2SO4 as the electrolyte. Initially, we investigated the impact of NF thickness on the electrode performance. As depicted in Fig. S3 (ESI†), the NF-1.0 electrode, prepared using a 1 mm NF template and MnOx deposition time for 10 minutes, exhibits the highest Ca of 289.8 mF cm−2 at 1 mA cm−2, surpassing the Ca values of NF-0.5 (233.8 mF cm−2) and NF-1.5 (117.4 mF cm−2). The superior value of NF-1.0 can be attributed to its interconnected porous structure similar to that of the NF template. As shown in Fig. S1 (ESI†), the majority of pores within the PU matrix prepared with thinner or thicker NF exhibit blockage, which hampers electrolyte infiltration into the composite and consequently diminishes the electrochemical performance of the corresponding synthesized electrodes. Subsequently, we also examined the influence of PEDOT deposition time on the electrode performance. As shown in Fig. 2, decorating NF with PEDOT can reduce its hydrophobicity, thereby facilitating the infiltration of PU mixed solution into the foam. However, excessive PEDOT deposition may impede PU solution penetration, compromising the 3D interconnected structure (Fig. S4, ESI†). As shown in Fig. S5 (ESI†), with the same deposition time of MnOx (10 min), the P2 electrode prepared with a 2 min deposition time of PEDOT demonstrates the largest CV integral area, longest discharging time, and highest capacity retention. Finally, the optimized deposition time of MnOx was performed. The electrochemical performance of the electrode demonstrates enhancement with increasing deposition time of MnOx, as illustrated in Fig. S6 (ESI†). However, beyond a deposition time of 15 minutes, although the integral area, discharging time and Ca continue to increase, the capacity retention significantly decreases with an increased current density. This decrease in capacity retention may be attributed to the unique structure of PEUS-Mn. As depicted in Fig. 3(c), PEUS-Mn exhibited a large-scale macroporous structure while maintaining solid film properties in smaller regions. With an increasing deposition time, the thickness of the MnOx layer increased (Fig. S7, ESI†). However, due to the intrinsic low conductivity of MnOx and reduced contact area with the electrolyte, there was an associated rise in charge/ion transfer resistance of the electrode. Consequently, electrodes with longer deposition time of MnOx exhibit a more pronounced decline in capacity retention as the current densities increase. Therefore, the optimized deposition time for MnOx in the PEUS-Mn-PS electrode is 15 min.
The electrochemical performance of NF-Mn (prepared by directly depositing MnOx on NF), PUS-Mn-PS (prepared without PEDOT decoration), and PEUS-Mn-PS (prepared with PEDOT decoration) is compared in Fig. 5(a)–(c). As shown in Fig. 5(a) and (b), when MnOx is deposited for 15 minutes, NF-Mn exhibits the smallest enclosed CV area and shortest discharge time, suggesting that NF-Mn processes the lowest Ca. Utilizing a 3D polymer matrix as the scaffold can enhance the electrochemical performance of the electrode, which may be ascribed to the larger capacitive contribution from the polymer matrix. As shown in Fig. S8 (ESI†), the Ca for NF is only 3.75 mF cm−2, much smaller than those of PUS (62.3 mF cm−2) and PEUS (94.2 mF cm−2). As anticipated, PEUS-Mn-PS exhibits both the largest CV integral area and the longest discharge time. The Ca calculated from the discharge time at 1 mA cm−2 for all the samples is illustrated in Fig. 5(c), which is 681.7, 358.9, and 318.7 mF cm−2 for NF-Mn, PUS-Mn-PS, and PEUS-Mn-PS, respectively.
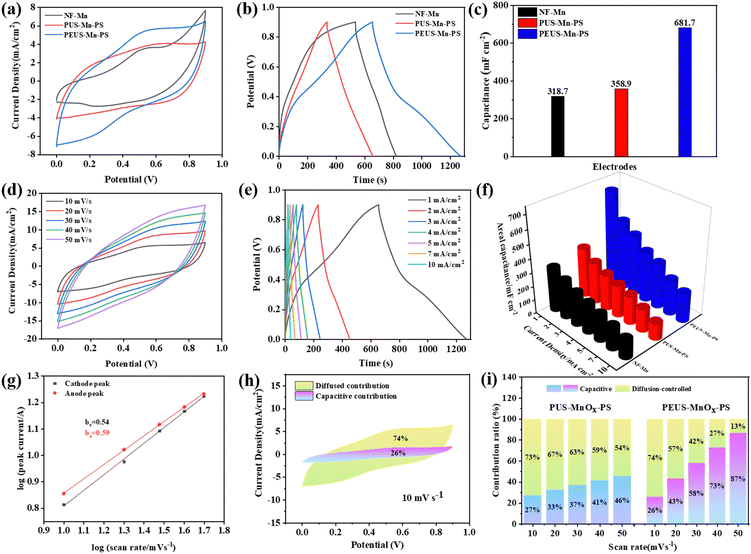 |
| Fig. 5 CV curves at 10 mV s−1 (a), GCD curves at 1 mA cm−2 (b) and Ca at 1 mA cm−2 (c) for NF-Mn, PUS-Mn-PS, and PEUS-Mn-PS; CV curves at 10–50 mV s−1 (d) and GCD curves at 1–10 mA cm−2 (e) for PEUS-Mn-PS; plots of the Ca at different current densities (f) for NF-Mn, PUS-Mn-PS, and PEUS-Mn-PS; linear relationship of the peak current as a function of the scan rate observed from the CV curves (g) and the capacitive contribution (h) of PEUS-Mn-PS at 10 mV s−1. Histograms of the capacitance contributions at different scan rates (i) for PUS-Mn-PS and PEUS-Mn-PS. | |
The CV curves of PEUS-Mn-PS at different scan rates within a potential window of 0–0.9 V are presented in Fig. 5(d). As the scan rate increases, the current response exhibits an upward trend, resulting in slight variations in the shape of CV curves at different scan rates, thereby indicating the excellent capacitive behavior of PEUS-Mn-PS. Notably, at lower scan rates (10–20 mV s−1), certain redox reaction peaks associated with Mn/PEDOT redox reactions can be observed. However, these peaks become less prominent as the scan rate rises due to restricted charge/ion transfer diffusion. Peak currents were consistently observed at both ends of all CV curves. The relationship between scan rate (v1/2) and peak current is illustrated in Fig. S9 (ESI†). The fitted results indicate that the peak currents exhibit a favorable linear relationship with v1/2, implying a diffusion-controlled process at the electrode/electrolyte interface. As shown in Fig. 5(e), PEUS-Mn-PS exhibits an extended discharge time of ∼614 s at 1 mA cm−2, which can maintain ∼190 s even with a nine-fold increase in current density, revealing its rapid charge–discharge response. Additionally, all GCD profiles exhibit nearly symmetrical characteristics, suggesting its fast charge diffusion within the electrode. The CV curves at the corresponding scan rates and GCD curves at specific current densities for NF-Mn and PUS-Mn-PS are also performed in Fig. S10 (ESI†). Fig. 5(f) compares the Ca values at each current density of the samples. It can be observed that due to the minimal capacitance contribution of NF, the Ca value at each current density for NF-Mn is smaller than those of PUS-Mn-PS and PEUS-Mn-PS, which is 318.7 mF cm−2 at 1 mA cm−2 and decreases to 133.3 at 10 mA cm−2. At 1 mA cm−2, the Ca value for PUS-Mn-PS prepared without PEDOT decoration is ∼358.9 mF cm−2, while it can be enhanced to 681.7 mF cm−2 for PEUS-Mn-PS, representing an improvement of about 90%. The corresponding Cs for PEUS-Mn-PS is ∼486.9 F g−1. The values of Ca decrease as the current density increases. When increasing the current density to 10 mA cm−2, the Ca values for PUS-Mn-PS decrease to 118 mF cm−2, whereas it still reaches 212 mF cm−2 for PEUS-Mn-PS. Compared to PEU-Mn-PS, the higher Ca value at each current density of PEUS-Mn-PS can be attributed to the reduced WCA (Fig. 2(d)) and more regular 3D interconnected structure (Fig. 1(d)) of the PEU matrix. Both of them facilitate the homogeneous deposition of the current collect layer and active material throughout the entire 3D matrix, thereby affording more electroactive sites and significantly enhancing electrode conductivity. As shown in Fig. S11 (ESI†), the internal resistance (Rs) and charge-transfer resistance (Rct) for PEUS-Mn-PS are 19.5 and 13.6 Ω, respectively. Both values are significantly smaller than those of PUS-Mn-PS. The substantial reduction in Rs and Rct further confirms that the 3D porous structure of PEU offers more electroactive sites as well as enhanced electrode conductivity, leading to a higher reaction rate and improved charge transfer ability of the electrode. As shown in Table 1, the capacitance of PEUS-Mn-PS is superior to other previously reported MnOx-based electrodes, suggesting its good performance.
Table 1 Capacitance comparison of PEUS-Mn-PS with other previously reported MnOx-based electrodes
Electrode |
C
s (F g−1) |
C
a (mF cm−2) |
Ref. |
SG: porous laser-scan graphene.
SSM: stainless-steel mesh.
3DIHBC: 3D interconnected mesoporous honeycomb-like carbon by pyrolysis of purified bacterial cellulose.
|
SiNWs/PEDOT@Pt/MnOx |
— |
352.98 (2 mA cm−2) |
45
|
SG/MnO nanocomposite on PETa |
— |
470 (1 mA cm−2) |
46
|
MnOx/carbamide carbon nanofiber |
271 (1 A g−1) |
— |
47
|
p(Py-co-Cz)/MnOx on SSMb |
352 (5 A g−1) |
— |
19
|
DIHBC/MnO2 on NFc |
170 (1 A g−1) |
— |
48
|
MnOx on NF with alcohol clothing |
469 (1 A g−1) |
— |
20
|
MnO2-PANI/titanium foam |
— |
97.38 (0.2 mA cm−2) |
21
|
PEUS-MnOx-PS |
486.9 (0.7 A g−1) |
681.7 (1 mA cm−2) |
This work |
The electrochemical kinetic properties of PEUS-Mn-PS were investigated using the equation:
where
i represents the peak current density (mA cm
−2),
v denotes the scan rate (mV s
−1), and
a and
b are constants. As depicted in
Fig. 5(g), the logarithmic cathodic and anodic peak current densities exhibit a linear correlation with the logarithmic scan rate. The computed b values were found to be 0.54 and 0.59, indicating that the diffusion-controlled process predominantly contributes to the charge storage mechanism of PEUS-Mn-PS. Moreover, the capacitive (
k1ν) and diffusion-controlled (
k2ν1/2) contribution to the total charge storage can be quantified by the equation
As present in Fig. 5(h), 26% of the total Ca for PEUS-Mn-PS is contributed by the surface capacitive process at 10 mV s−1, implying a predominant contribution from the diffusion-controlled process, which is in agreement with the results from the peak current and scan rate (Fig. S8, ESI† and Fig. 5(g)). The capacitive contribution generally increases with the scanning rate. Interestingly, at each scan rate, PEUS-Mn-PS exhibits a greater surface capacitive contribution compared to PUS-Mn-PS (Fig. 5(i)). This can be contributed to the decoration of PEDOT on the template of NF, which benefits for infiltration of PU mix solution and PEDOT:PSS, thereby forming a more regular 3D interconnected structure with larger surface area and enhanced conductivity. The results are consistent with the findings from the EIS analysis.
Electrochemical properties of the symmetric SC
The practical applicability of PEUS-Mn-PS was assessed by fabricating a flexible all-solid-state symmetrical SC (SSC) using Na2SO4/PVA as the gel electrolyte and separator. Fig. 6(a) illustrates the CV curves of the device obtained at scan rates ranging from 10 to 50 mV s−1, which maintain their shape consistently across different scan rates, suggesting the excellent electrochemical reversibility of the device. Furthermore, the GCD curves exhibit a nearly symmetric shape at current densities ranging from 1 to 10 mA cm−2 (Fig. 6(b)), confirming the device has outstanding charge/discharge delivery capability as supported by the CV results. Calculating from the discharge time, the areal capacitance of the device was 282 mF cm−2 at 1 mA cm−2, which still retained 39 mF cm−2 even at 10 mA cm−2, revealing it has good reversible capability. The CV and GCD curves of the device with bending angles of 0°, 45°, 90°, 135°, and 180° are shown in Fig. 6(c) and (d). Minimal changes can be observed in both shapes of the CV and GCD curves, indicating that the device exhibits excellent flexibility and reversibility. As shown in Fig. 6(e), the device could maintain 78% of its initial capacitance after 1500 charge/discharge cycles at 2.0 mA cm−2, suggesting its good stability. Furthermore, as plotted in Fig. 6(f), the energy density of the device achieves 0.0257 mW h cm−2 at a power density of 0.450 mW cm−2. It can maintain 0.043 mW h cm−2 at the power density of 2.24 mW cm−2, which is much better than those of reported MnOx-based SSCs, such as S-LrGO/S-MnO–Mn3O4 based SSC49 (0.0147 mW h cm−2 at 1.29 mW cm−2), SiNW/PEDOT@Pt/MnOx based SSC45 (0.0133 mW h cm−2 at 0.125 mW cm−2), MnHCF-MnOx/ErGO based SSC50 (0.0023 mW h cm−2 at 0.5 mW cm−2), PET/Pt/MnO2 based SSC51 (0.00036 mW h cm−2 at 0.09 mW cm−2), V2O5//MnO2@PEDOT based SSC52 (0.0151 mW h cm−2 at 1.8 mW cm−2), Mn3O4/CC based SSC53 (0.025 mW h cm−2 at 0.64 mW cm−2), and LSG/MnO based SSC46 (0.00489 mW h cm−2 at 0.72 mW cm−2).
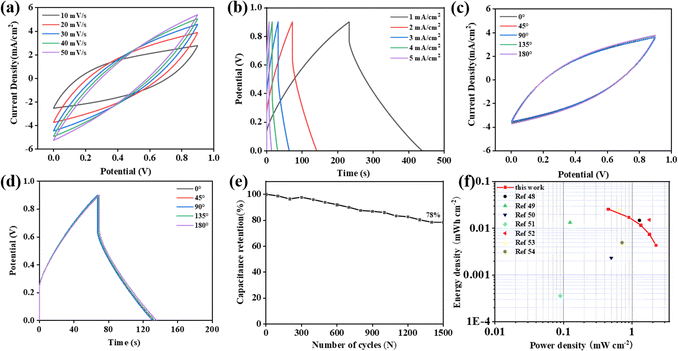 |
| Fig. 6 Electrochemical performance of the assembled flexible SCs devices. (a) CV curves at 10–50 mV s−1; (b) GCD curves at 1–10 mA cm−2; (c) CV curves at 20 mV s−1 and (d) GCD curves at 2 mA cm−2 for the device measured with different bending angles; (e) cycling stability at 2 mA cm−2 for 1500 cycles; (f) Ragone plots of the device and other reported MnOx-based SSCs for comparison. | |
Conclusions
In this work, we successfully developed a novel 3D porous interconnected sandwich-like electrode of PUS-Mn-PS with a facile solution-based method, where the active material of MnOx was electrodeposited between the two high conductive layers of PEDOT:PSS within the 3D PU matrix. The 3D PU matrix was prepared by employing NF as the sacrificial template. Interestingly, after decorating NF with a thin layer of PEDOT, the synthesized porous PEU matrix exhibited a more regular 3D interconnected structure as well as a more hydrophilic surface, facilitating the infiltration of PEDOT:PSS and penetration of electrolyte. The effect of the thickness of the NF template, the deposition time of the surface modification layer of PEDOT, and the deposition time of MnOx on the electrochemical performance of the electrodes was investigated. After optimization, the resulting flexible PEUS-Mn-PS electrode demonstrated an outstanding Ca of 681.7 mF cm−2 (∼486.9 F g−1) at 1 mA cm−2, which is much larger than 358.9 mF cm−2 for PUS-Mn-PS and 318.7 mF cm−2 for NF-Mn. In addition, the flexible solid PEUS-Mn-PS SC was fabricated, which exhibited an excellent areal capacitance of 282 mF cm−2, superior energy density of 0.043 mW h cm−2, and excellent electrochemical performance even while bending to various angles. This work demonstrates that high-performance flexible SC electrode materials can be prepared with facile, low-cost, and environmentally friendly methods and materials.
Author contributions
Xiaojuan Shen: supervision, conceptualization, funding acquisition, methodology, investigation, writing – original draft. Shouyan Sun: conceptualization, resources, formal analysis, visualization, writing – original draft preparation. Pengwei Liu: data curation, writing – review & editing, formal analysis. Manlin Wei: data curation, formal analysis.
Data availability
The data supporting this article have been included as part of the ESI.†
Conflicts of interest
There are no conflicts to declare.
Acknowledgements
We are grateful to the Youth Talent Cultivation Program of Jiangsu University.
References
- S. M. Desai, R. Y. Sonawane and A. P. More, Thermoplastic polyurethane for three-dimensional printing applications: A review, Polym. Adv. Technol., 2023, 34, 2061–2082 CrossRef CAS.
- Y. Zhao, C. Liu, Q. Lu, O. Ahmad, X. Pan and M. Daria, Recent progress on freestanding carbon electrodes for flexible supercapacitors, New Carbon Mater., 2022, 37, 875–897 CrossRef CAS.
- H. A. Khan, M. Tawalbeh, B. Aljawrneh, W. Abuwatfa, A. Al-Othman, H. Sadeghifar and A. G. Olabi, A comprehensive review on supercapacitors: Their promise to flexibility, high temperature, materials, design, and challenges, Energy, 2024, 295, 131043 CrossRef CAS.
- Y. Zhang, C. Zhou, X. Yan, Y. Cao, H. Gao, H. Luo, K. Gao, S. Xue and X. Jing, Recent advances and perspectives on graphene-based gels for superior flexible all-solid-state supercapacitors, J. Power Sources, 2023, 565, 232916 CrossRef CAS.
- R. I. Mohanty, A. Mukherjee, P. Bhanja and B. K. Jena, Organophosphorus ligand-assisted layered metal phosphonates as flexible electrode materials for high-performance interdigital micro-supercapacitors, J. Power Sources, 2024, 596, 234080 CrossRef CAS.
- S. Zhu, J. Ni and Y. Li, Carbon nanotube-based electrodes for flexible supercapacitors, Nano Res., 2020, 13, 1825–1841 CrossRef CAS.
- J. Liu, D. Tang, W. Hou, D. Ding, S. Yao, Y. Liu, Y. Chen, W. Chi, Z. Zhang, M. Ouyang and C. Zhang, Conductive polymer electrode materials with excellent mechanical and electrochemical properties for flexible supercapacitor, J. Energy Storage, 2023, 74, 109329 CrossRef.
- S. Jayakumar, P. C. Santhosh, M. M. Mohideen and A. V. Radhamani, A comprehensive review of metal oxides (RuO2, Co3O4, MnO2 and NiO) for supercapacitor applications and global market trends, J. Alloys Compd., 2024, 976, 173170 CrossRef CAS.
- D. Shao, X. Li, M. Yang, J. Li, R. Chen, X. Zheng, Y. Niu and Y. Qi, Synthesis of porous Mn2O3 architecture for supercapacitor electrode application, Colloids Surf., A, 2023, 658, 130532 CrossRef CAS.
- M. AlAnazi, T. Ghrib, F. Ercan, M. Alsubaie, T. Demirci, O. Kaygili, T. S. Kayed and I. Ercan, Structural, optical, and electrical investigation of multilayered MnO2(n)/NiO(p) heterojunctions for supercapacitors applications, Surf. Interfaces, 2023, 42, 103321 CrossRef CAS.
- Y. Zhao, H. Hao, T. Song, X. Wang, C. Li and W. Li, MnO2-graphene based composites for supercapacitors: Synthesis, performance and prospects, J. Alloys Compd., 2022, 914, 165343 CrossRef CAS.
- J. Yao, Y. Jia, Q. Han, D. Yang, Q. Pan, S. Yao, J. Li, L. Duan and J. Liu, Ternary flower-sphere-like MnO2-graphite/reduced graphene oxide nanocomposites for supercapacitor, Nanotechnology, 2021, 32, 185401 CrossRef CAS PubMed.
- R. Li, P. Chen, Y. He, Z. Wang, X. Xu, J. Shi, Y. Li, R. Jia and S. Han, Mn-doped V2O5@rGO towards high-performance electrode materials for aqueous zinc-ion supercapacitors, Ceram. Int., 2024, S0272884224016948 Search PubMed.
- F. Lu, Y. Ji, D. Shi, J. Yao and L. Pei, Electrochemically activated 3D Mn doped NiCo hydroxide electrode materials toward high-performance supercapacitors, J. Colloid Interface Sci., 2023, 641, 510–520 CrossRef CAS PubMed.
- O. Gerard, S. Ramesh, K. Ramesh, A. Numan, M. Norhaffis Mustafa, M. Khalid, S. Ramesh and S. K. Tiong, Evaluation of the effect of precursor ratios on the electrochemical performances of binder-free NiMn-phosphate electrodes for supercapattery, J. Colloid Interface Sci., 2024, 667, 585–596 CrossRef CAS PubMed.
- Y. Li, H. Jiang, X. Yan, W. Zhang, M. Zhang, W. Zhu, J. Pan, M. Sufyan Javed, W. Cheng and Y. Guan, Rationally designed Mn2O3/CuxO core–shell heterostructure generated on copper foam as binder-free electrode for flexible asymmetric supercapacitor, Appl. Surf. Sci., 2021, 566, 150715 CrossRef CAS.
- L. Zhang, X. Peng, J. Wan, Q. Cui, X. Chu, H. Suo, C. Zhao and D. He, Preparation of Stainless Steel Mesh-Supported MnO2 /Polypyrrole Nanocomposites as Binder-Free Electrode for Supercapacitor, Nano, 2020, 15, 2050031 CrossRef CAS.
- S. M. Qashqay, J. Rahimi, M. R. Zamani-Meymian and A. Maleki, Porous Co3O4/VS4/rGO-SDBS@NF nanoflower as a high performance supercapacitor electrode, J. Energy Storage, 2023, 72, 108548 CrossRef.
- E. Karaca, G. Özgenç, N. Ö. Pekmez and K. Pekmez, Nano MnOx encapsulated pyrrole-carbazole copolymer and polyvinyl chloride/carbon-coated flexible electrodes for solid-state supercapacitor cell, J. Energy Storage, 2022, 55, 105794 CrossRef.
- M. Zhang, Y. Chen, D. Yang and J. Li, High performance MnO2 supercapacitor material prepared by modified electrodeposition method with different electrodeposition voltages, J. Energy Storage, 2020, 29, 101363 CrossRef.
- J. Yan, Y. Huang, C. Chen, X. Liu and H. Liu, The 3D CoNi alloy particles embedded in N-doped porous carbon foams for high-performance microwave absorbers, Carbon, 2019, 152, 545–555 CrossRef CAS.
- P. F. Wu, H. Y. Yue, S. Huang, X. Gao, X. R. Guo, T. Zhang, Z. Z. Wang and H. T. Chen, ZnS Nanosphere Arrays/Graphene Foam for Voltammetric Sensing of Dopamine in the Presence of Uric Acid, IEEE Sens. J., 2020, 20, 2839–2843 CAS.
- C. Bacon, P. Simon, M. Salanne and A. Serva, Simulating the charging mechanism of a realistic nanoporous carbon-based supercapacitor using a fully polarizable model, Energy Storage Mater., 2024, 69, 103415 CrossRef.
- W. Li, X. Xu, C. Liu, M. C. Tekell, J. Ning, J. Guo, J. Zhang and D. Fan, Ultralight and Binder-Free All-Solid-State Flexible Supercapacitors for Powering Wearable Strain Sensors, Adv. Funct. Mater., 2017, 27, 1702738 CrossRef.
- J. S. Ko, M. B. Sassin, J. F. Parker, D. R. Rolison and J. W. Long, Combining battery-like and pseudocapacitive charge storage in 3D MnOx @carbon electrode architectures for zinc-ion cells, Sustainable Energy Fuels, 2018, 2, 626–636 RSC.
- Y. J. Yang, C. Jiang, N. Wang, S. Chen, Y. Cheng, P. Yang and M. Liu, In situ hydrothermal growth of manganese hexacyanoferrate with Ni foam as the sacrificing template for high-performance asymmetrical supercapacitor, Ionics, 2022, 28, 2957–2966 CrossRef CAS.
- S. Ghosh, J. Majhi, S. Sharma, K. Priya and A. Bandyopadhyay, A review on the development of electron and ion conductive polymer hydrogels and their composites for flexible and smart supercapacitors, J. Energy Storage, 2023, 74, 109423 CrossRef.
- D. Wang, F. Yang, L. Cong, W. Feng, C. Wang, F. Chu, J. Nan and R. Chen, Lignin-containing hydrogel matrices with enhanced adhesion and toughness for all-hydrogel supercapacitors, Chem. Eng. J., 2022, 450, 138025 CrossRef CAS.
- W. Liu, Y. Feng, X. Yan, J. Chen and Q. Xue, Superior Micro-Supercapacitors Based on Graphene Quantum Dots, Adv. Funct. Mater., 2013, 23, 4111–4122 CrossRef CAS.
- S. Zhou, C. Chiang, J. Zhao, G. Cheng, T. Bashir, W. Yin, J. Yao, S. Yang, W. Li, J. Wang, X. Wang, Y. Lin and L. Gao, Extra Storage Capacity Enabled by Structural Defects in Pseudocapacitive NbN Monocrystals for High-Energy Hybrid Supercapacitors, Adv. Funct. Mater., 2022, 32, 2112592 CrossRef CAS.
- V.-P. Vu, V.-D. Mai, D. C. T. Nguyen and S.-H. Lee, Flexible and Self-Healable Supercapacitor with High Capacitance Restoration, ACS Appl. Energy Mater., 2022, 5, 2211–2220 CrossRef CAS.
- F. Chen, X. Li, G. Liu, J. Zhao, L. Zhao, Y. Shi, Y. Shi, Z. Xu, W. Guo and Y. Liu, Flexible Electronic Skin Based on Silk/Polyurethane Composite Film Fabricated by Ink-Jet Printing and Electrodeposition, Adv. Mater. Technol., 2023, 8, 2201980 CrossRef CAS.
- X. Wang, D. Ouyang, Q. Wang, X. Zhang, Q. Liu, J. Chen, H. Zhou, Y. Zhang, X. Zhu and K. Ye, The preparation and properties performance of lithium carbonate@manganese dioxide@white carbon black electrode materials, Ionics, 2022, 28, 975–979 CrossRef CAS.
- N. L. Wu and S. Y. Wang, Conductivity percolation in carbon–carbon supercapacitor electrodes, J. Power Sources, 2002, 110, 233–236 CrossRef CAS.
- Z. Y. Sui, C. Wang, K. Shu, Q. S. Yang, Y. Ge, G. G. Wallace and B.-H. Han, Manganese dioxide-anchored three-dimensional nitrogen-doped graphene hybrid aerogels as excellent anode materials for lithium ion batteries, J. Mater. Chem. A, 2015, 3, 10403–10412 RSC.
- X. Zhang, S. Wu, S. Deng, W. Wu, Y. Zeng, X. Xia, G. Pan, Y. Tong and X. Lu, 3D CNTs Networks Enable MnO2 Cathodes with High Capacity and Superior Rate Capability for Flexible Rechargeable Zn–MnO2 Batteries, Small Methods, 2019, 3, 1900525 CrossRef CAS.
- X. Shen, T. Wang, X. Wei and S. Li, Facile Synthesis of Metal Oxide and Conductive Polymers around Silicon Nanowire Arrays for a High-Performance Aqueous Supercapacitor, ACS Appl. Energy Mater., 2022, 5, 2596–2605 CrossRef CAS.
- X. Shen, X. Wei, T. Wang, S. Li and H. Li, Polypyrrole embedded in nickel-cobalt sulfide nanosheets grown on nickel particles passivated silicon nanowire arrays for high-performance supercapacitors, Chem. Eng. J., 2023, 461, 141745 CrossRef CAS.
- F. Jing, Z. Ma, J. Wang, Y. Fan, X. Qin and G. Shao, Oxygen vacancy inducing phase transition during charge storage in MnOx@rGO supercapacitor electrode, Chem. Eng. J., 2022, 435, 135103 CrossRef CAS.
- W. Li, A. Xu, Y. Zhang, Y. Yu, Z. Liu and Y. Qin, Metal-organic framework-derived Mn3O4 nanostructure on reduced graphene oxide as high-performance supercapacitor electrodes, J. Alloys Compd., 2022, 897, 162640 CrossRef CAS.
- X. X. Li, X. H. Deng, Q. J. Li, S. Huang, K. Xiao, Z. Q. Liu and Y. Tong, Hierarchical double-shelled poly(3,4-ethylenedioxythiophene) and MnO2 decorated Ni nanotube arrays for durable and enhanced energy storage in supercapacitors, Electrochim. Acta, 2018, 264, 46–52 CrossRef CAS.
- X. Guan, L. Zhao, P. Zhang, X. Song, J. Liu and L. Gao, Self-Supporting Electrode of High Conductive PEDOT:PSS/CNTs Coaxial Nanocables Wrapped by MnO2 Nanosheets, ChemistrySelect, 2019, 4, 2009–2017 CrossRef CAS.
- M. A. A. Mohd Abdah, N. H. N. Azman, S. Kulandaivalu, N. Abdul Rahman, A. H. Abdullah and Y. Sulaiman, Potentiostatic deposition of poly(3, 4-ethylenedioxythiophene)
and manganese oxide on porous functionalised carbon fibers as an advanced electrode for asymmetric supercapacitor, J. Power Sources, 2019, 444, 227324 CrossRef CAS.
- D. S. Patil, S. A. Pawar and J. C. Shin, Silver decorated PEDOT:PSS wrapped MnO2 nanowires for electrochemical supercapacitor applications, J. Ind. Eng. Chem., 2018, 62, 166–175 CrossRef CAS.
- X. Shen, X. Wei, T. Wang, S. Li and H. Li, Solution-processable hierarchical SiNW/PEDOT/MnOx electrodes for high-performance supercapacitors, Mater. Chem. Front., 2022, 6, 2894–2904 RSC.
- M. Gao, X. Dong, K. Wang, W. Duan, X. Sun, C. Zhu and W. Wang, Laser direct preparation and processing of graphene/MnO nanocomposite electrodes for microsupercapacitors, J. Energy Storage, 2021, 33, 102162 CrossRef.
- E. Samuel, H. S. Jo, B. Joshi, H. G. Park, Y. I. Kim, S. An, M. T. Swihart, J. M. Yun, K. H. Kim and S. S. Yoon, High-performance supercapacitors using flexible and freestanding MnOx/carbamide carbon nanofibers, Appl. Surf. Sci., 2017, 423, 210–218 CrossRef CAS.
- J. Zhang, X. Du, H. Kimura, C. Hou, X. Sun, X. Yang, Y. Zhang, X. Xie and W. Du, MnO2 nanoflowers loaded on three-dimensional interconnected bacterial cellulose-derived honeycomb-like carbon for high-performance supercapacitors, Appl. Surf. Sci., 2023, 623, 157095 CrossRef CAS.
- M. Gao, X. Dong, X. Mei, K. Wang, W. Wang, C. Zhu, W. Duan and X. Sun, Laser direct writing of graphene/MnO–Mn3O4 doped with sulfur for high-performance microsupercapacitors, J. Energy Storage, 2022, 49, 104118 CrossRef.
- J. Liang, B. Tian, S. Li, C. Jiang and W. Wu, All-Printed MnHCF-MnOx-Based High-Performance Flexible Supercapacitors, Adv. Energy Mater., 2020, 10, 2000022 CrossRef CAS.
- X. Long, Z. Zeng, E. Guo, X. Shi, H. Zhou and X. Wang, Facile fabrication of all-solid-state flexible interdigitated MnO2 supercapacitor via in situ catalytic solution route, J. Power Sources, 2016, 325, 264–272 CrossRef CAS.
- Q. He, J. Ye, Z. Peng, Y. Guo, L. Tan and Y. Chen, Electrodeposition of poly(3,4-ethylenedioxythiophene) coated manganese dioxide nanospheres for flexible asymmetric planar supercapacitor with superior energy density, J. Power Sources, 2021, 506, 230176 CrossRef CAS.
- H. Jiang, C. Zhou, X. Yan, J. Miao, M. You, Y. Zhu, Y. Li, W. Zhou and X. Cheng, Effects of various electrolytes on the electrochemistry performance of Mn3O4/carbon cloth to ultra-flexible all-solid-state asymmetric supercapacitor, J. Energy Storage, 2020, 32, 101898 CrossRef.
|
This journal is © the Partner Organisations 2025 |
Click here to see how this site uses Cookies. View our privacy policy here.