DOI:
10.1039/D4QO01388C
(Research Article)
Org. Chem. Front., 2025,
12, 167-172
A two-step Kinugasa/Conia-ene-type sequential reaction for the asymmetric synthesis of 8-methylene-2,6-diazaspiro[3.4]octane-1,5-diones†
Received
29th July 2024
, Accepted 26th October 2024
First published on 28th October 2024
Abstract
2,6-Diazaspiro[3.4]octane-1,3-dione, integrating β-lactam and γ-lactam into one structure, is an important skeleton found in many bioactive molecules. This article describes a simple and practical synthesis of 8-methylene-2,6-diazaspiro[3.4]octane-1,5-diones from N-(prop-2-yn-1-yl)propiolamides and nitrones. The method proceeds via a two-step sequential process: a Kinugasa reaction, followed by a Conia-ene-type cyclization. The transformation afforded 8-methylene-2,6-diazaspiro[3.4]octane-1,5-diones in satisfactory yields and high diastereo- and enantioselectivity.
Introduction
β-Lactam and γ-lactam are privileged heterocyclic skeletons that widely exist in many bioactive molecules.1,2 2,6-Diaza-spiro[3.4]octane 1,5-diones and analogues, which integrate the two distinctive units of β-lactam and γ-lactam, have drawn tremendous attention in the field of synthetic chemistry and medicinal chemistry (Fig. 1).3 However, the methods for constructing such rigid structures have rarely been reported and multiple-step transformations are generally required.4 Simple and practical protocols toward 2,6-diazaspiro[3.4]octane 1,5-diones are highly desirable.
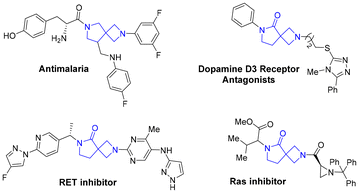 |
| Fig. 1 Selected examples of 2,6-diazaspiro[3.4]octane-containing bioactive molecules. | |
Among existing protocols,5 copper catalyzed 1,3-dipolar cycloaddition of terminal alkynes and nitrones is one of the most straightforward and practical methods for the formation of functionalized β-lactams. This type of cycloaddition, known as the Kinugasa reaction, was initially developed by Kinugasa and Hashimoto in 1972,6 and in 1995 Miura et al. reported the first example of asymmetric transformation for the construction of chiral β-lactams.7 Since then, significant progress has been witnessed with remarkable efforts from Fu, Tang, Feng, and Sawamura et al.8–10
According to the proposed mechanism,11 an enolate copper(I) intermediate was in situ generated in the Kinugasa reaction. Considerable efforts have been devoted to the development of cascade or one-pot transformations by trapping this Kinugasa enolate copper(I) with additional electrophiles (Scheme 1a).12 Elegant asymmetric cascade reactions have been realized by Fu,13 Enders,14 Xu15 and Lautens.16 However, the Kinugasa enolate copper(I) intermediate is extremely reactive with a strong tendency for protonation rather than competitive trapping by electrophiles. Thus, only highly electrophilic reagents or functional groups may be suitable for the trapping, greatly limiting the development of interrupted Kinugasa reactions.
 |
| Scheme 1 Copper(I)-catalyzed asymmetric Kinugasa and related reactions. | |
Recently, by taking propiolamides as the terminal alkyne substrates, we have developed a series of novel cascade reactions by interrupting the Kinugasa reaction with additional electrophiles. In these cascade reactions, the α-amide enolate copper(I) intermediates were trapped by intramolecular electrophilic functional groups such as aryl iodide,17a ketone17b or α,β-unsaturated ester groups17c to afford different spiro β-lactam products (Scheme 1b). The electron-withdrawing effect of the α-amide group made the intermediates more stable and a protonation–deprotonation equilibrium process under basic conditions is conducive to the following transformations. The use of propiolamides as the terminal alkyne substrates made the Kinugasa reaction and following transformations more controllable, and thus further expanded the reaction scope. In continuation of our interest in the Kinugasa reaction, an asymmetric two-step sequential reaction of N-(prop-2-yn-1-yl)propiolamides with nitrones was studied. The process is through a copper-catalyzed Kinugasa reaction, followed by a Conia-ene-type cyclization reaction,18 which provided a simple and practical access to chiral 8-methylene-2,6-diazaspiro[3.4]-octane-1,5-diones (Scheme 1c). Herein we'd like to report the details.
Results and discussion
Our exploration was initiated with a two-step reaction using N-benzyl-N-(prop-2-yn-1-yl)propiolamide 1a and nitrone 2a as the model substrates. As shown in Table 1, although a single solvent like MeCN can be used for both the steps,19 the desired product 3aa was afforded in only 31% yield and with a moderate enantiomeric ratio (78
:
22, Table 1, entry 1). Thus, the two step reactions were optimized in two different solvents. Generally, low temperature is good for the Kinugasa reaction since nitrones are not stable at high temperatures, whereas high temperature is beneficial for the second spirocyclization step. Thus, the first Kinugasa reaction was performed at −10 °C in one solvent for 6 hours, then another solvent was added to the mixture and the reaction temperature was elevated to 60 °C for the second spirocyclization step. Screening of solvents (Table 1, entries 1–8) revealed that PhCF3 is the optimal solvent for the Kinugasa reaction step and the polar solvent DMSO will facilitate the challenging spirocyclization process to afford the desired product 3aa in 49% yield (Table 1, entry 8) with good enantioselectivity (90.5
:
9.5 er) and diastereomeric ratio (3aa/3aa′ = 7
:
1). Different chiral bisoxazoline ligands L2–L8 were further screened (Table 1, entries 9–15), and it was found that L5 is the optimal ligand for this cascade reaction and the enantioselectivity of 3aa was increased to 94
:
6 (dr = 7
:
1, Table 1, entry 12). Next, different bases, such as tBuOLi, K2CO3 and Et3N, were examined and all performed poorly (Table 1, entries 16–18).20
Table 1 Optimization of the reaction conditionsa
Under the optimized conditions (Table 1, entry 12), we then explored the reaction scope with a variety of N-(prop-2-yn-1-yl)propiolamides and nitrones. As shown in Scheme 2, the N-(prop-2-yn-1-yl)propiolamide substrates 1b and 1c with different protecting groups on the amide nitrogen were first examined.
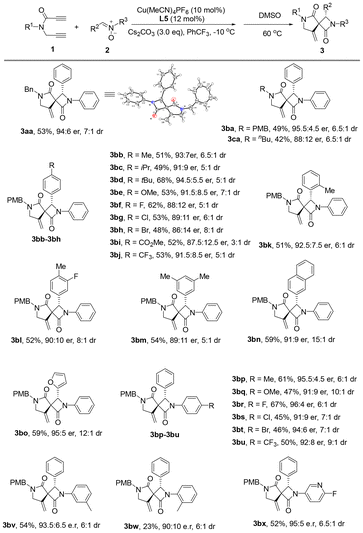 |
| Scheme 2 Substrate scope. | |
The PMB group displayed good performance and the expected spiro product 3ba was achieved in 49% yield with 95.5
:
4.5 er and 6.5
:
1 dr whereas the enantioselectivity for the N-nbutyl substrate 1c is inferior and the corresponding product 3ca was obtained in 42% yield, with 88
:
12 er and 6.5
:
1 dr. We next examined the scope of the nitrones by reacting with 1b. Different substituents on the C-aryl ring of nitrones, including alkyl, methoxy, halogen, ester and trifluoromethyl, showed good tolerance to the reaction system and the corresponding 3bb–3bj were delivered in moderate yields with high enantio- and diastereoselectivities. The steric factor did not affect the reaction efficiency, as shown by the formation of product 3bk with good stereoselectivity. Disubstituted phenyl, naphthyl and furyl-containing substrates were also applicable to the reaction system and the corresponding spiro products 3bl–3bo were furnished in 52–59% yields with good er and dr. Further substrate scope exploration suggested that both electron-donating groups and electron-withdrawing groups on the N-aryl ring of nitrones were also compatible with the reaction, and the corresponding products 3bp–3bv were furnished in acceptable yields and high enantio- and diastereoselectivities. When N-o-tolyl nitrone was exploited, an inferior yield was obtained, as observed in the case of 3bw. An N-pyridinyl nitrone was also explored and the desired product 3bx was afforded in satisfactory yield and high er and dr. The absolute configurations of 3aa and 3aa′ were unambiguously determined through X-ray single-crystal diffraction experiments.21
As shown in Scheme 3, a gram-scale synthesis of 3ba was performed to demonstrate the potential synthetic application of the method. The desired product 3ba was obtained in 48% yield and with 95.5
:
4.5 enantiomeric ratio under the standard conditions. Reduction of 3ba with LiAlH4/AlCl3 led to the formation of 2,6-diazaspiro[3.4]octane 4 in 50% yield without any loss of enantiomeric purity. Removal of the PMB group with CAN, followed by hydrogenation afforded product 6 with three contiguous stereogenic centres.
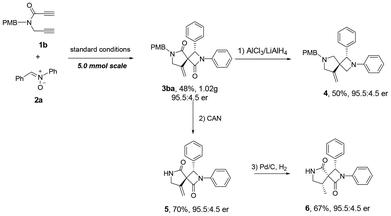 |
| Scheme 3 Gram-scale reaction and synthetic transformations of 3ba. | |
For a better understanding of the reaction process, the control experiments were performed as shown in Scheme 4. The reaction of 1a and 2a was carried out in PhCF3 at −10 °C for 6 hours, and the Kinugasa reaction product 7 was isolated in 55% yield with 94
:
6 er. Complete conversion of compound 7 was observed with 1.0 equivalent of Cs2CO3 in DMSO at 60 °C under copper- and ligand-free conditions. Similar base-promoted cyclization has been recognized as the variant of classic thermal Conia-ene reaction by Trauner and co-workers.18b In our case, the Cs2CO3-promoted transformation delivered the desired spirobilactam 3aa in 99% yield, with 94
:
6 er and 7
:
1 dr. The production of the minor diastereomer may be due to the high temperature in the second step. These results indicated that the Cu(MeCN)4PF6/L5 catalyst was required only for the first Kinugasa step and was not necessary for the second spirocyclization process. Based on the experimental observations and literature reports,11,18 our protocol proposed that the reaction is a two-step process as shown in Fig. 2. The first step is a copper-catalyzed asymmetric Kinugasa reaction and the enantioselectivity was controlled by the chiral ligand. The second step is possibly through a copper-promoted Conia-ene-type transformation of intermediate III or a base-promoted Conia-ene-type reaction of Kinugasa product 7,18 both leading to the formation of the spiro product.
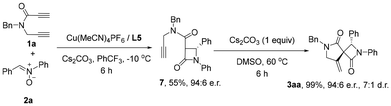 |
| Scheme 4 Control experiments. | |
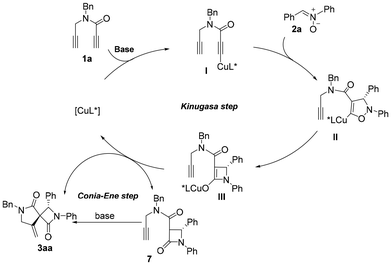 |
| Fig. 2 Proposed reaction process. | |
In summary, a mild copper-catalyzed asymmetric Kinugasa/Conia-ene sequential process of propargyl-tethered propiolamides with nitrones was developed. The reaction provided an efficient way for the assembly of a set of structurally novel chiral 8-methylene-2,6-diazaspiro[3.4]octane-1,5-dione in satisfactory yields and with good enantio- and diastereoselectivities. Further research on the applications of the method is ongoing in our laboratory.
Data availability
Experimental procedures, characterisation data, crystallographic data, NMR spectra and HPLC spectra for this article can be found in the ESI.†
Conflicts of interest
There are no conflicts to declare.
Acknowledgements
The authors are grateful to the Zhejiang Normal University and the National Natural Science Foundation of China (22001093 and 21971087) for financial support.
Notes and references
- For selected reviews on γ-lactam, see:
(a) L.-W. Ye, C. Shu and F. Gagosz, Recent Progress Towards Transition Metal-Catalyzed Synthesis of γ-Lactams, Org. Biomol. Chem., 2014, 12, 1833–1845 RSC;
(b) J. Caruano, G. G. Muccioli and R. Robiette, Biologically Active γ-Lactams: Synthesis and Natural Sources, Org. Biomol. Chem., 2016, 14, 10134–10156 RSC;
(c) G. Pandey, A. Mishra and J. Khamrai, Generation of All Carbon Quaternary Stereocenters at the C-3 Carbon of Piperidinonesand Pyrrolidinones and Its Application in Natural Product Total Synthesis, Tetrahedron, 2018, 74, 4903–4915 CrossRef CAS.
- For selected reviews on β-lactam, see:
(a) G. S. Singh, β-Lactams in the New Millennium. Part-I: Monobactams and Carbapenems, Mini-Rev. Med. Chem., 2004, 4, 69–92 CrossRef CAS;
(b) A. Brandi, S. Cicchi and F. M. Cordero, Chem. Rev., 2008, 108, 3988–4035 CrossRef CAS;
(c) P. Galletti and D. Giacomini, Monocyclic β-lactams: new structures for new biological activities, Curr. Med. Chem., 2011, 18, 4265–4283 CrossRef CAS PubMed;
(d) C. R. Pitts and T. Lectka, Chemical Synthesis of β-Lactams: Asymmetric Catalysis and Other Recent Advances, Chem. Rev., 2014, 114(16), 7930–7953 CrossRef CAS PubMed;
(e) L. M. Lima, B. N. M. da Silva, G. Barbosa and E. J. Barreiro, β-Lactam antibiotics: An overview from a medicinal chemistry perspective, Eur. J. Med. Chem., 2020, 208, 112829–112854 CrossRef CAS.
-
(a) C. L. Manach, J. Dam, J. G. Woodland, G. Kaur, L. P. Khonde, C. Brunschwig, C. L. Manach, M. Njoroge, K. J. Wicht, A. Horatscheck, T. Paquet, G. A. Boyle, L. Gibhard, D. Taylor, N. Lawrence, T. Yeo, S. Mok, R. T. Eastman, D. Dorjsuren, D. C. Talley, H. Guo, A. Simeonov, J. Reader, M. van der Watt, E. Erlank, N. Venter, J. W. Zawada, A. Aswat, L. Nardini, T. L. Coetzer, S. B. Lauterbach, B. C. Bezuidenhout, A. Theron, D. Mancama, L. L. Koekemoer, L.-M. Birkholtz, S. Wittlin, M. Delves, S. Ottilie, E. A. Winzeler, T. W. von Geldern, D. Smith, D. A. Fidock, L. J. Street, G. S. Basarab, J. Duffy and K. Chibale, Identification and Profiling of a Novel Diazaspiro[3.4]octane Chemical Series Active against Multiple Stages of the Human Malaria Parasite Plasmodium falciparum and Optimization Efforts, J. Med. Chem., 2021, 64, 2291–2309 CrossRef;
(b) S. W. Reilly, S. Griffin, M. Taylor, K. Sahlholm, C.-C. Weng, K. Xu, D. A. Jacome, R. R. Luedtke and R. H. Mach, Highly Selective Dopamine D3 Receptor Antagonists with Arylated Diazaspiro Alkane Cores, J. Med. Chem., 2017, 60, 9905–9910 CrossRef CAS PubMed;
(c) K. Fu, W. Xu, R. Yang, H. Zhao, H. Xu, Y. Wei, H. Liu, Y. Qiu, D. Chen, D. Guo and B. Xiong, 2,6-Diazaspiro[3.4]octan-7-one derivatives as potent sigma-1 receptor antagonists that enhanced the antinociceptive effect of morphine and rescued morphine tolerance, Eur. J. Med. Chem., 2023, 249, 115178–115180 CrossRef CAS;
(d)
Y. Hu, D. Wu, W. Peng, L. Lu, X. Li, X. Zhang, R. Wang, S. Zhu, B. Huang, T. Liu, J. Zhu and Y. Wu, Heterocyclic compound, application and pharmaceutical composition and application, WO2020114474A1, 2020;
(e)
M. A. Khan, Preparation of spiro-lactam NMDA receptor modulators for the treatment of depression and related disorders, WO2018026763, 2018.
- For selected reports on the synthesis of the 2,6-diazaspiro[3.4]octane skeleton, see:
(a) M. Johner, G. Rihs, S. Gürtler and H.-H. Otto, Monocarboxylates of 3-Methylidene-β-lactams: Synthesis and unusual oxidative rearrangement into a spiro[azetidine-3,3′-pyrrolidine] derivative, Helv. Chim. Acta, 1994, 77, 2147–2152 CrossRef CAS;
(b) D. Orain, S. Hintermann, M. Pudelko, D. Carballa and A. Jedrzejczaka, Synthesis of Orthogonally Protected 2,6-Diazaspiro[3.5]nonane and 2,6-Diazaspiro[3.4]octane Analogues as Versatile Building Blocks in Medicinal Chemistry, Synlett, 2015, 1815–1818 CAS;
(c) A. Lukin, K. Komarova, L. Vinogradova, M. Dogonadze, T. Vinogradova, P. Yablonsky, A. Kazantsev and M. Krasavin, Periphery Exploration around 2,6-Diazaspiro[3.4]octane Core Identifies a Potent Nitrofuran Antitubercular Lead, Molecules, 2023, 28, 2529–2544 CrossRef CAS PubMed;
(d) C. M. Martínez-Viturro, A. A. Trabanco, J. Royes, E. Fernández, G. Tresadern, J. A. Vega, A. del Cerro, F. Delgado, A. G. Molina, F. Tovar, P. Shaffer, A. Ebneth, A. Bretteville, L. Mertens, M. Somers, J. M. Alonso and J. M. Bartolomé-Nebreda, Diazaspirononane Nonsaccharide Inhibitors of O-GlcNAcase (OGA) for the Treatment of Neurodegenerative Disorders, J. Med. Chem., 2020, 63, 14017–14044 CrossRef;
(e) M. Wang, T. Tang, R. Li, Z. Huang, D. Ling, L. Zheng, Y. Ding, T. Liu, W. Xu, F. Zhu, H. Min, R. Boonhok, F. Mao, J. Zhu, X. Li, L. Jiang and J. Li, Drug Repurposing of Quisinostat to Discover Novel Plasmodium falciparum HDAC1 Inhibitors with Enhanced Triple-Stage Antimalarial Activity and Improved Safety, J. Med. Chem., 2022, 65, 4156–4181 CrossRef CAS PubMed.
- For reviews about Staudinger cycloaddition, see:
(a) B. Alcaide, P. Almendros and C. Aragoncillo, β-Lactams: Versatile Building Blocks for the Stereoselective Synthesis of Non-β-Lactam Products, Chem. Rev., 2007, 107, 4437–4492 CrossRef CAS;
(b) F. P. CossÍo, A. Arrieta and M. A. Sierra, The Mechanism of the Ketene–Imine (Staudinger) Reaction in Its Centennial: Still an Unsolved Problem, Acc. Chem. Res., 2008, 41, 925–936 CrossRef. For a book and selected examples, see:
(c)
M. Shi, Y. Wei, M.-X. Zhao and J. Zhang, Organocatalytic Cycloadditions for Synthesis of Carbo- and Heterocycles, Wiley-VCH Verlag Gmbh & Co. KgaA, 2018 CrossRef;
(d) Y.-R. Zhang, L. He, X. Wu, P.-L. Shao and S. Ye, Chiral N-Heterocyclic Carbene Catalyzed Staudinger Reaction of Ketenes with Imines: Highly Enantioselective Synthesis of N-Boc β-Lactams, Org. Lett., 2008, 10, 277–280 CrossRef CAS PubMed;
(e) M. He and J. W. Bode, Enantioselective, NHC-Catalyzed Bicyclo-β-Lactam Formation via Direct Annulations of Enals and Unsaturated N-Sulfonyl Ketimines, J. Am. Chem. Soc., 2008, 130, 418–419 CrossRef CAS PubMed.
- M. Kinugasa and S. Hashimoto, The reactions of copper(I) phenylacetylide with nitrones, J. Chem. Soc., Chem. Commun., 1972, 466–467 RSC.
- M. Miura, M. Enna, K. Okura and M. Nomura, Copper-Catalyzed Reaction of Terminal Alkynes with Nitrones. Selective Synthesis of 1-Aza-1-buten-3-yne and 2-Azetidinone Derivatives, J. Org. Chem., 1995, 60, 4999–5004 CrossRef CAS.
- For reviews about the Kinugasa reaction, see:
(a) R. K. Khangaraot and K. P. Kaliappan, Kinugasa Reaction: A Direct One-Pot Route to Highly Functionalized β-Lactams, Eur. J. Org. Chem., 2013, 7644–7677 Search PubMed;
(b) S. Stecko, B. Furman and M. Chmielewski, Kinugasa reaction: an ‘ugly duckling’ of β-lactam chemistry, Tetrahedron, 2014, 70, 7817–7844 CrossRef CAS.
- For selected examples of racemic Kinugasa reactions, see:
(a) K. Okuro, M. Enna, M. Miura and M. Nomura, Copper-catalysed reaction of arylacetylenes with C,N-diarylnitrones, J. Chem. Soc., Chem. Commun., 1993, 1107–1108 RSC;
(b) C. S. McKay, D. C. Kennedy and J. P. Pezacki, Studies of multicomponent Kinugasa reactions in aqueous media, Tetrahedron Lett., 2009, 50, 1893–1896 CrossRef CAS;
(c) A. Basak, K. Chandra, R. Pal and S. C. Ghosh, Kinugasa Reaction under Click Chemistry Conditions, Synlett, 2007, 1585–1588 CrossRef CAS.
- For selected examples of asymmetric Kinugasa reactions, see:
(a) M. M.-C. Lo and G. C. Fu, Cu(I)/Bis(azaferrocene)-Catalyzed Enantioselective Synthesis of β-Lactams via Couplings of Alkynes with Nitrones, J. Am. Chem. Soc., 2002, 124, 4572–4573 CrossRef CAS PubMed;
(b) M.-C. Ye, J. Zhou, Z.-Z. Huang and Y. Tang, Chiral tris(oxazoline)/Cu(II) catalyzed coupling of terminal alkynes and nitrones, Chem. Commun., 2003, 2554–2255 RSC;
(c) M.-C. Ye, J. Zhou and Y. Tang, Trisoxazoline/Cu(II)-Promoted Kinugasa Reaction. Enantioselective Synthesis of β-Lactams, J. Org. Chem., 2006, 71, 3576–3582 CrossRef CAS;
(d) Z. Chen, L. Lin, M. Wang, X. Liu and X. Feng, Asymmetric Synthesis of trans-β-Lactams by a Kinugasa Reaction on Water, Chem. – Eur. J., 2013, 19, 7561–7567 CrossRef CAS;
(e) Y. Takayama, T. Ishii, H. Ohmiya, T. Iwai, M. C. Schwarzer, S. Mori, T. Taniguchi, K. Monde and M. Sawamura, Asymmetric Synthesis of β-Lactams through Copper-Catalyzed Alkyne–Nitrone Coupling with a Prolinol–Phosphine Chiral Ligand, Chem. – Eur. J., 2017, 23, 8400–8404 CrossRef CAS;
(f) B. Baeza, L. Casarrubios and M. A. Sierra, Towards a General Synthesis of 3-Metal-Substituted β-Lactams, Chem. – Eur. J., 2013, 19, 11536–11540 CrossRef CAS;
(g) M. Michalak, M. Stodulski, S. Stecko, A. Mames, I. Panfil, M. Soluch, B. Furman and M. Chmielewski, A Formal Synthesis of Ezetimibe via Cycloaddition/Rearrangement Cascade Reaction, J. Org. Chem., 2011, 76, 6931–6936 CrossRef CAS PubMed;
(h) B. Grzeszczyk, K. Poławska, Y. M. Shaker, S. Stecko, A. Mames, M. Woźnica, M. Chmielewski and B. Furman, Asymmetric Kinugasa reaction involving six-membered cyclic nitrones, Tetrahedron, 2012, 68, 10633–10639 CrossRef CAS.
-
(a) L. K. Ding and W. J. Irwin, cis- and trans-Azetidin-2-ones from nitrones and copper acetylide, J. Chem. Soc., Perkin Trans. 1, 1976, 2382–2386 RSC;
(b) S. Santoro, R.-Z. Liao, T. Marcelli, P. Hammar and F. Himo, Theoretical Study of Mechanism and Stereoselectivity of Catalytic Kinugasa Reaction, J. Org. Chem., 2015, 80, 2649–2660 CrossRef CAS PubMed;
(c) T. C. Malig, D. Yu and J. E. Hein, A Revised Mechanism for the Kinugasa Reaction, J. Am. Chem. Soc., 2018, 140, 9167–9173 CrossRef CAS PubMed;
(d) S. Santoro and F. Himo, Mechanism of the Kinugasa Reaction Revisited, J. Org. Chem., 2021, 86, 10665–10671 CrossRef CAS PubMed.
- For reviews about organocopper(I) intermediate trapping, see:
(a) F. Brunelli, C. Russo, M. Giustiniano and G. C. Tron, Each Interruption is an Opportunity: Novel Synthetic Strategies Explored Through Interrupted Click Reactions, Chem. – Eur. J., 2024, 30, e202303844 CrossRef CAS;
(b) K. Qiu, J. Li, H. Ma, W. Zhou and Q. Cai, Recent Advances in the Construction of Nitrogen-Containing Heterocycles via Trapping Organocopper(I) Intermediates, Acta Chim. Sin., 2023, 81, 42–63 CrossRef CAS;
(c) F. Wei, W. Wang, Y. Ma, C.-H. Tung and Z. Xu, Regioselective synthesis of multisubstituted 1,2,3-triazoles: moving beyond the copper-catalyzed azide–alkyne cycloaddition, Chem. Commun., 2016, 52, 14188–14199 RSC.
- R. Shintani and G. C. Fu, Catalytic Enantioselective Synthesis of β-Lactams: Intramolecular Kinugasa Reactions and Interception of an Intermediate in the Reaction Cascade, Angew. Chem., Int. Ed., 2003, 42, 4082–4085 CrossRef CAS PubMed.
- T. Shu, L. Zhao, S. Li, X.-Y. Chen, C. von Essen, K. Rissanen and D. Enders, Asymmetric Synthesis of Spirocyclic β-Lactams through Copper Catalyzed Kinugasa/Michael Domino Reactions, Angew. Chem., Int. Ed., 2018, 57, 10985–10988 CrossRef CAS PubMed.
-
(a) J. Qi, F. Wei, S. Huang, C.-H. Tung and Z. Xu, Copper(I)-Catalyzed Asymmetric Interrupted Kinugasa Reaction: Synthesis of α-Thiofunctional Chiral β-Lactams, Angew. Chem., Int. Ed., 2021, 60, 4561–4565 CrossRef CAS;
(b) J. Qi, F. Wei, C.-H. Tung and Z. Xu, Modular Synthesis of α-Quaternary Chiral β-Lactams by a Synergistic Copper/Palladium-Catalyzed Multicomponent Reaction, Angew. Chem., Int. Ed., 2021, 60, 13814–13818 CrossRef CAS;
(c) J. Qi, C.-H. Tung and Z. Xu, Interrupted Kinugasa allylic alkylation, Trends Chem., 2021, 3, 984–985 CrossRef CAS;
(d) J. Qi, T. Song, Z. Yang, S. Sun, C.-H. Tong and Z. Xu, Simultaneous Dual Cu/Ir Catalysis: Stereodivergent Synthesis of Chiral β-Lactams with Adjacent Tertiary/Quaternary/Tertiary Stereocenters, ACS Catal., 2023, 13, 2555–2564 CrossRef CAS.
- A. Torelli, E. S. Choi, A. Dupeux, M. N. Perner and M. Lautens, Stereoselective Kinugasa/Aldol Cyclization: Synthesis of Enantioenriched Spriocyclic β-Lactams, Org. Lett., 2023, 25, 8520–8525 CrossRef CAS PubMed.
-
(a) X. Zhong, M. Huang, H. Xiong, Y. Liang, W. Zhou and Q. Cai, Asymmetric Synthesis of Spiro[Azetidine-3,3′-Indoline]-2,2′-Diones via Copper(I)-Catalyzed Kinugasa/C–C Coupling Cascade Reaction, Angew. Chem., Int. Ed., 2022, e202208323 CAS;
(b) J. Li, H. Ma, X. Zhong, S. Li, J. Zhang, Y. Ao, W. Zhou and Q. Cai, Highly diastereo- and enantioselective synthesis of spiro β-lactams via copper-catalyzed Kinugasa/aldol cascade reaction, Org. Chem. Front., 2023, 10, 5383–5388 RSC;
(c) Y. Ao, H. Ma, B. Gan, W. Wang, J. Zhang, W. Zhou, X. Zhang and Q. Cai, Copper-Catalyzed Asymmetric Kinugasa/Michael Addition Cascade Reactions for the Sythesis of Chiral Sprio β-lactams, Org. Lett., 2024, 26, 4761–4766 CrossRef CAS PubMed.
- For selected examples of Conia-ene reactions, see:
(a) N. Monteiro, G. Balme and J. Gore, Copper-Promoted Cyclisation of α-sulfonyl ε-Acetylenic Esters: A Route to Allylic Cyclopentanic Sulfones, Synlett, 1992, 227–228 CrossRef CAS;
(b) F. W. W. Hartrampf, T. Furukawa and D. Trauner, A Conia-Ene-Type Cyclization under Basic Conditions Enables an Efficient Synthesis of (−)-Lycoposerramine R, Angew. Chem., Int. Ed., 2017, 56, 893–896 CrossRef CAS;
(c) C. Kourra, F. Klotter, F. Sladojevich and D. J. Dixon, Alkali Base-Initiated Michael Addition/Alkyne Carbocyclization Cascades, Org. Lett., 2012, 14, 1016–1019 CrossRef CAS PubMed;
(d) O. Kitagawa, T. Suzuki, H. Fujiwara, M. Fujita and T. Taguchi, Alkaline Metallic Reagent-Catalyzed Hydrocarbocyclization Reaction of Various Active Methine Compounds Having an Unactivated 4-Alkynyl or Allenyl Group, Tetrahedron Lett., 1999, 40, 4585–4588 CrossRef CAS.
- Other single solvents such as THF and 1,4-dioxane delivered a trace amount of desired product 3aa.
- For more details about condition screening, see the ESI.†.
- CCDC 2371699 (3aa) and 2371702 (3aa′) contain the supplementary crystallographic data for this paper.†.
|
This journal is © the Partner Organisations 2025 |
Click here to see how this site uses Cookies. View our privacy policy here.