DOI:
10.1039/D4QO01546K
(Research Article)
Org. Chem. Front., 2025,
12, 76-84
HSAB theory guiding electrophilic substitution reactions of o-carborane†
Received
22nd August 2024
, Accepted 25th October 2024
First published on 26th October 2024
Abstract
Although the three-dimensional aromatic character of carboranes has been well-known for a long time and their electrophilic substitution reactions have been studied, systematic comparison of the differences and similarities of these reactions with aromatic compounds has not been explored. In the electrophilic substitution reaction, the electrophiles act as Lewis acids to accept electrons, and the aromatic compounds and carboranes act as Lewis bases to donate electrons. Therefore, the HSAB (hard and soft, acids and bases) theory can principally predict the outcome of such reactions. Herein, we systematically compared the reactivity of o-carborane and benzene toward different electrophiles to conclude that hard electrophiles prefer to react with benzene and soft electrophiles prefer o-carborane, which is consistent with the HSAB principle. Directed by this finding, we developed the electrophilic selenylation, oxidation, electrophilic mercuration, and Pd(II)-catalyzed B(9)–H bond halogenation, sulfuration, and amination of o-carboranes. We believe this finding will have a guiding significance for the selective B–H functionalization of carboranes.
Introduction
Carboranes, a class of polyhedral boron–carbon molecular clusters that are stabilized by electron-delocalized covalent bonding in the skeletal framework, have been widely used in many fields, including optoelectronics,1–5 catalysis,6–11 supramolecular design,12–15 organometallic coordination chemistry,16–20 and pharmaceuticals.21–25 Further development of these applications relies on more efficient synthetic methods and selective functionalization of the carborane framework. Generally, the hydrogen of the C–H bond of carboranes displays acidity so that the C–H bond can be easily functionalized with strong bases.26–28 In contrast, the polarity of the B–H bond of carboranes is weak, so the B–H bond activation is relatively difficult. Therefore, the selective B–H functionalization of carboranes has attracted much attention in recent years.29–33
Electrophilic aromatic substitution reaction, one of the most classical reactions of aromatic compounds, has been widely used in organic syntheses.34,35 On the other hand, the electrophilic substitution reaction of carboranes to realize the selective B–H functionalization has also been explored since the carboranes were discovered because carboranes exhibit three-dimensional σ-aromaticity due to electron-delocalization and are often considered as 3D analogs of benzene.36–42
However, compared with aromatics, the studies on the electrophilic substitution reactions of carboranes are still very limited. Additionally, the differences and similarities of the reactivity of carboranes and aromatics to different electrophiles have not been studied systematically.
Recently, we developed practical methods for the selective B(9)–H functionalization of o/m-carboranes and achieved the electrophilic halogenation43,44 and amination,45 hydroxylation,46 and the Pd(II)-catalyzed B–H amination47 and oxidative dehydrogenation coupling of carboranes to bis(carborane)s.48 In these transformations, the substrate 1,2-diphenyl-o-carborane was explored. Interestingly, the electrophilic halogenation and Pd(II)-catalyzed B(9)–H amination prefer to occur on the carboranyl (Scheme 1a and b). In contrast, the electrophilic nitration prefers to react with the phenyl (Scheme 1c). Additionally, the amination products of both the B–H and C–H bonds were detected in the electrophilic amination with diethyl azodicarboxylate (Scheme 1d). These results illustrated that the reactivity of carboranes and aromatics was related to the properties of electrophiles.
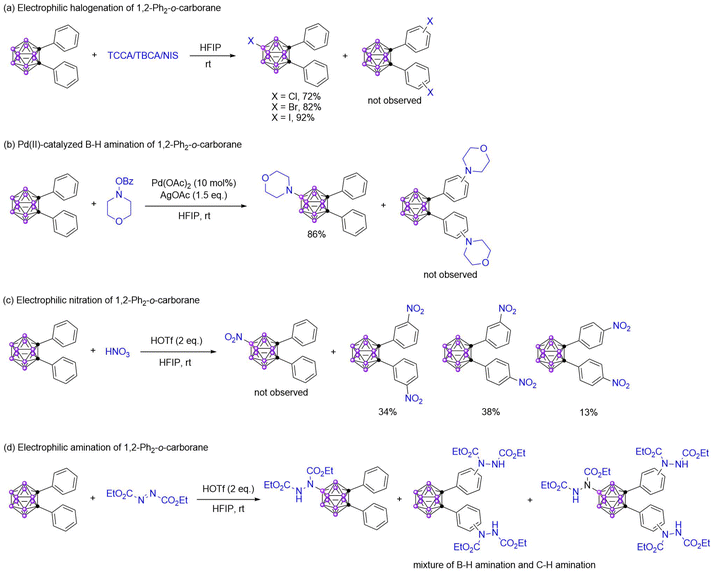 |
| Scheme 1 Chemoselective functionalization of 1,2-diphenyl-o-carborane. | |
The Pd(II)-catalyzed selective B(8,9,10,12)–H tetrafluorination reaction of o-carborane was reported by Xie's group.49 In their transformations, the substrates with aryl substituents gave the desired B–H bond functionalization products on the boron cluster in high yields, and no C–H bond functionalization on aryl was observed. Later, Cao's group achieved the Pd(II)-catalyzed B(8)/B(9)–H arylation,50 alkenylation51 and acetoxylation52 of o-carboranes. Similarly, the functionalization only occurred on the B–H bond, and no C–H bond functionalization on the aryl group was detected for the substrates with a phenyl substituent. In these works, the authors pointed out that the electrophilic palladation of o-carborane to form a B–Pd bond was involved. These results suggested that the reactivity of electrophilic palladation of o-carborane is much higher than that of benzene. Acids such as trifluoroacetic acid (TFA) and trifluoromethanesulfonic acid (HOTf) could increase the electrophilicity of Pd(II), subsequently, the Pd(II)-catalyzed directly C–H activation of aromatic through electrophilic palladation process has been achieved.53 Nonetheless, the Pd(II)-catalyzed directly B–H functionalization of o-carboranes can be easily achieved under mild conditions.47
Herein, we systematically compared the reactivity of o-carborane and benzene towards different electrophiles and explained the results with the HSAB principle. Electrophilic substitution reactions can be regarded as the Lewis acid–base reactions, where electrophiles can be considered as the Lewis acids to accept electrons, and aromatics as Lewis bases to donate electrons. The results indicated that the reactivity of the electrophilic substitution reactions of carboranes and aromatics is consistent with the HSAB theory, e.g., hard electrophiles (hard Lewis acids) prefer to react with aromatics (hard Lewis bases), and vice versa, soft electrophiles (soft Lewis acids) prefer to carboranes (soft Lewis bases). Guided by these results, we established a series of electrophilic substitution reactions and Pd(II)-catalyzed B(9)–H functionalization reactions of o-carborane.
Results and discussion
Comparison of the reported electrophilic substitution reactions of o-carborane and benzene
The carbon atom is slightly more electronegative than the boron atom, and the carbon atoms contribute more electrons to cluster bonding than the boron atoms, which results in the carbon atoms effectively being electron-deficient. Thus, the o-carborane is a polar molecule with a net dipole moment of 4.45, pointing away from two carbon vertices.54 Such electron structural features result in the BH vertices, especially the B(9)H and B(8)H, readily occurring electrophilic substitution reactions. The less electronegative of the boron atom than the carbon atom also makes the boron vertices on the carboranes softer than the carbon vertices on the benzene. Additionally, the valence electrons of carborane are more delocalized in the three-dimensional system than those of benzene ring in the two-dimensional system. Therefore, in these electrophilic reactions, the BH vertices in the carborane act as nucleophilic sites and display a soft Lewis bases character, compared with the benzene ring, prior reactions with soft Lewis acids.
To verify our hypothesis, we analyzed and compared the reactivity of benzene and o-carborane towards different electrophiles based on our previous reports.43–46 Firstly, the electrophilic halogenation of benzene and o-carborane with TCCA, TBCA, and NIS as the electrophiles in 1,1,1,3,3,3-hexafluoro-2-propanol (HFIP) was explored under catalyst-free conditions (Scheme 2a).43,45,55,56 The results indicated that bromination and iodination prefer to occur at o-carborane and chlorination at benzene. When HOTf was used as a catalyst, the proportion of bromination on benzene increased (Scheme 2b), because the electrophilicity of NBS increased when HOTf was added and sped up the reaction rate, thus the selectivity was reduced. These results were consistent with the HSAB principle that soft Lewis acids NIS, NBS, and TBCA prefer to attack soft Lewis base o-carborane, and hard Lewis acid TCCA prefers to hard Lewis base benzene. Then, the electrophilic nitration with HNO3 as the electrophile was examined, and the results indicated that the reactivity of benzene was higher than that of o-carborane, as expected (Scheme 2c).46,57 When the electrophile was changed to DEAD, the electrophilic substitution reaction was also preferred to occur at the benzene,51,58 which is also in line with the HSAB principle (Scheme 2d). The Friedel–Crafts alkylation of carboranes with benzyl alcohols as the electrophiles has been reported by Yan's group.59 Under similar reaction conditions, the Friedel–Crafts alkylation of aromatic compounds was achieved.60 On the basis of these reports, we conducted the competing reaction of o-carborane and benzene towards pentafluorobenzyl alcohol, which indicated that benzene has a higher reactivity than o-carborane (Scheme 2e).
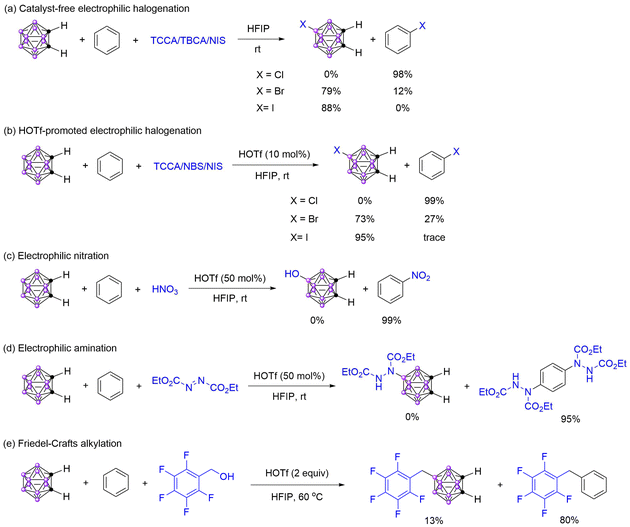 |
| Scheme 2 Comparison of electrophilic substitution reactions between o-carborane and benzene. | |
Study on the electrophilic chalcogenation/Friedel–Crafts acylation/sulfonylation
To further support our proposal, we studied the electrophilic chalcogenation of o-carborane (Scheme 3a). Firstly, the electrophilic sulfuration of o-carborane was explored with 1-(phenylthio)pyrrolidine-2,5-dione as the electrophile, and the desired product 1a was obtained in 10% yield in the presence of 2 equiv. HOTf in HFIP. Under similar reaction conditions, the electrophilic selenylation of o-carborane was established with phenylselenenyl chloride as the electrophile, and the corresponding product 2a was obtained in 94% yield at room temperature. Then the competitive experiments of benzene and o-carborane towards these two electrophiles were conducted, which indicated that the reactivity of benzene was higher than o-carborane towards 1-(phenylthio)pyrrolidine-2,5-dione,61 in contrast, the reactivity of o-carborane was slightly higher than benzene towards electrophile phenylselenenyl chloride. The results of the electrophilic chalcogenation were consistent with the HSAB theory.
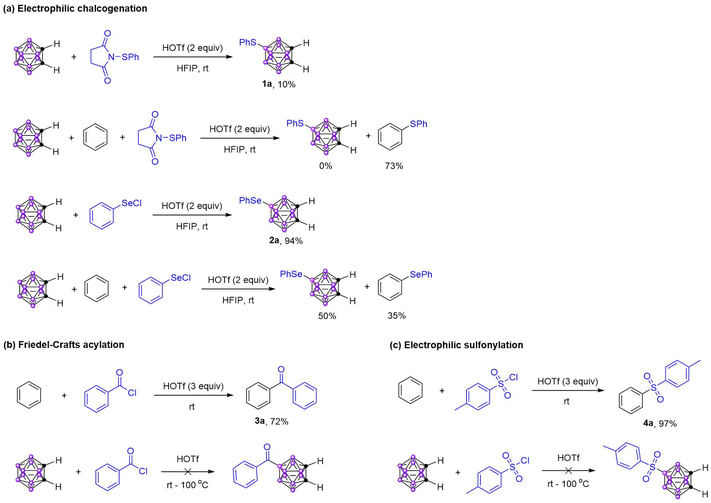 |
| Scheme 3 Electrophilic chalcogenation, Friedel–Crafts acylation and sulfonylation. | |
The Friedel–Crafts acylation reaction is one of the most powerful tools for the synthesis of aromatic ketones. Therefore, the Friedel–Crafts acylation of arenes has been widely explored in organic synthesis.62 However, the Friedel–Crafts acylation of carboranes has not been reported so far. Recently, we found that the HOTf-promoted electrophilic aromatic substitution reactions have a high reactivity under metal-free conditions. Based on this, we achieved the Friedel–Crafts acylation of benzene and obtained the desired product 3a in 72% yield (Scheme 3b). No corresponding acylation product of o-carborane was detected even with HOTf as the solvent at 100 °C. Obviously, the reactivity of benzene is much higher than that of o-carborane towards electrophile acyl cation.
Electrophilic sulfonylation is also an important part of electrophilic aromatic substitution reactions. Under similar reaction conditions with Friedel–Crafts acylation, the sulfonylation of benzene was achieved to provide sulfone 4a in 97% yield (Scheme 3c), and no product was detected when o-carborane was used as the substrate.
These results suggested that the acylation and sulfonylation of carboranes were difficult to achieve through the electrophilic substitution process as the acyl cation and sulfonyl cation are hard Lewis acids, resulting in no direct B–H acylation or sulfonylation of carboranes reported.
Electrophilic metallization
Electrophilic metallization of arenes is one part of electrophilic aromatic substitution reactions, including electrophilic auration,63 mercuration,64 thallation,65 and plumbation.66 The electrophilic mercuration67 and thallation68 of carboranes have also been reported. Based on our previous reports, the electrophilic auration, mercuration, and thallation of o-carborane were next explored.
Firstly, the electrophilic thallation was studied (Scheme 4a). With thallium(III) trifluoroacetate Tl(TFA)3 as the electrophile, the electrophilic thallation of benzene in TFA has been reported.65 However, trace amounts of B–O coupling products 9-CF3COO-o-carborane 5a were detected for the reaction of o-carborane with Tl(TFA)3 under the same reaction conditions. To increase the electrophilicity of Tl(TFA)3, 6 equiv. HOTf was added to the reaction system, and the products 5a, 5b and 5c were obtained in 35%, 50%, and 10% yields, respectively. Changing the solvent from TFA to HFIP, only 5c was isolated in 79% yield. We think the B–Tl intermediate int 1 should be initially formed through electrophilic thallation, and further reductive elimination provided the B–O coupling product 5c. These results suggested that the reactivity of benzene is higher than o-carborane towards electrophile Tl(TFA)3.
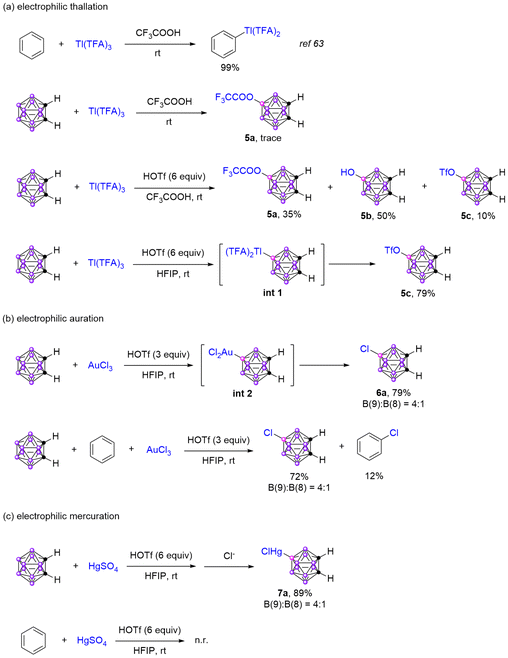 |
| Scheme 4 Electrophilic metallization. | |
Next, the electrophilic auration was examined (Scheme 4b). Employing AuCl3 as the electrophilic reagent, 79% chlorocarborane with a 4
:
1 ratio of 9-Cl-o-carborane and 8-Cl-o-carborane was obtained in HFIP when 3 equiv. HOTf was added to the reaction system. Similarly, the B–Au intermediate int 2 should be initially formed, which further transformed to chlorocarborane 6a after reductive elimination. Subsequently, the competitive reaction between o-carborane and benzene towards AuCl3 was conducted, revealing the formation of 72% chlorocarborane and 12% chlorobenzene. This outcome signifies a slight preference of o-carborane over benzene towards AuCl3.
Finally, the electrophilic mercuration was investigated (Scheme 4c). With HgSO4 as the electrophile and HFIP as the solvent, the desired 9-HgCl-o-carborane 7a was isolated in 89% yield after anion exchange when 6 equiv. HOTf was added to the reaction mixture. In contrast, no reaction was detected when benzene was used as the substrate under the same reaction conditions, which suggested that o-carborane has a higher reactivity than benzene towards HgSO4.
We provided the chemical hardness (η) of these three metal cations in Table 1, which suggested that the hardness of them is Tl3+ (10.4) > Au3+ (8.4) > Hg2+ (7.7).69,70 We also summarized the results of the electrophilic substitution reactions of these three metal cations with o-carborane and benzene, which is consistent with the HSAB theory, that hard metal ions prefer to react with benzene, and vice versa soft metal ions prefer to o-carborane.
Table 1 Chemical hardness and reactivity of Tl3+, Au3+ and Hg2+
E |
Tl3+ |
Au3+ |
Hg2+ |
η
|
10.4 |
8.4 |
7.7 |
Reactivity |
Benzene > o-carborane |
Benzene < o-carborane (slightly) |
Benzene < o-carborane |
Proposal for Pd(II)-catalyzed B(9)–H functionalization of o-carborane
The chemical hardness of Pd(II) is 6.75, which is softer than Hg(II), Au(III), and Tl(III),69,70 based on which, the reactivity of the electrophilic palladation of o-carborane should be much higher than that of benzene. Actually, the Pd(II)-catalyzed B(9)–H activation of o-carborane can be easily achieved in HFIP. However, the direct C–H activation of benzene through the electrophilic palladation process is only possible under strong acidic conditions.53 We speculate this is because the electrophilic palladation of o-carborane to form the B–Pd bond can be easily achieved, which can be further transformed into functionalized o-carboranes. In contrast, the electrophilic palladation of benzene cannot be achieved under similar reaction conditions, making the directly Pd(II)-catalyzed C–H activation of benzene almost impossible. Based on these results, the Pd(II)-catalyzed B(9)–H functionalization of o-carborane was proposed.
Directing group assisted Pd(II)-catalyzed B–H halogenation of o-carboranes and C–H halogenation of arenes with N-haloamides as the halogenated reagents have been reported. In these transformations, a B–Pd or C–Pd intermediate was generated through B–H or C–H bond activation and further reacted with N-haloamide to form a Pd(IV) intermediate, subsequently reductive elimination to give the desired halogenated products.71 So we proposed that the B(9)–H halogenation of o-carborane should also be achieved by Pd(II)-catalyzed B–H activation in HFIP. To verify our hypothesis, the Pd(II)-catalyzed B(9)–H halogenation of o-carborane was investigated (Table S1†). In the absence of Pd(OAc)2, no product was observed when o-carborane reacted with N-chlorosuccinimide (NCS) in HFIP at room temperature (Table S1,† entry 1). Upon the introduction of Pd(OAc)2, enhanced reactivity was achieved, leading to the formation of the B(9)–H chlorination product 9-Cl-o-carborane 6a in 36% yield, along with a trace amount of 9,12-Cl2-o-carborane (Table S1,† entry 2). Utilizing N-bromosuccinimide (NBS) as the electrophile, a 26% yield of 8a was isolated without the presence of Pd(OAc)2, and the yield of 8a was increased to 69% when Pd(OAc)2 was introduced to the reaction system (Table S1,† entries 3 and 4). In contrast, the Pd(OAc)2 has little influence on the B(9)–H iodination, as the directly electrophilic iodination has a high reactivity towards o-carborane (Table S1,† entries 5 and 6). Based on these initial findings, we compared the reactivity of benzene and o-carborane towards halogenated reagents NCS, NBS, and NIS under Pd(OAc)2 catalysis (Scheme 5a). Different from the results that in the absence of Pd(OAc)2, all the halogenated reagents were reacted with o-carborane, as the Pd(II) is a soft Lewis acid and will have a higher reactivity towards o-carborane over benzene.
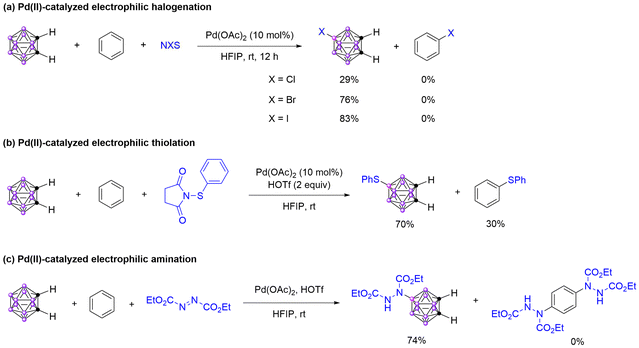 |
| Scheme 5 Comparison of Pd(II)-catalyzed B–H/C–H activation of o-carborane and benzene. | |
Based on the above results and literature reports,72 we conducted the competitive experiment between o-carborane and benzene towards electrophile 1-(phenylthio)pyrrolidine-2,5-dione under Pd(II) catalyst. As a result, the ratio of the B–H thiolation product was increased compared with the reaction that in the absence of Pd(OAc)2, but the C–H thiolation product was still obtained in 30% yield (Schemes 3a and 5b). We infer that the C–H thiolation product should be formed through a direct electrophilic thiolation of benzene, not the Pd(II)-catalyzed C–H activation. The proposed mechanism of Pd(II)-catalyzed B(9)–H halogenation and thiolation of o-carborane was depicted in the ESI (Scheme S1†). The electrophilic palladation to form a B–Pd intermediate was the key to this transformation, and the benzene cannot occur the same transformation to produce a C–Pd intermediate under the same reaction conditions.
The HOTf-promoted electrophilic amination of o-carboranes has been developed in the absence of transition metals.43 However, only some electron-rich o-carboranes were compatible with this transformation. We wonder that whether this transformation can be achieved through Pd(II)-catalyzed B–H activation. To our delight, the yield increased greatly after Pd(OAc)2 was added to the reaction system, and some electron-deficient carboranes were successfully transformed into corresponding aminated products (Table S3†). We also compared the reactivity of o-carborane and benzene under Pd(OAc)2 catalysis. In contrast with the result that in the absence of Pd(OAc)2, the amination prefers to react with o-carborane in the presence of Pd(OAc)2, and no aminated product of benzene was detected (Schemes 2d and 5c). The possible reaction mechanism was proposed, and an electrophilic palladation process was involved (Scheme S2†).
All the above results suggested that o-carborane has a higher reactivity than benzene towards Pd(II)-catalyzed reactions. As the electrophilic palladation of o-carborane can be easily achieved under mild reaction conditions, the Pd(II)-catalyzed B–H activation shows an excellent application value in the selective B–H functionalization of carboranes. Other Pd(II)-catalyzed B(9)–H functionalization of o-carborane is underway in our laboratory.
Conclusions
In summary, we have systematically analyzed and compared the reactivity of o-carborane and benzene towards different electrophiles and found that the reactivity of such reactions followed the HSAB principle. The electrophiles can be considered as Lewis acids, and the benzene or o-carborane as Lewis bases in these reactions, in which soft electrophiles prefer to react with o-carborane and hard electrophiles prefer the benzene. As the Pd(II) is a much softer Lewis acid, the reactivity for the electrophilic palladation of o-carborane is much higher than benzene, resulting in the Pd(II)-catalyzed direct B(9)–H bond activation of o-carborane can be achieved under mild reaction conditions. Guided by these results, a series of electrophilic substitution reactions and Pd(II)-catalyzed B(9)–H functionalization of o-carboranes were realized. A comprehensive understanding of the differences and similarities between carboranes and aromatics in the electrophilic substitution reactions will inspire new synthetic methods for the selective B–H functionalization of carboranes.
Author contributions
Y.-N. M and X. C. conceived and designed the study. Y. W., Y.-G. L. and F. C. performed the experiments and analyzed the data. All authors discussed the results and commented on the manuscript.
Data availability
Data for all compounds in this manuscript are available in the ESI,† which includes experimental details, characterisation data, and copies of 1H, 13C, 11B NMR spectra. The data supporting this article have been included as part of the ESI.†
Conflicts of interest
The authors declare no competing interests.
Acknowledgements
This work is supported by the National Natural Science Foundation of China (Grant No. 22271256 to Y.-N. M. and U23A2078 to X. C.), and Natural Science Foundation of Henan Province (232300421088 to Y.-N. M.).
References
- R. Núñez, M. Tarrés, A. Ferrer-Ugalde, F. F. de Biani and F. Teixidor, Electrochemistry and Photoluminescence of Icosahedral Carboranes, Boranes, Metallacarboranes, and Their Derivatives, Chem. Rev., 2016, 116, 14307–14378 CrossRef.
- J. Ochi, K. Tanaka and Y. Chujo, Recent Progress in the Development of Solid-State Luminescent o-Carboranes with Stimuli Responsivity, Angew. Chem., Int. Ed., 2020, 59, 9841–9855 CrossRef CAS.
- X. Wei, M.-J. Zhu, Z. Cheng, M. Lee, H. Yan, C. Lu and J.-J. Xu, Aggregation-Induced Electrochemiluminescence of Carboranyl Carbazoles in Aqueous Media, Angew. Chem., Int. Ed., 2019, 58, 3162–3166 CrossRef CAS PubMed.
- L. Wu, M. Holzapfel, A. Schmiedel, F. Peng, M. Moos, P. Mentzel, J. Shi, T. Neubert, R. Bertermann, M. Finze, M. A. Fox, C. Lambert and L. Ji, Optically Induced Charge-Transfer in Donor-Acceptor-Substituted p- and m- C2B10H12 Carboranes, Nat. Commun., 2024, 15, 3005 CrossRef CAS PubMed.
- A. D. Ready, Y. A. Nelson, D. F. T. Pomares and A. M. Spokoyny, Redox-Active Boron Clusters, Acc. Chem. Res., 2024, 57, 1310–1324 CrossRef CAS.
- Q. Wu, E. Irran, R. Müller, M. Kaupp, H. F. T. Klare and M. Oestreich, Characterization of Hydrogen-Substituted Silylium Ions in the Condensed Phase, Science, 2019, 365, 168–172 CrossRef CAS.
- S. O. Gun-ther, Q. H. Lai, T. Senecal, R. Huacuja, S. Bremer, D. M. Pearson, J. C. DeMott, N. Bhuvanesh, O. V. Ozerov and J. Klosin, Highly Efficient Carborane-Based Activators for Molecular Olefin Polymerization Catalysts, ACS Catal., 2021, 11, 3335–3342 CrossRef CAS.
- Y. Xu, Y. Yang, Y. Liu, Z. Li and H. Wang, Boron-Catalysed Hydrogenolysis of Unactivated C(aryl)-C(alkyl) Bonds, Nat. Catal., 2023, 6, 16–22 CrossRef CAS.
- T. He, H. F. T. Klare and M. Oestreich, Arenium-Ion-Catalysed Halodealkylation of Fully Alkylated Silanes, Nature, 2023, 623, 538–543 CrossRef CAS.
- C. N. Kona, R. Oku, S. Nakamura, M. Miura, K. Hirano and Y. Nishii, Aromatic Halogenation Using Carborane Catalyst, Chem, 2024, 10, 402–413 CAS.
- A. J. Baublis and A. M. Spokoyny, Boron Cluster Organocatalysis, Chem, 2024, 10, 29–32 CAS.
- E. A. Qian, A. I. Wixtrom, J. C. Axtell, A. Saebi, D. Jung, P. Rehak, Y. Han, E. H. Moully, D. Mosallaei, S. Chow, M. S. Mes-sina, J. Y. Wang, A. T. Royappa, A. L. Rheingold, H. D. Maynard, P. Král and A. M. Spokoyny, Atomically Precise Organomimetic Cluster Nanomolecules Assembled via Perfluoroaryl-Thiol SNAr Chemistry, Nat. Chem., 2017, 9, 333–340 CrossRef CAS PubMed.
- J. M. Stauber, E. A. Qian, Y. Han, A. L. Rheingold, P. Král, D. Fujita and A. M. Spokoyny, An Organometallic Strategy for Assembling Atomically Precise Hybrid Nanomaterials, J. Am. Chem. Soc., 2020, 142, 327–334 CrossRef CAS PubMed.
- A. Saha, E. Oleshkevich, C. Vinas and F. Teixidor, Biomimetic Inspired Core–Canopy Quantum Dots: IonsTrapped in Voids Induce Kinetic Fluorescence Switching, Adv. Mater., 2017, 29, 1704238 CrossRef PubMed.
- A. M. Cioran, A. D. Musteti, F. Teixidor, Ž Krpetić, I. A. Prior, Q. He, C. J. Kiely, M. Brust and C. Viñus, Mercaptocarborane-Capped Gold Nanoparticles: Electron Pools andIon Traps with Switchable Hydrophilicity, J. Am. Chem. Soc., 2012, 134, 212–221 CrossRef CAS PubMed.
- W.-B. Yu, P.-F. Cui, W.-X. Gao and G.-X. Jin, B-H Activation of Carboranes Induced by Late Transition Metals, Coord. Chem. Rev., 2017, 350, 300–319 CrossRef CAS.
- P.-F. Cui, Y.-J. Lin, Z.-H. Li and G.-X. Jin, Dihydrogen Bond Interaction Induced Separation of Hexane Isomers by Self-Assembled Carborane Metallacycles, J. Am. Chem. Soc., 2020, 142, 8532–8538 CrossRef CAS PubMed.
- S.-T. Guo, P.-F. Cui, X.-R. Liu and G.-X. Jin, Synthesis of Carborane-Backbone Metallacycles for Highly Selective Capture of n-Pentane, J. Am. Chem. Soc., 2022, 144, 22221–22228 CrossRef CAS PubMed.
- R. Nan, Y. Li, Z. Zhu, F. Qi and X.-Q. Xiao, Nickelacarborane-Supported Bis-N-heterocyclic Carbenes, J. Am. Chem. Soc., 2023, 145, 15538–15546 CrossRef CAS.
- X. Xu, Q. Cui, H. Chen and N. Huang, Carborane-Based Three-Dimensional Covalent Organic Frameworks, J. Am. Chem. Soc., 2023, 145, 24202–24209 CrossRef CAS PubMed.
- M. F. Hawthorne and A. Maderna, Applications of Radiolabeled Boron Clusters to the Diagnosis and Treatment of Cancer, Chem. Rev., 1999, 99, 3421–3434 CrossRef CAS PubMed.
- F. Issa, M. Kassiou and L. Rendina, Boron in Drug Discovery: Carboranes as Unique Pharmacophores in Biologically Active Compounds, Chem. Rev., 2011, 111, 5701–5722 CrossRef CAS.
- P. Stockmann, G. Marta, R. Kuhnert, M. B. Sárosi and E. Hey-Hawkins, New Keys for Old Locks: Carborane-Containing Drugs as Platforms for Mechanism-Based Therapies, Chem. Soc. Rev., 2019, 48, 3497–3512 RSC.
- A. Marfavi, P. Kavianpour and L. M. Rendina, Carboranes in Drug Discovery, Chemical Biology and Molecular Imaging, Nat. Rev. Chem., 2022, 6, 486 CrossRef.
- W. Ma, Y. Wang, Y. Xue, M. Wang, C. Lu, W. Guo, Y. Liu, D. Shu, G. Shao, Q. Xu, D. Tu and H. Yan, Molecular Engineering of AIE-Active Boron Clustoluminogens for Enhanced Boron Neutron Capture Therapy, Chem. Sci., 2024, 15, 4019–4030 RSC.
- Z. Qiu and Z. Xie, Functionalization of o-Carboranes via Carboryne Intermediates, Chem. Soc. Rev., 2022, 51, 3164–3180 RSC.
- T. Bischof, X. Guo, I. Krummenacher, L. Beßler, Z. Lin, M. Finze and H. Braunschweig, Alkene insertion reactivity of a o-carboranyl-substituted 9-borafluorene, Chem. Sci., 2022, 13, 7492–7497 RSC.
- T. Bischof, L. Beßler, I. Krummenacher, L. Erhard, H. Braunschweig and M. Finze, Construction of a Diverse Range of Boron Heterocycles via Ring Expansion of a Carboranyl-Substituted 9-Borafluorene, Chem. – Eur. J., 2023, 29, e202300210 CrossRef CAS PubMed.
- Y. Quan and Z. Xie, Controlled Functionalization of o-Carborane via Transition Metal Catalyzed B–H Activation, Soc. Rev., 2019, 48, 3660–3673 RSC.
- Z. Qiu and Z. Xie, A Strategy for Selective Catalytic B–H Functionalization of o-Carboranes, Acc. Chem. Res., 2021, 54, 4065–4079 CrossRef CAS PubMed.
- L. Yang, Z.-J. Zhang, B. B. Jei and L. Ackermann, Electrochemical Cage Activation of Carboranes, Angew. Chem., Int. Ed., 2022, 61, e202200323 CrossRef CAS PubMed.
- H. Ren, P. Zhang, J. Xu, W. Ma, D. Tu, C.-S. Lu and H. Yan, Direct B–H Functionalization of Icosahedral Carboranes via Hydrogen Atom Transfer, J. Am. Chem. Soc., 2023, 145, 7638–7647 CrossRef CAS.
- S. Xu, H. Zhang, J. Xu, W. Suo, C.-S. Lu, D. Tu, X. Guo, J. Poater, M. Solà and H. Yan, Photoinduced Selective B–H Activation of nido-Carboranes, J. Am. Chem. Soc., 2024, 146, 7791–7802 CrossRef CAS PubMed.
- S. Brunen, B. Mitschke, M. Leutzsch and B. List, Asymmetric Catalytic Friedel–Crafts Reactions of Unactivated Arenes, J. Am. Chem. Soc., 2023, 145, 15708–15713 CrossRef CAS.
- V. K. Singh, C. Zhu, C. K. De, M. Leutzsch, L. Baldinelli, R. Mitra, G. Bistoni and B. List, Taming Secondary Benzylic Cations in Catalytic Asymmetric SN1 Reactions, Science, 2023, 382, 325–329 CrossRef CAS PubMed.
-
R. N. Grimes, Carboranes, 3rd edn, Elsevier, Oxford, UK, 2016, pp. 283–502 Search PubMed.
- J. Poater, C. Viñus, M. Solà and F. Teixidor, 3D and 2D Aromatic Units Behave Like Oil and Water in the Case of Benzocarborane Derivatives, Nat. Commun., 2022, 13, 3844 CrossRef CAS PubMed.
- J. Poater, S. Escayola, A. Poater, F. Teixidor, H. Ottosson, C. Viñus and M. Solà, Single—Not Double—3D-Aromaticity in an Oxidized Closo Icosahedral Dodecaiodo-Dodecaborate Cluster, J. Am. Chem. Soc., 2023, 145, 22527–22538 CrossRef CAS PubMed.
- R. B. King, Three-Dimensional Aromaticity in Polyhedral Boranes and Related Molecules, Chem. Rev., 2001, 101, 1119–1152 CrossRef CAS PubMed.
- J. Poater, C. Viñus, I. Bennour, S. Eskayola, M. Solà and F. Teixidor, Too Persistent to Give Up: Aromaticity in Boron Clusters Survives Radical Structural Changes, J. Am. Chem. Soc., 2020, 142, 9396–9407 CrossRef CAS.
- J. Poater, M. Solà, C. Viñus and F. Teixidor, Heixidor Rule of Aromaticity Categorizes Aromatic closo Boron Hydride Clusters, Chem. – Eur. J., 2016, 22, 7437–7443 CrossRef CAS.
- J. Poater, M. Solà, C. Viñus and F. Teixidor, A Simple Link between Hydrocarbon and Borohydride Chemistries, Chem. – Eur. J., 2013, 19, 4169–4175 CrossRef CAS.
- W. Guo, C. Guo, Y.-N. Ma and X. Chen, Practical Synthesis of B(9)-Halogenated Carboranes with N-Haloamides in Hexafluoroisopropanol, Inorg. Chem., 2022, 61, 5326–5334 CrossRef.
- W. Lu, Y. Wu, Y.-N. Ma, F. Chen and X. Chen, A Method for Highly Selective Halogenation of o-Carboranes and m-Carboranes, Inorg. Chem., 2023, 62, 885–892 CrossRef CAS.
- Y. Wang, Y. Gao, W. Guo, Q. Zhao, Y.-N. Ma and X. Chen, Highly Selective electrophilic B(9)-amination of o-carborane driven by HOTf and HFIP, Org. Chem. Front., 2022, 9, 4975–4980 RSC.
- Y.-N. Ma, H. Ren, Y. Wu, N. Li, F. Chen and X. Chen, B(9)-OH-o-Carboranes: Synthesis, Mechanism, and Property Exploration, J. Am. Chem. Soc., 2023, 145, 7331–7342 CrossRef CAS PubMed.
- Y.-N. Ma, Y. Gao, Y. Ma, Y. Wang, H. Ren and X. Chen, Palladium-Catalyzed Regioselective B(9)-Amination of o-Carboranes and m-Carboranes in HFIP with Broad Nitrogen Sources, J. Am. Chem. Soc., 2022, 144, 8371–8378 CrossRef CAS PubMed.
- F. Chen, W. Guo, Y.-N. Ma and X. Chen, 9,9′-Bis-o-Carboranes: Synthesis and Exploration of Properties, Chem. Commun., 2024, 60, 614–617 RSC.
- Z. Qiu, Y. Quan and Z. Xie, Palladium-Catalyzed Selective Fluorination of o-Carboranes, J. Am. Chem. Soc., 2013, 135, 12192–12195 CrossRef CAS PubMed.
- K. Cao, Y. Huang, J. Yang and J. Wu, Palladium Catalyzed Selective mono-Arylation of o-Carboranes via B–H Activation, Chem. Commun., 2015, 51, 7257–7260 RSC.
- J. Wu, K. Cao, T.-T. Xu, X.-J. Zhang, L. Jiang, J. Yang and Y. Huang, Palladium Catalyzed Regioselective mono-Alkenylation of o-Carboranes via Heck Type Coupling Reaction of a Cage B–H Bond, RSC Adv., 2015, 5, 91683–91685 RSC.
- K. Cao, T.-T. Xu, J. Wu, L. Jiang and J. Yang, Palladium Catalyzed/Silver Tuned Selective mono-/tetra-Acetoxylation of o-Carboranes via B–H Activation, Chem. Commun., 2016, 52, 11446–11449 RSC.
- Y. Liu, X. Wang, X. Cai, G. Chen, J. Li, Y. Zhou and J. Wang, Highly Active Palladium-Based Catalyst System for the Aerobic Oxidative Direct Coupling of Benzene to Biphenyl, ChemCatChem, 2016, 8, 448–454 CrossRef CAS.
- A. M. Spokoyny, C. W. Machan, D. J. Clingerman, M. S. Rosen, M. J. Wiester, R. D. Kennedy, C. L. Stern, A. A. Sarjeant and C. A. Mirkin, A Coordination Chemistry Dichotomy for Icosahedral Carborane-Based Ligands, Nat. Chem., 2011, 3, 590–596 CrossRef CAS PubMed.
- R.-J. Tang, T. Milcent and B. Crousse, Regioselective Halogenation of Arenes and Heterocycles in Hexafluoroisopropanol, J. Org. Chem., 2018, 83, 930–938 CrossRef CAS PubMed.
- W. Wang, X. Yang, R. Dai, Z. Yan, J. Wei, X. Dou, X. Qiu, H. Zhang, C. Wang, Y. Liu, S. Song and N. Jiao, Catalytic Electrophilic Halogenation of Arenes with Electron-Withdrawing Substituents, J. Am. Chem. Soc., 2022, 144, 13415–13425 CrossRef CAS PubMed.
- Y. Wu, W. Lu, Y.-N. Ma, F. Chen, W. Ren and X. Chen, Trifluoromethanesulfonic Acid Promoted Controllable Electrophilic Aromatic Nitration, J. Org. Chem., 2023, 88, 11322–11327 CrossRef CAS PubMed.
- R.-J. Tang, P. Retailleau, T. Milcent and B. Crousse, Direct Amination of Arenes with Azodicarboxylates Catalyzed by Bisulfate Salt/HFIP Association, ACS Omega, 2019, 4, 8960–8966 CrossRef CAS PubMed.
- L. Zhu, Q.-B. Jiang and H. Yan, Synthesis and Characterization of Boron-substituted o-Carborane Derivatives Containing B−C, B−Cl or B−P Bond via B−H Activation, Chin. J. Inorg. Chem., 2014, 30, 2246–2252, DOI:10.11862/CJIC.2014.291.
- V. D. Vuković, E. Richmond, E. Wolf and J. Moran, Catalytic Friedel–Crafts Reactions of Highly Electronically Deactivated Benzylic Alcohols, Angew. Chem., Int. Ed., 2017, 56, 3085–3089 CrossRef PubMed.
- T. Hostier, V. Ferey, G. Ricci, D. G. Pardo and J. Cossy, Synthesis of Aryl Sulfides: Metal-Free C−H Sulfenylation of Electron Rich Arenes, Org. Lett., 2015, 17, 3898–3901 CrossRef CAS PubMed.
- R. H. Vekariya and J. Aubé, Hexafluoro-2-propanol-Promoted Intermolecular Friedel−Crafts Acylation Reaction, Org. Lett., 2016, 18, 3534–3537 CrossRef CAS PubMed.
- M. S. Kharasch and H. S. Isbell, The Chemistry of Organic Gold Compounds. III. Direct Introduction of Gold into the Aromatic Nucleus (Preliminary Communications), J. Am. Chem. Soc., 1931, 53, 3053–3059 CrossRef CAS.
- K. A. Kobe and T. F. Doumani, Aromatic Mercuration, Ind. Eng. Chem., 1941, 33, 170–176 CrossRef CAS.
- A. McKillop, J. D. Hunt, M. J. Zelesko, J. S. Fowler, E. C. Tay-lor, G. McGiIlivray and F. Kienzle, Thallium in Organic Synthesis. XXII. Electrophilic Aromatic Thallation Using Thallium(III) Trifluoroacetate. A Simple Synthesis of Aromatic Iodides, J. Am. Chem. Soc., 1971, 93, 4841–4844 CrossRef CAS.
- D. R. Harvey and R. O. C. Norman, Reactions of Lead Tetra-acetate. Part I. Reactions with Some Benzenoid Compounds, J. Chem. Soc., 1964, 4860–4868 RSC.
- V. I. Bregadze, V. T. Kampel and N. N. Godovikov, The Mercuration of ortho- and meta-Carboranes, J. Organomet. Chem., 1976, 112, 249–251 CrossRef CAS.
- V. I. Bregadze, A. Y. Usiatinsky and N. N. Godovikov, Thallation of Carboranes, J. Organomet. Chem., 1985, 292, 75–80 CrossRef CAS.
- R. G. Pearson, Absolute Electronegativity and Hardness: Application to Inorganic Chemistry, Inorg. Chem., 1988, 27, 734–740 CrossRef CAS.
- D. Xiang, J. A. Iñiguez, J. Deng, X. Guan, A. Martinez and X. Liu, AgII-Mediated Electrocatalytic Ambient CH4 Functionalization Inspired by HSAB Theory, Angew. Chem., Int. Ed., 2021, 60, 18152–18161 CrossRef CAS PubMed.
- H. Lyu, Y. Quan and Z. Xie, Transition Metal Catalyzed, Regioselective B(4)-Halogenation and B(4,5)-Diiodination of Cage B-H Bonds in o-Carboranes, Chem. – Eur. J., 2017, 23, 14866–14871 CrossRef CAS PubMed.
- P. Saravanan and P. Anbarasan, Palladium Catalyzed Aryl(alkyl)thiolation of Unactivated Arenes, Org. Lett., 2014, 16, 848–851 CrossRef CAS PubMed.
|
This journal is © the Partner Organisations 2025 |
Click here to see how this site uses Cookies. View our privacy policy here.