DOI:
10.1039/D4QO01312C
(Research Article)
Org. Chem. Front., 2025,
12, 85-89
Palladium-catalyzed hydrocarbonylative cross-coupling with two different alkenes†
Received
17th July 2024
, Accepted 20th October 2024
First published on 22nd October 2024
Abstract
Transition metal-catalyzed hydrocarbonylation of alkenes has been widely studied; however, the hydrocarbonylation reaction that took place between two alkenes has been largely unexplored. Herein, we report a palladium-catalyzed hydrocarbonylative cross-coupling reaction with two different alkenes to produce structurally diverse β-ketoaldehyde surrogates, which can be easily converted to different types of heterocycles including pyrazole, isoxazole and pyrimidine.
Transition metal-catalyzed hydrocarbonylation of alkenes is one of the most prominent methods for synthesizing carbonylated compounds. This process is widely used in the industrial production of fine chemicals and in the synthesis of natural products and bioactive molecules.1 Since Reppe's seminal work in the last century,2 transition metal-catalyzed hydrocarbonylation reactions using different types of nucleophiles to trap acylmetal species have been extensively studied, providing efficient and rapid synthesis of value-added amides, imides, carboxylic acids, esters, and thioesters.1,3 Despite the power and scope of these tremendous advances, most of the known methods focus on the hydrocarbonylation of a single alkene and the construction of C–O, C–N and C–S bonds. Only a few types of hydrocarbonylation reactions have been developed to form C–C bonds.4 Given the robustness of cross-coupling reactions with different alkenes in constructing C–C bonds and building molecular complexity,5 the hydrocarbonylative cross-coupling reaction with two different alkenes to form C–C bonds is thus of considerable interest. There are two studies involving this type of cross-coupling reaction,4b,c but both of them focused on the formation of linear carbonyl compounds and the use of aromatic alkenes as coupling partners.
Recently, we have developed a new and efficient strategy for the carbonylative assembly of two different alkenes, initiated by palladium-catalyzed hydrocarbonylative cycloadditions.6,7 These reactions provided novel strategies to furnish bridged carbonylated polycyclic molecules from two different alkenes in the presence of CO. Remarkable chemoselectivity was achieved by using the directing effect of the aldehyde or ketone functionality attached to one of the alkenes (Scheme 1A). Inspired by these results and in line with our continuing interest in carbonylation chemistry,1d,3i,j,8 we envisioned that the hydrocarbonylative cross-coupling reactions might also be realized between two alkenes with opposite electronic properties. Specifically, electron-deficient alkenes tend to be hydrocarbonylated to generate the corresponding acylmetal species under suitable conditions. Meanwhile, electron-rich alkenes, such as alkenyl ethers and alkenyl amines, could act as nucleophiles to capture the acylmetal species to furnish the carbonylative coupling products. Herein, we describe the development of a palladium-catalyzed chemoselective hydrocarbonylative cross-coupling reaction between electron-rich and electron-deficient alkenes in the presence of CO (Scheme 1B). Using this strategy, a wide range of β-ketoaldehyde surrogates were obtained, which are not only common structural motifs in bioactive compounds and natural products, such as tandyukisin, lymphostin and australifungin,9 but are also known as attractive building blocks in organic synthesis.10 Furthermore, a series of heterocycles were synthesized from the carbonylated products, which further demonstrated the applicability of this reaction.
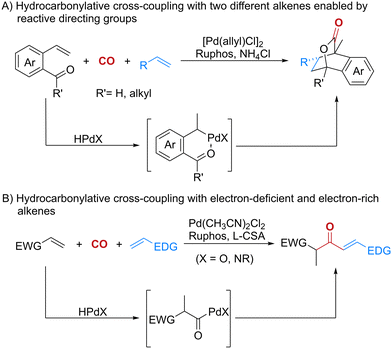 |
| Scheme 1 Hydrocarbonylative cross-coupling with two different alkenes. | |
We started our investigations with 3,4-dihydro-2H-pyran (1a) and acrylonitrile (2a) as substrates in anisole at 100 °C using Ruphos as a ligand to test different palladium catalyst precursors. To our delight, the desired product 3a could be easily obtained in 87% isolated yield when Pd(CH3CN)2Cl2 was utilized as the catalyst (Table 1, entry 1). PdBr2 and PdI2 showed lower catalytic activity, while other catalysts such as Pd(OAc)2 and Pd(acac)2 were ineffective for this carbonylation reaction (Table 1, entries 2–5). Subsequently, several ligands were evaluated (Table 1, entries 6–9), with Sphos and Davephos exhibiting comparable outcomes in this reaction, providing the desired products in 84% and 81% yields, respectively. Moreover, several common solvents such as THF, toluene, PhOMe and PhCl were also assessed (Table 1, entries 10–13); however, they afforded the products in lower yields.
Table 1 Optimization of the reaction conditionsa

|
Entry |
[Pd] |
Ligand |
Solvent |
Yield (%) |
Reaction conditions: 1 (0.5 mmol), 2 (0.6 mmol), catalyst (0.025 mmol, 5 mol%), ligand (0.06 mmol, 12 mol%), L-CSA (L-camphorsulfonic acid, 0.025 mmol, 5 mol%), CO (40 atm), solvent (1.0 mL), 100 °C, 12 hours, isolated yield.
Xantphos (0.03 mmol, 6 mol%).
|
1 |
Pd(CH3CN)2Cl2 |
Ruphos |
NMP |
87 |
2 |
PdBr2 |
Ruphos |
NMP |
61 |
3 |
PdI2 |
Ruphos |
NMP |
23 |
4 |
Pd(OAc)2 |
Ruphos |
NMP |
Trace |
5 |
Pd(acac)2 |
Ruphos |
NMP |
Trace |
6 |
Pd(CH3CN)2Cl2 |
Sphos |
NMP |
84 |
7 |
Pd(CH3CN)2Cl2 |
Davephos |
NMP |
81 |
8 |
Pd(CH3CN)2Cl2 |
n
BuPAd2 |
NMP |
63 |
9 |
Pd(CH3CN)2Cl2 |
Xantphosb |
NMP |
21 |
10 |
Pd(CH3CN)2Cl2 |
Ruphos |
THF |
15 |
11 |
Pd(CH3CN)2Cl2 |
Ruphos |
Toluene |
Trace |
12 |
Pd(CH3CN)2Cl2 |
Ruphos |
PhOMe |
25 |
13 |
Pd(CH3CN)2Cl2 |
Ruphos |
PhCl |
13 |
With the optimized reaction conditions established, the scope of this hydrocarbonylation process with respect to the electron-rich alkenes was first investigated (Scheme 2). As expected, 2,3-dihydrofuran was also an effective substrate for this reaction, affording the corresponding product (3b) in 66% yield. Notably, substrates containing acid-sensitive acetal functionality survived in this reaction, although product 3c was obtained in a lower yield. A variety of enol ethers bearing various substituents such as tetrahydro-2H-pyranyl, piperidyl, cyclohexyl and benzyl groups were all converted smoothly to the corresponding β-ketoaldehyde surrogates (3d–3g) in moderate to good yields. Moreover, enamides were also suitable substrates and reacted efficiently to deliver products 3h–3j. In addition to these medicinally important motifs, the chloro group, as well as ester and phthalimide functionalities, in products 3k–3m could provide a useful handle for further elaboration. It is worth noting that the reaction showed excellent chemoselectivity when enol ethers containing another alkenyl group were used as the substrates. The corresponding β-ketoaldehyde surrogates 3n–3p were smoothly formed, leaving another C
C bond behind. This good chemoselectivity may arise from the higher nucleophilicity of enol ethers. Furthermore, to demonstrate the potential of this hydrocarbonylation process in the diversification of natural products, a variety of enol ethers derived from citronellal, Lily aldehyde and cyclamen aldehyde were exploited, providing the desired products in moderate yields (3n and 3q–3r). We then turned our attention to vinyl ethers, and this electron-rich alkene afforded the desired mono-protected β-ketoaldehyde (3s) with a slightly reduced yield. Next, we investigated the generality of this hydrocarbonylation of alkenes. Unfortunately, only a few electron-deficient alkenes could be employed in this reaction (3t–3v).
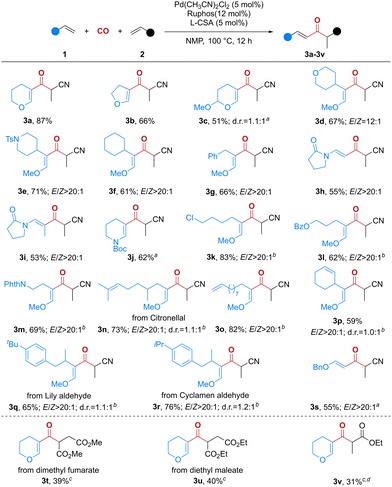 |
| Scheme 2 Substrate scopes. Reaction conditions: 1 (0.5 mmol), 2 (0.6 mmol), Pd(CH3CN)2Cl2 (0.025 mmol, 5 mol%), Ruphos (0.06 mmol, 12 mol%), L-CSA (0.025 mmol, 5 mol%), CO (40 atm), NMP (1.0 mL), 100 °C, 12 hours. Isolated yield. The values of E/Z and dr were determined by H NMR. a 2 (1.5 mmol), 90 °C, 24 h. b 2 (1.5 mmol), 110 °C. c PdI2 instead of Pd(CH3CN)2Cl2. d Davephos instead of Ruphos. | |
To demonstrate the synthetic potential of this reaction, we first conducted this carbonylation reaction on a gram scale, and the corresponding product 3g was obtained in 1.7 g with 74% yield (Scheme 3). In addition, a series of reactions were conducted to demonstrate the utility of our carbonylation reaction in achieving relevant heterocycles, which generally exhibit a diverse range of biological activities and play pivotal roles in medicinal chemistry.11 As shown in Scheme 3, the corresponding N-aryl-pyrazole and pyrazole derivatives (4 and 5) were readily obtained by treating 3g with hydrazine hydrate or phenylhydrazine in HOAc with 81% and 84% yields, respectively. In addition, 3,4-disubstituted isoxazole derivative 6 could also be obtained smoothly with 64% yield starting from 3g and hydroxylamine hydrochloride. Furthermore, 2,4,5-trisubstituted pyrimidine 7 was obtained in 63% yield from 3g, benzamidine hydrochloride and NaOMe at 80 °C.
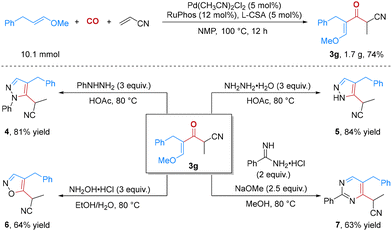 |
| Scheme 3 Gram-scale reaction and synthetic applications. | |
Based on the previous reports,4 a tentative mechanism of this hydrocarbonylation of two alkenes to construct β-ketoaldehyde surrogates is proposed (Fig. 1). Initially, the pre-catalyst Pd(II) is reduced to Pd(0) under the reaction conditions and then an oxidative addition of Pd(0) with L-camphorsulfonic acid (L-CSA) results in the formation of a cationic [H–PdL]+ complex and a camphorsulfonic anion (X−). The hydropalladium of acrylonitrile affords the alkylpalladium species I, which further reacts with CO to give acylpalladium II. The subsequent nucleophilic substitution of II produces intermediate III and regenerates the Pd(0) species for the next catalytic cycle. The desired product 3 is then generated via the deprotonation of intermediate III with the assistance of the anion.
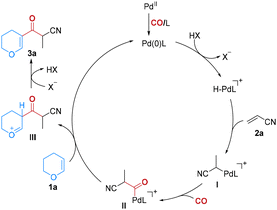 |
| Fig. 1 Proposed reaction mechanism. | |
Conclusions
In conclusion, we have developed a novel approach for palladium-catalyzed hydrocarbonylative cross-coupling between electron-deficient alkenes and enol ethers or enamides. The reaction provides a shortcut to access a series of β-ketoaldehyde surrogates from a diverse range of electron-rich alkenes. Different types of heterocycles including pyrazole, isoxazole and pyrimidine have been prepared originating from simple enol ether, CO and acrylonitrile, demonstrating the synthetic potential of this hydrocarbonylation reaction.
Author contributions
Y. Chen, J. Wu and Y. Ding conducted the experiments. Y. Ding wrote the initial manuscript draft. H. Huang conceptualized and directed the project and finalized the manuscript draft. All authors contributed to discussions.
Data availability
The data supporting this article have been included as part of the ESI.†
Conflicts of interest
The authors declare no conflicts of interest.
Acknowledgements
We are grateful for financial support from the National Key R&D Program of China (2023YFA1507500), the National Natural Science Foundation of China (21925111, 22350008 and 22301289), and the Strategic Priority Research Program of the Chinese Academy of Sciences (XDB0450301). This work was partially carried out at the Instruments Center for Physical Science, University of Science and Technology of China.
References
-
(a) R. Franke, D. Selent and A. Börner, Applied hydroformylation, Chem. Rev., 2012, 112, 5675–5732 CrossRef CAS PubMed;
(b) J. Li and Y. Shi, Progress on transition metal catalyzed asymmetric hydroesterification, hydrocarboxylation, and hydroamidation reactions of olefins, Chem. Soc. Rev., 2022, 51, 6757–6773 RSC;
(c) J.-B. Peng, X.-L. Liu, L. Li and X.-F. Wu, Palladium-catalyzed enantioselective carbonylation reactions, Sci. China: Chem., 2022, 65, 441–461 CrossRef CAS;
(d) S. Cai, H. Zhang and H. Huang, Transition-metal-catalyzed hydroaminocarbonylations of alkenes and alkynes, Trends Chem., 2021, 3, 218–230 CrossRef CAS;
(e) S. Zhang, H. Neumann and M. Beller, Synthesis of α, β-unsaturated carbonyl compounds by carbonylation reactions, Chem. Soc. Rev., 2020, 49, 3187–3210 RSC;
(f) W. Clegg, M. R. J. Elsegood, G. R. Eastham, R. P. Tooze, X. L. Wang and K. Whiston, Highly active and selective catalysts for the production of methyl propanoate via the methoxycarbonylation of ethene, Chem. Commun., 1999, 1877–1878 RSC;
(g) C. J. Rodriguez, D. F. Foster, G. R. Eastham and D. J. Cole-Hamilton, Highly selective formation of linear esters from terminal and internal alkenes catalysed by palladium complexes of bis-(di-tert-butylphosphinomethyl)benzene, Chem. Commun., 2004, 1720–1721 RSC.
- W. Reppe and H. Kröper, Carbonylierung II. Carbonsäuren und ihre derivate aus olefinischen verbindungen und kohlenoxyd, Justus Liebigs Ann. Chem., 1953, 582, 38–71 CrossRef CAS.
-
(a) P. Roesle, C. J. Dürr, H. M. Möller, L. Cavallo, L. Caporaso and S. Mecking, Mechanistic features of isomerizing alkoxycarbonylation of methyl oleate, J. Am. Chem. Soc., 2012, 134, 17696–17703 CrossRef CAS PubMed;
(b) H. Li, K. Dong, H. Jiao, H. Neumann, R. Jackstell and M. Beller, The scope and mechanism of palladium-catalysed Markovnikov alkoxycarbonylation of alkenes, Nat. Chem., 2016, 8, 1159–1166 CrossRef CAS PubMed;
(c) K. Dong, X. Fang, S. Gülak, R. Franke, A. Spannenberg, H. Neumann, R. Jackstell and M. Beller, Highly active and efficient catalysts for alkoxycarbonylation of alkenes, Nat. Commun., 2017, 8, 14117–14123 CrossRef PubMed;
(d) V. Hirschbeck, P. H. Gehrtz and I. Fleischer, Regioselective thiocarbonylation of vinyl arenes, J. Am. Chem. Soc., 2016, 138, 16794–16799 CrossRef CAS;
(e) X. Wang, B. Wang, X. Yin, W. Yu, Y. Liao, J. Ye, M. Wang, L. Hu and J. Liao, Palladium-catalyzed enantioselective thiocarbonylation of styrenes, Angew. Chem., Int. Ed., 2019, 58, 12264–12270 CrossRef CAS PubMed;
(f) H.-J. Ai, F. Zhao, H.-Q. Geng and X.-F. Wu, Palladium-catalyzed thiocarbonylation of alkenes toward linear thioesters, ACS Catal., 2021, 11, 3614–3619 CrossRef CAS;
(g) H. Liu, N. Yan and P. J. Dyson, Acid-free regioselective aminocarbonylation of alkenes, Chem. Commun., 2014, 50, 7848–7851 RSC;
(h) H. Li, K. Dong, H. Neumann and M. Beller, Palladium-catalyzed hydroamidocarbonylation of olefins to imides, Angew. Chem., Int. Ed., 2015, 54, 10239–10243 CrossRef CAS PubMed;
(i) G. Zhang, B. Gao and H. Huang, Palladium-catalyzed hydroaminocarbonylation of alkenes with amines: A strategy to overcome the basicity barrier imparted by aliphatic amines, Angew. Chem., Int. Ed., 2015, 54, 7657–7661 CrossRef CAS;
(j) B. Gao, G. Zhang, X. Zhou and H. Huang, Palladium-catalyzed regiodivergent hydroamino-carbonylation of alkenes to primary amides with ammonium chloride, Chem. Sci., 2018, 9, 380–386 RSC;
(k) J. Liu, H. Li, A. Spannenberg, R. Franke, R. Jackstell and M. Beller, Selective palladium-catalyzed aminocarbonylation of olefins to branched amides, Angew. Chem., Int. Ed., 2016, 55, 13544–13548 CrossRef CAS PubMed;
(l) T. Xu, F. Sha and H. Alper, Highly ligand-controlled regioselective Pd-catalyzed aminocarbonylation of styrenes with aminophenols, J. Am. Chem. Soc., 2016, 138, 6629–6635 CrossRef CAS PubMed;
(m) H.-Y. Yang, Y.-H. Yao, M. Chen, Z.-H. Ren and Z.-H. Guan, Palladium-catalyzed Markovnikov hydroaminocarbonylation of 1,1-disubstituted and 1,1,2-trisubstituted alkenes for formation of amides with quaternary carbon, J. Am. Chem. Soc., 2021, 143, 7298–7305 CrossRef CAS;
(n) Y.-H. Yao, H.-Y. Yang, M. Chen, F. Wu, X.-X. Xu and Z.-H. Guan, Asymmetric Markovnikov hydroaminocarbonylation of alkenes enabled by palladium-monodentate phosphoramidite catalysis, J. Am. Chem. Soc., 2021, 143, 85–91 CrossRef CAS PubMed;
(o) J.-B. Peng, H.-Q. Geng and X.-F. Wu, The chemistry of CO: Carbonylation, Chem, 2019, 5, 526–552 CrossRef CAS;
(p) J.-B. Peng, F.-P. Wu and X.-F. Wu, First-row transition-metal-catalyzed carbonylative transformations of carbon electrophiles, Chem. Rev., 2019, 119, 2090–2127 CrossRef CAS PubMed;
(q) J.-B. Peng and X.-F. Wu, Ligand- and solvent-controlled regio- and chemodivergent carbonylative reactions, Angew. Chem., Int. Ed., 2018, 57, 1152–1160 CrossRef CAS PubMed.
-
(a) J. Liu, Z. Wei, H. Jiao, R. Jackstell and M. Beller, Toward green acylation of (hetero)arenes: Palladium-catalyzed carbonylation of olefins to ketones, ACS Cent. Sci., 2018, 4, 30–38 CrossRef CAS;
(b) X.-F. Wu, H. Neumann and M. Beller, Palladium-catalyzed carbonylative dimerization of styrenes to 1,5-diarylpent-1-en-3-ones, Chem. – Asian J., 2012, 7, 1199–1202 CrossRef CAS PubMed;
(c) W. Chang, J. Dai, J. Li, Y. Shi, W. Ren and Y. Shi, A facile approach to ketones via Pd-catalyzed sequential carbonylation of olefins with formic acid, Org. Chem. Front., 2017, 4, 1074–1078 RSC.
-
(a) J. C. Lo, Y. Yabe and P. S. Baran, A practical and catalytic reductive olefin coupling, J. Am. Chem. Soc., 2014, 136, 1304–1307 CrossRef CAS PubMed;
(b) J. C. Lo, J. Gui, Y. Yabe, C.-M. Pan and P. S. Baran, Functionalized olefin cross-coupling to construct carbon-carbon bonds, Nature, 2014, 516, 343–348 CrossRef CAS PubMed;
(c) J. C. Lo, D. Kim, C.-M. Pan, J. T. Edwards, Y. Yabe, J. Gui, T. Qin, S. Gutiereez, J. Giacoboni, M. W. Smith, P. I. Holland and P. S. Baran, Fe-catalyzed C–C bond construction from olefins via radicals, J. Am. Chem. Soc., 2017, 139, 2484–2503 CrossRef CAS PubMed;
(d) Y. Tanaka, S. Kubosaki, K. Osaka, M. Yamawaki, T. Morita and Y. Yoshimi, Two types of cross-coupling reactions between electron-rich and electron-deficient alkenes assisted by nucleophilic addition using an organic photoredox catalyst, J. Org. Chem., 2018, 83, 13625–13635 CrossRef CAS PubMed.
- B. Gao, S. Zou, G. Yang, Y. Ding and H. Huang, Carbonylative cycloaddition between two different alkenes enabled by reactive directing groups: Expedited construction of bridged polycyclic skeletons, Chem. Commun., 2020, 56, 12198–12201 RSC.
- Y. Ding, M. Si and H. Huang, Palladium-catalyzed tandem hydrocarbonylative cycloaddition for expedient construction of bridged lactones, Org. Chem. Front., 2022, 9, 715–719 RSC.
-
(a) J. Li, S. Wang, S. Zou and H. Huang, Palladium-catalyzed relay hydroaminocarbonylation of alkenes with hydroxyl-amine hydrochloride as an ammonia equivalent, Commun. Chem., 2019, 2, 14 CrossRef;
(b) X. Ji, B. Gao, X. Zhou, Z. Liu and H. Huang, Palladium-catalyzed regioselective hydroaminocarbonylation of alkynes to α, β-unsaturated primary amides with ammonium chloride, J. Org. Chem., 2018, 83, 10134–10141 CrossRef CAS PubMed;
(c) Y. Ding and H. Huang, Carbonylative cycloaddition of alkenes and imines to β-lactams enabled by resolving the acid-base paradox, Chem. Catal., 2022, 2, 1467–1479 CrossRef CAS.
-
(a) A. Miyanaga, J. E. Janso, L. McDonald, M. He, H. Liu, L. Barbieri, A. S. Eustáquio, E. N. Fielding, G. T. Carter, P. R. Jensen, X. Feng, M. Leighton, F. E. Koehn and B. S. Moore, Discovery and assembly-line biosynthesis of the lymphostin pyrroloquinoline alkaloid family of mTOR inhibitors in Salinispora bacteria, J. Am. Chem. Soc., 2011, 133, 13311–13313 CrossRef CAS;
(b) O. D. Hensens, G. L. Helms, E. T. T. Jones and G. H. Harris, Structure elucidation of australifungin, a potent inhibitor of sphinganine N-acyltransferase in sphingolipid biosynthesis from Sporormiella australis, J. Org. Chem., 1995, 60, 1772–1776 CrossRef CAS;
(c) S. M. Mandala, R. A. Thornton, B. R. Frommer, J. E. Curotto, W. Rozdilsky, M. B. Kurtz, R. A. Giacobbe, G. F. Bills, M. A. Cabello, I. Martín and F. Peláez, The discovery of australifungin, a novel inhibitor of sphinganine N-acyltransferase from Sporormiella australis. Producing organism, fermentation, isolation, and biological activity, J. Antibiot., 1995, 48, 349–356 CrossRef CAS;
(d) T. Yamada, Y. Mizutani, Y. Umebayashi, N. Inno, M. Kawashima, T. Kikuchi and R. Tanaka, Tandyukisin, a novel ketoaldehyde decalin derivative, produced by a marine sponge-derived Trichoderma harzianum, Tetrahedron Lett., 2014, 55, 662–664 CrossRef CAS;
(e) J. Liu, F. Wu, H. Song, Z. Wang and L. Zhao, Synthesis and antibacterial activities of para-alkoxy phenyl-β-ketoaldehyde derivatives, Med. Chem. Res., 2013, 22, 4228–4238 CrossRef CAS.
-
(a) L.-H. Li and M. A. Tius, Stereospecific synthesis of cryptophycin 1, Org. Lett., 2002, 4, 1637–1640 CrossRef CAS PubMed;
(b) X.-J. Zhang, Z. Wang, H. Zhang, J.-J. Gao, K.-R. Yang, W.-Y. Fan, R.-X. Wu, M.-L. Feng, W. Zhu and Y.-P. Zhu, Iodine-mediated domino cyclization for one-pot synthesis of indolizine-fused chromones via metal-free sp3 C–H functionalization, J. Org. Chem., 2022, 87, 835–845 CrossRef CAS PubMed;
(c) S. Sundstrom, T. S. Nguyen and J. A. May, Relay catalysis to synthesize β-substituted enones: Organocatalytic substitution of vinylogous esters and amides with organoboronates, Org. Lett., 2020, 22, 1355–1359 CrossRef CAS PubMed;
(d) M. Li, Y. Liu and Y. J. Zhang, Route to chiral tetrahydrofuran acetals via Pd-catalyzed asymmetric allylic cycloaddition of vinyl epoxides with β-keto enol ethers, Org. Lett., 2022, 24, 6716–6721 CrossRef CAS.
- J. Shearer, J. L. Castro, A. D. G. Lawson, M. MacCoss and R. D. Taylor, Rings in clinical trials and drugs: Present and future, J. Med. Chem., 2022, 65, 8699–8712 CrossRef CAS.
|
This journal is © the Partner Organisations 2025 |
Click here to see how this site uses Cookies. View our privacy policy here.