DOI:
10.1039/D4QO01702A
(Research Article)
Org. Chem. Front., 2025,
12, 142-147
Metal-free visible-light-induced borylative/silylative pyridylation of vinylarenes†
Received
10th September 2024
, Accepted 20th October 2024
First published on 22nd October 2024
Abstract
We present a metal-free and mild three-component reaction involving vinylarenes, NHC–BH3 complexes, hydrosilanes, and 4-cyanopyridine. This versatile reaction encompasses a broad scope, with over 40 examples of vinylarenes, NHC–BH3 complexes, and hydrosilanes, leading to the synthesis of diverse β-pyridinyl boranes and β-pyridinyl silanes. Furthermore, we demonstrate the potential of this methodology by functionalizing pharmaceutical molecules, showcasing its practical applications. Remarkably, this reaction was performed with simple and inexpensive benzophenone, and notably, it did not require the presence of alkali or any costly transition metals.
Introduction
Organoboron compounds are effectively employed in a plethora of transformations pertinent to organic synthesis, catalysis, drug invention, materials science, and various other disciplines.1 As a result, a plethora of borylation reagents and synthetic techniques have been developed until now,2 encompassing hydroboration of alkenes and alkynes,3 the Miyaura borylation reaction,4 and C–H bond borylation,5 all of which have significantly propelled the progress of boron-centred chemistry. Despite these accomplishments, the endeavour to develop practical and widely applicable borylation reactions, which employ conceptually novel mechanisms to synthesize diverse functionalized organoboron compounds, continues to be a prominent aim in organic chemistry.
Recently, the field of boron-centred radical chemistry has gained significant momentum, with a significant proportion of these radicals stemming from N-heterocyclic carbene (NHC)–boranes, along with amine–boranes, which have emerged as key players in driving the advancement of this dynamic research area.6 The outstanding research conducted by Curran, Fensterbank, Lacôte, Malacria, and Wang7 demonstrates that N-heterocyclic carbene (NHC)–boryl radicals can be formed through the abstraction of hydrogen atoms from NHC–BH3 complexes, these being potent intermediates that facilitate the synthesis of a diverse array of high-value boron compounds.8 For instance, the seminal Giese-type addition of NHC–boryl radicals to unsaturated C–C bonds was independently pioneered by the groups of Curran and Wang,8,9 marking a significant milestone in the field. More recently, the Wang and Xie groups have independently disclosed a novel addition reaction involving NHC–boryl radicals generated through hydrogen atom transfer to activated alkenes.10,11 This advancement leverages the cooperative power of homogeneous photocatalysis and thiol catalysis, further expanding the boundaries of boron-centred radical chemistry. NHC–boryl radicals reacted with polyfluoroarenes, gem-difluoroalkenes, trifluoromethyl alkenes, and substituted 1,4-dicyanobenzenes have also been achieved by the Yang, Wu, Wang, and Curran groups.12
Despite the considerable advancements achieved in the addition of NHC–boryl radicals to multiple bonds,13 the utilization of these radicals in multicomponent reactions has thus far remained relatively scarce. In 2020, Wang and his team discovered a novel three-component reaction catalyzed by Ir(ppy)3, which involves the oxidation of NHC–BH3 to generate NHC–boryl radicals, followed by the addition of these NHC–boryl radicals to styrenes (Fig. 1a).12c In addition, the Quan group reported on the dialkylation of NHC–BH3 facilitated by a tetrabutylammonium decatungstate (TBADT)-catalyzed hydrogen atom transfer (HAT) process.14 Nevertheless, the use of transition metals iridium and tungsten in these two processes may introduce limitations regarding scalability and sustainability.
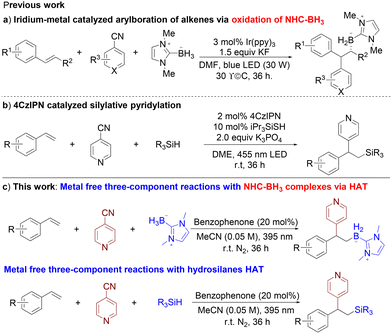 |
| Fig. 1 (a) Iridium metal-catalyzed arylboration of alkenes via oxidation of NHC–BH3. (b) 4CzIPN-catalyzed silylation pyridylation reaction. (c) Metal-free three-component reactions with NHC–BH3 complexes via HAT. | |
Consistent with our continuing dedication to developing a metal-free multicomponent reaction driven by hydrogen atom transfer (HAT) from diverse C–H precursors,15 we envision a novel approach where the boryl radical is generated through a HAT process with NHC–BH3 (Fig. 1c). This innovative strategy would enable the synthesis of diverse compounds through three-component reactions involving styrenes and cyano-pyridines, all without the need for any metallic catalysts. Such a development would further broaden the scope of environmentally friendly and cost-effective synthetic methodologies. Here, we showcase that only utilizing a benzophenone catalyst enables the efficient three-component borylative pyridylation of an extensive array of vinylarenes, leading to the synthesis of the targeted β-pyridinyl boranes in satisfactory yields under mild conditions. The key to the success of this reaction lies in the employment of an economical benzophenone catalyst, devoid of both alkaline metals and costly transition metals. Furthermore, this approach presents an innovative three-component pathway to β-pyridinyl silanes, which serve as crucial structural units in diverse biologically active molecules.
Results and discussion
We initiated our research by employing 20 mol% benzophenone as the catalyst, styrene as the alkene component, and NHC–BH3 as the source of boryl radicals, under irradiation with 395 nm light for 12 hours, resulting in the isolation of the desired product in 53% yield (Table S1,† entry 1). When the reaction time was prolonged to 36 hours, the desired product was isolated in 78% yield (Table S1,† entry 3), demonstrating a significant improvement in production efficiency. After an extensive screening of various catalysts (Table S1,† entries 6–9), benzophenone emerged as the most suitable choice for the reaction. To enhance product yield, an attempt was made to investigate the NHC–BH3 loading; however, this adjustment did not result in a corresponding increase in the yield of the desired product (Table S1,† entries 10–13). We further delved into the effect of varying both the concentration and the solvent (Table S1,† entries 14–19). Surprisingly, increasing the concentration led to a slight decrease in product yield. In addition, switching to alternative solvents also resulted in a decrease in the yield (DMSO) or totally suppressed the reaction (DMF and DCM), indicating that the initial conditions were optimal for maximizing the yield. When the reaction was conducted in the dark or in the absence of a catalyst, no product was observed. However, when the reaction was performed under air, the yield was significantly reduced to 46%. These results indicated that light irradiation, benzophenone, and the exclusion of oxygen were crucial to the success for this reaction (Table S1,† entries 20–22).
After identifying the optimal conditions for the reaction, we initially explored the versatility of vinylarenes as substrates in the multicomponent reaction. As shown in Scheme 1, the borylative pyridylation reaction occurred smoothly with a variety of substituted vinylarenes. Diverse styrenes proved to be efficient linchpins in the formation of borylative pyridylation products, facilitated by the reaction with NHC–BH3 and cyanopyridine. These reactions afforded borylative pyridylation products in good yields (ranging from 30% to 78%) with styrenes that possess electron-withdrawing and electron-donating functional groups. The electronic properties of styrenes had a pronounced impact on their reactivity, leading to notable variations. As an illustration, the target product was obtained in a relatively low yield of just 30% when using electron-rich styrenes, which is attributed to the destabilizing effect of the methoxy group on the benzyl radical (4h). In addition, the desired products were efficiently obtained in good yields through the successful transformation of α-methyl substituted styrene (4k and 4l). To our delight, 2-vinylthiophene as a vinylheterocycle has also been transformed to the corresponding product in 71% yield (4m). Moreover, we found that only the Giese addition product of the boryl radical was isolated with 2-vinylpyridine and 4-vinylpyridine as the substrates (4n and 4o). To demonstrate its potential pharmaceutical applications, we applied our boryl radical-involved multicomponent reaction in late-stage functionalization reactions. In particular, we treated substrates derived from ibuprofen, phytol, geraniol, oxaprozin, epiandrosterone, and estrone with NHC–BH3 under our reaction conditions and obtained the borylative pyridylation products in 31–81% yields (4p–4u).
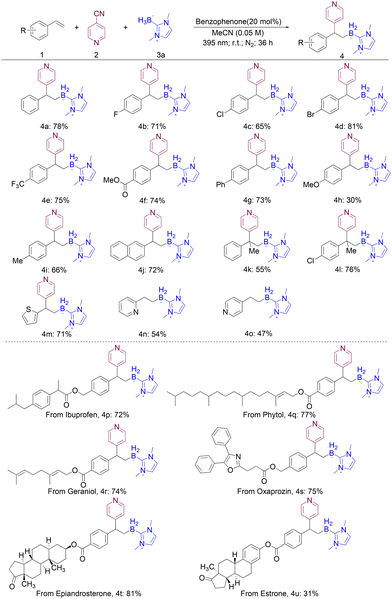 |
| Scheme 1 Scope of vinylarenes. Reaction conditions: compound 1 (0.4 mmol), compound 2 (0.2 mmol), compound 3a (0.6 mmol), and benzophenone (20 mol%) in MeCN (4 mL), room temperature, 395 nm blue LEDs, and 36 h, with an isolated yield. | |
We subsequently tested the substrate scope of NHC–BH3 in this multicomponent reaction and found that NHC–boranes were efficiently converted to the corresponding products in 66–72% yields (Scheme 2, 4v–4x).
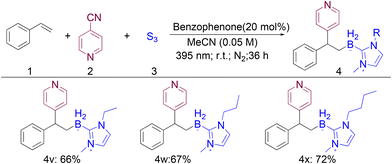 |
| Scheme 2 Scope of NHC–BH3 complexes. Reaction conditions: compound 1 (0.4 mmol), compound 2 (0.2 mmol), compound 3 (0.6 mmol), and benzophenone (20 mol%) in MeCN (4 mL), room temperature, 395 nm blue LEDs, and 36 h, with an isolated yield. | |
To enhance the synthetic versatility of this metal-free multicomponent reaction, we embarked on an exploration of reactions employing alternative heteroatom hydrogen (X–H) precursors. Organosilicon compounds are ubiquitous in numerous facets of contemporary chemistry, notably in chemical synthesis where organosilanes play a pivotal role in classic reactions, including the Fleming–Tamao, Hosomi–Sakurai, and Hiyama coupling reactions.16 In addition, the integration of silicon into pharmaceutical compounds has been shown to enhance their pharmacokinetic properties.17 Hence, the pursuit of novel methodological advancements aimed at the selective synthesis of intricate organosilicon molecules is of immense importance in organic synthesis.18
As depicted in Scheme 3, our excitement stemmed from the discovery that a wide array of hydrosilanes served as efficient substrates under the optimized conditions for borylative pyridylation reactions, affording silapyridylation products in yields ranging from moderate to outstanding. In stark contrast to prior investigations, this methodology transcends the limitation of solely utilizing sterically congested silane, as it demonstrates compatibility with a diverse range of sterically less hindered hydrosilanes as well. Trialkylsilanes, including those with varying alkyl group sizes such as triethylsilane 5a, tripropylsilane 5b, triisopropylsilane 5c, and tributylsilane 5d, all yielded the corresponding silapyridylation products in excellent yields. Furthermore, the silapyridylation reaction proceeded smoothly with triarylsilanes (5j–5l), yielding the corresponding products in good to excellent yields. Remarkably, this procedure exhibits the potential to be extended to primary and secondary silanes (5f and 5g), affording products that possess two Si–H bonds and one Si–H bond, respectively.
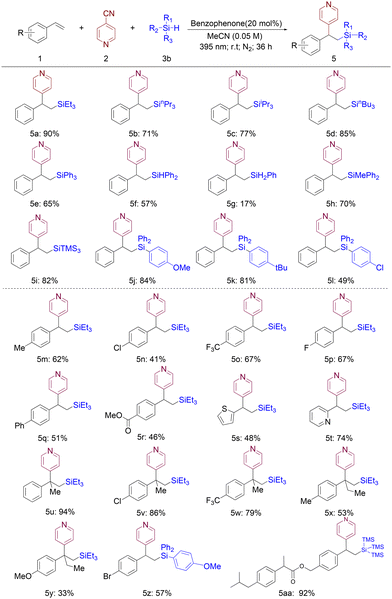 |
| Scheme 3 Substrate scope of hydrosilanes and styrenes. Reaction conditions: compound 1 (0.4 mmol), compound 2 (0.2 mmol), compound 3 (4 mmol), and benzophenone (20 mol%) in MeCN (4 mL), room temperature, 395 nm blue LEDs, and 36 h, with an isolated yield. | |
The scope of styrenes was explored subsequently. As shown in Scheme 3, styrenes with an electron-withdrawing and electron-donating substituent at the para-position, such as halogen (5n and 5p), trifluoromethyl (5o), ester (5r), methyl (5m), and methoxy (5y), delivered the expected products in good yields. Upon further investigation into this transformation with vinyl heterocycles, it was discovered that both 2-vinylpyridine (5t) and 2-vinylthiophene (5s) exhibited satisfactory performance, yielding the respective heterocycle β-pyridinyl silane products in 74% and 48% yields. Furthermore, 1,1-disubstituted styrenes can be effectively utilized to yield products (5x and 5y) featuring a quaternary carbon center in high yields. This mild and efficient reaction has been successfully employed in the late-stage functionalization of ibuprofen (5aa).
To demonstrate the potential application of our metal-free photoredox catalytic borylation methods, we successfully conducted a reaction by treating 4a with pinacol and N-chlorosuccinimide (NCS) in toluene at room temperature, resulting in the formation of β-pyridine boronate 11 in satisfactory yield (Fig. 2a). To gain insight into the underlying mechanism of this transformation, we designed and performed several meticulous control experiments. Among them, a radical clock experiment utilizing vinylcyclopropane as the olefin substrate was carried out, yielding the ring-open product 8 in 55% yield as a mixture of Z/E isomers in a 1
:
1 ratio (Fig. 2b).
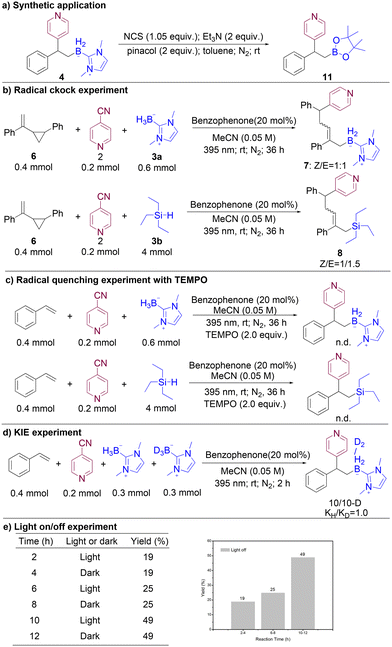 |
| Fig. 2 (a) Synthetic application; (b) radical quenching experiment with TEMPO; (c) radical clock experiment; (d) KIE experiment; and (e) light on/off experiment. | |
Notably, when 2,2,6,6-tetramethylpiperidine-1-oxyl (TEMPO) was introduced as a radical scavenger into the reaction mixture, the yield of the desired product was markedly diminished (Fig. 2c). The measurement of the kinetic isotope effect (kH/kD) for borane BH/BD was determined to be 1.0 (Fig. 2d), suggesting that the breaking of the B–H bond may not be the rate-determining step. In addition, we performed light on/off experiments, whose results pointed to the necessity of light irradiation for the reaction to proceed (Fig. 2e). Collectively, these findings strongly suggest that this protocol likely involves boron-centred radical intermediates as key players in the catalytic cycle (Fig. S7†).
Conclusions
In summary, we have disclosed a versatile strategy for the borylative and silylative pyridylation of vinylarenes, employing NHC–BH3 complexes and hydrosilanes. This approach enables the late-stage functionalization of complex, pharmaceutically significant molecules with good to excellent yields. Furthermore, it demonstrates broad tolerance for various functional groups, offering a unique method for accessing boron- and silicon-containing complex compounds. Our laboratory is currently engaged in intensified efforts to explore additional benzophenone-catalyzed multicomponent reactions.
Data availability
The data supporting this article have been included as part of the ESI.†
Conflicts of interest
There are no conflicts to declare.
Acknowledgements
This work is supported by the Jiangxi Provincial Natural Science Foundation (20224BAB203013) and the start-up Fund of Nanchang University (9167-28170030).
References
-
(a)
D. G. Hall, Boronic Acids: Preparation and Applications in Organic Synthesis Medicine and Materials, Wiley-VCH, Weinheim, Germany, 2nd edn, 2011 CrossRef;
(b) E. C. Neeve, S. J. Geier, I. A. I. Mkhalid, S. A. Westcott and T. B. Marder, Diboron(4) Compounds: From Structural Curiosity to Synthetic Workhorse, Chem. Rev., 2016, 116, 9091–9161 CrossRef CAS PubMed;
(c) A. B. Cuenca, R. Shishido, H. Ito and E. Fernández, Transition-Metal-Free B−B and B−Interelement Reactions with Organic Molecules, Chem. Soc. Rev., 2017, 46, 415–430 RSC;
(d) Z. Huang, S. Wang, R. D. Dewhurst, N. V. Ignat'ev, M. Finze and H. Braunschweig, Boron: Its Role in Energy-Related Processes and Applications, Angew. Chem., Int. Ed., 2020, 59, 8800–8816 CrossRef CAS.
-
(a) N. Miyaura and A. Suzuki, Palladium-Catalyzed Cross-Coupling Reactions of Organoboron Compounds, Chem. Rev., 1995, 95, 2457 CrossRef CAS;
(b) A. Suzuki, Cross-Coupling Reactions of Organoboranes: An Easy Way to Construct C–C Bonds (Nobel Lecture), Angew. Chem., Int. Ed., 2011, 50, 6722–6737 CrossRef CAS;
(c) I. A. I. Mkhalid, J. H. Barnard, T. B. Marder, J. M. Murphy and J. F. Hartwig, C–H Activation for the Construction of C–B Bonds, Chem. Rev., 2010, 110, 890–931 CrossRef CAS PubMed;
(d) D. Leonori and V. K. Aggarwal, Stereospecific Couplings of Secondary and Tertiary Boronic Esters, Angew. Chem., Int. Ed., 2015, 54, 1082–1096 CrossRef CAS;
(e) S. Namirembe and J. P. Morken, Reactions of Organoboron Compounds Enabled by Catalyst-Promoted Metalate Shifts, Chem. Soc. Rev., 2019, 48, 3464–3474 RSC;
(f) S. K. Bose, L. Mao, L. Kuehn, U. Radius, J. Nekvinda, W. L. Santos, S. A. Westcott, P. G. Steel and T. B. Marder, First-Row d-Block Element-Catalyzed Carbon−Boron Bond Formation and Related Processes, Chem. Rev., 2021, 121, 13238–13341 CrossRef CAS PubMed;
(g) J. Hu, M. Ferger, Z. Shi and T. B. Marder, Recent Advances in Asymmetric Borylation by Transition Metal Catalysis, Chem. Soc. Rev., 2021, 50, 13129–13188 RSC;
(h) Y.-M. Tian, X.-N. Guo, H. Braunschweig, U. Radius and T. B. Marder, Photo-Induced Borylation for the Synthesis of Organoboron Compounds, Chem. Rev., 2021, 121, 3561–3597 CrossRef CAS;
(i) Y. Li, D. Shen, H. Zhang and Z. Liu, Transition-Metal-Free Coupling Reactions Involving Gem-Diborylalkanes, Chin. Chem. Lett., 2023, 34, 108048 CrossRef CAS.
- S. J. Geier, C. M. Vogels, J. A. Melanson and S. A. Westcott, The Transition Metal-Catalysed Hydroboration Reaction, Chem. Soc. Rev., 2022, 51, 8877–8922 RSC.
-
(a) T. Ishiyama, M. Murata and N. Miyaura, Palladium(0)-Catalyzed Cross-Coupling Reaction of Alkoxydiboron with Haloarenes: A Direct Procedure for Arylboronic Esters, J. Org. Chem., 1995, 60, 7508–7510 CrossRef CAS;
(b) T. Ishiyama and N. Miyaura, Metal-Catalyzed Reactions of Diborons for Synthesis of Organoboron Compounds, Chem. Rec., 2004, 3, 271–280 CrossRef CAS;
(c) W. K. Chow, O. Y. Yuen, P. Y. Choy, C. M. So, C. P. Lau, W. T. Wong and F. Y. Kwong, A Decade Advancement of Transition Metal-Catalyzed Borylation of Aryl Halides and Sulfonates, RSC Adv., 2013, 3, 12518–12539 RSC;
(d) M. Wang and Z. Shi, Methodologies and Strategies for Selective Borylation of C–Het and C–C Bonds, Chem. Rev., 2020, 120, 7348–7398 CrossRef CAS PubMed.
-
(a) T. Ishiyama and N. Miyaura, Transition Metal-Catalyzed Borylation of Alkanes and Arenes via C–H Activation, J. Organomet. Chem., 2003, 680, 3–11 CrossRef CAS;
(b) J. F. Hartwig, Regioselectivity of the Borylation of Alkanes and Arenes, Chem. Soc. Rev., 2011, 40, 1992–2002 RSC;
(c) J. F. Hartwig, Borylation and Silylation of C–H Bonds: A Platform for Diverse C–H Bond Functionalizations, Acc. Chem. Res., 2012, 45, 864–873 CrossRef CAS PubMed;
(d) A. Ros, R. Fernández and J. M. Lassaletta, Functional Group Directed C–H Borylation, Chem. Soc. Rev., 2014, 43, 3229–3243 RSC;
(e) Y. Kuroda and Y. Nakao, Catalyst-Enabled Site-Selectivity in the Iridium-Catalyzed C–H Borylation of Arenes, Chem. Lett., 2019, 48, 1092–1100 CrossRef CAS;
(f) S. A. Iqbal, J. Pahl, K. Yuan and M. J. Ingleson, Intramolecular (Directed) Electrophilic C–H Borylation, Chem. Soc. Rev., 2020, 49, 4564–4591 RSC.
-
(a) J. H. Kim, T. Constantin, M. Simonetti, J. Llaveria, N. S. Sheikh and D. Leonori, A Radical Approach for the Selective C–H Borylation of Azines, Nature, 2021, 595, 677–683 CrossRef CAS;
(b) T. Taniguchi, Advances in Chemistry of N-Heterocyclic Carbene Boryl Radicals, Chem. Soc. Rev., 2021, 50(16), 8995–9021 RSC;
(c) L. Capaldo, T. Noël and D. Ravelli, Photocatalytic Generation of Ligated Boryl Radicals from Tertiary Amine-Borane Complexes: An Emerging Tool in Organic Synthesis, Chem. Catal., 2022, 2(5), 957–966 CrossRef CAS;
(d) T. Y. Peng, F. L. Zhang and Y. F. Wang, Lewis Base-Boryl Radicals Enabled Borylation Reactions and Selective Activation of Carbon-Heteroatom Bonds, Acc. Chem. Res., 2023, 56(2), 169–186 CrossRef CAS PubMed.
- S.-C. Ren, F.-L. Zhang, J. Qi, Y.-S. Huang, A.-Q. Xu, H.-Y. Yan and Y.-F. Wang, Radical Borylation/Cyclization Cascade of 1,6-Enynes for the Synthesis of Boron handled Hetero- and Carbocycles, J. Am. Chem. Soc., 2017, 139, 6050–6053 CrossRef CAS.
-
(a) S.-H. Ueng, M. M. Brahmi, E. Derat, L. Fensterbank, E. Lacôte, M. Malacria and D. P. Curran, Complexes of Borane and N-Heterocyclic Carbenes: A New Class of Radical Hydrogen Atom Donor, J. Am. Chem. Soc., 2008, 130, 10082–10083 CrossRef CAS PubMed;
(b) J. C. Walton, M. M. Brahmi, J. Monot, L. Fensterbank, M. Malacria, D. P. Curran and E. Lacôte, Electron Paramagnetic Resonance and Computational Studies of Radicals Derived from Boron-Substituted N-Heterocyclic Carbene Boranes, J. Am. Chem. Soc., 2011, 133, 10312–10321 CrossRef CAS;
(c) X. Pan, E. Lacôte, J. Lalevée and D. P. Curran, Polarity Reversal Catalysis in Radical Reductions of Halides by N-Heterocyclic Carbene Boranes, J. Am. Chem. Soc., 2012, 134, 5669–5674 CrossRef CAS;
(d) W. Dai, S. J. Geib and D. P. Curran, Facile Synthesis of α-N-Heterocyclic Carbene-Boryl Ketones from N-Heterocyclic Carbene-Boranes and Alkenyl Triflates, J. Am. Chem. Soc., 2019, 141, 12355–12361 CrossRef CAS PubMed.
- T. Watanabe, D. Hirose, D. P. Curran and T. Taniguchi, Borylative Radical Cyclizations of Benzo[3,4]cyclodec-3-ene-1,5-diynes and N-Heterocyclic Carbene-Boranes, Chem. – Eur. J., 2017, 23, 5404–5409 CrossRef CAS.
- S.-C. Ren, F.-L. Zhang, A.-Q. Xu, Y. Yang, M. Zheng, X. Zhou, Y. Fu and Y.-F. Wang, Regioselective Radical α-Borylation of α,β-Unsaturated Carbonyl Compounds for Direct Synthesis of α-Boryl Carbonyl Molecules, Nat. Commun., 2019, 10, 1934 CrossRef PubMed.
- C. Zhu, J. Dong, X. Liu, L. Gao, Y. Zhao, J. Xie, S. Li and C. Zhu, Photoredox-Controlled β-Regioselective Radical Hydroboration of Activated Alkenes with NHC-Boranes, Angew. Chem., Int. Ed., 2020, 59, 12817–12821 CrossRef CAS.
-
(a) P.-J. Xia, D. Song, Z.-P. Ye, Y.-Z. Hu, J.-A. Xiao, H.-Y. Xiang, X.-Q. Chen and H. Yang, Photoinduced
Single-Electron Transfer as an Enabling Principle in the Radical Borylation of Alkenes with NHC-Borane, Angew. Chem., Int. Ed., 2020, 59, 6706–6710 CrossRef CAS;
(b) W. Xu, H. Jiang, J. Leng, H.-W. Ong and J. Wu, Visible-Light-Induced Selective Defluoroborylation of Polyfluoroarenes, gem-Difluoroalkenes, and Trifluoromethylalkenes, Angew. Chem., Int. Ed., 2020, 59, 4009–4016 CrossRef CAS PubMed;
(c) J. Qi, F.-L. Zhang, J.-K. Jin, Q. Zhao, B. Li, L.-X. Liu and Y.-F. Wang, New Radical Borylation Pathways for Organoboron Synthesis Enabled by Photoredox Catalysis, Angew. Chem., Int. Ed., 2020, 59, 12876–12884 CrossRef CAS PubMed;
(d) W. Dai, S. J. Geib and D. P. Curran, 1,4-Hydroboration Reactions of Electron-Poor Aromatic Rings by N-Heterocyclic Carbene Boranes, J. Am. Chem. Soc., 2020, 142, 6261–6267 CrossRef PubMed.
- T. Taniguchi, Boryl Radical Addition to Multiple Bonds in Organic Synthesis, Eur. J. Org. Chem., 2019, 6308–6319 CrossRef CAS.
- F.-X. Li, X. Wang, J. Lin, X. Lou, J. Ouyang, G. Hu and Y. Quan, Selective Multifunctionalization of N-Heterocyclic Carbene Boranes via the Intermediacy of Boron-Centered Radicals, Chem. Sci., 2023, 14, 6341–6347 RSC.
- J. Xu and B. Liu, Metal Free Functionalization of Saturated Heterocycles with Vinylarenes and Pyridine Enabled by Photocatalytic Hydrogen Atom Transfer, Chem. – Eur. J., 2024, 30, e202400612 CrossRef CAS PubMed.
-
(a)
M. A. Brook, Silicon in Organic, Organometallic, and Polymer Chemistry, Wiley-Interscience, New York, 2000 Search PubMed;
(b) C. Cheng and J. F. Hartwig, Catalytic Silylation of Unactivated C–H Bonds, Chem. Rev., 2015, 115, 8946–8975 CrossRef CAS PubMed.
- A. K. Franz and S. O. Wilson, Organosilicon Molecules with Medicinal Applications, J. Med. Chem., 2013, 56, 388–405 CrossRef CAS PubMed.
-
(a) Y. Yang, R.-J. Song, X.-H. Ouyang, C.-Y. Wang, J.-H. Li and S. Luo, Iron-Catalyzed Intermolecular 1,2-Difunctionalization of Styrenes and Conjugated Alkenes with Silanes and Nucleophiles, Angew. Chem., Int. Ed., 2017, 56, 7916–7919 CrossRef CAS;
(b) J. Hou, A. Ee, H. Cao, H. W. Ong, J. H. Xu and J. Wu, Visible-Light-Mediated Metal-Free Difunctionalization of Alkenes with CO2 and Silanes or C(sp3)–H Alkanes, Angew. Chem., Int. Ed., 2018, 57, 17220–17224 CrossRef CAS PubMed;
(c) Z. Liu, J. Chen, H.-X. Lu, X. Li, Y. Gao, J. R. Coombs, M. J. Goldfogel and K. M. Engle, Palladium(0)-Catalyzed Directed syn-1,2-Carboboration and -Silylation: Alkene Scope, Applications in Dearomatization, and Stereocontrol by a Chiral Auxiliary, Angew. Chem., Int. Ed., 2019, 58, 17068–17073 CrossRef CAS;
(d) Z. Zhang and X. Hu, Arylsilylation of Electron-Deficient Alkenes via Cooperative Photoredox and Nickel Catalysis, ACS Catal., 2020, 10, 777–782 CrossRef CAS;
(e) M. Zheng, J. Hou, L. L. Hua, W. Tang, L. Zhan and B. Li, Visible-Light-Mediated Divergent Silylfunctionalization of Alkenes, Org. Lett., 2021, 23, 5128–5132 CrossRef CAS PubMed;
(f) S. Neogi, A. Kumar Ghosh, S. Mandal, D. Ghosh, S. Ghosh and A. Hajra, Three-Component Carbosilylation of Alkenes by Merging Iron and Visible-Light Photocatalysis, Org. Lett., 2021, 23, 6510–6514 CrossRef CAS PubMed;
(g) W. Zheng, Y. Xu, H. Luo, Y. Feng, J. Zhang and L. Lin, Light-Promoted Arylsilylation of Alkenes with Hydrosilanes, Org. Lett., 2022, 24, 7145–7150 CrossRef CAS PubMed;
(h) H. Xu, W. Zheng, W.-D. Liu, Y. Zhou, L. Lin and J. Zhao, Silylacylation of Alkenes through N-Heterocyclic Carbene Catalysis, Org. Lett., 2023, 25, 5579–5584 CrossRef CAS PubMed.
|
This journal is © the Partner Organisations 2025 |
Click here to see how this site uses Cookies. View our privacy policy here.