Eco-biocompatible periphyton-inhabited polyvinyl chloride (PVC) and polyacrylic acid (PAC) sheets indicate aquaculture bio-sustainability by oxidative stress and steatosis in zebrafish†
Received
10th November 2024
, Accepted 13th February 2025
First published on 14th February 2025
Abstract
Aquaculture practices increasingly rely on synthetic materials for tank construction, with poly vinyl chloride (PVC) and poly acrylic acid sheets (PAC) being prevalent due to their durability and cost-effectiveness. Moreover, periphytons play a crucial role in determining the efficiency of aquaculture. The eco-compatibility and impact on aquatic biota remain under-explored in the synthetic materials embedded with periphyton. This study investigates the effects of periphyton-inhabited PVC and PAC on the developmental and cellular physiological phenomena of embryonic zebrafish (Danio rerio). By exposing zebrafish embryos to aqueous environments containing periphyton-inhabited PVC and PAC sheets, we assessed the morphological development, survival rates, hatching rates, heart rates, and cellular stress responses. The presence of periphyton on these surfaces created microhabitats and was hypothesized to facilitate the recruitment and growth of desirable species, contributing to overall cellular and molecular biocompatibility. The cellular and molecular level assessment was done to gain mechanistic insights into the eco-biocompatibility of polymer sheets. Our findings indicate that exposure to periphyton inhabiting both materials can affect zebrafish embryogenesis, manifesting in developmental delays, increased mortality, and elevated cellular stress levels. Notably, PAC exhibited a higher degree of eco-compatibility compared to PVC, which showed more pronounced toxicological effects. The study detailed the ecotoxicological impact of PVC and PAC sheets with an indication of further research on eco-compatible design in aquaculture.
Sustainability spotlight
Aquaculture's role in global food security and rural economic development is expanding, with innovative practices driving its sustainability. A novel approach currently under study involves using synthetic materials like PVC and acrylic as substrata in aquaculture environments. These materials, commonly used in constructing aquaculture infrastructure, offer resilience, durability, and cost-effectiveness. When eco-compatible, such materials can enhance aquaculture efficiency by providing stable, reusable surfaces that support aquatic life while reducing environmental impact. This research explores the interaction of periphyton—a nutrient-rich biofilm that improves water quality—on polymer surfaces like PVC and acrylic to support aquaculture productivity. The periphyton on these surfaces could provide a natural food source and foster microhabitats beneficial to aquatic species, such as zebrafish. By assessing the developmental impact of periphyton-inhabited polymer sheets on zebrafish, this study offers insights into how these synthetic materials can support cellular and ecological health. Ultimately, findings from this research could lead to eco-friendlier aquaculture systems, where material choice balances enhanced fish production with the preservation of aquatic ecosystems. This research demonstrates how advancing aquaculture through eco-compatible materials aligns with multiple UN SDGs particularly SDG 2: Zero Hunger, SDG 8: Decent Work and Economic Growth, SDG 9: Industry, Innovation, and Infrastructure, SDG 12: Responsible Consumption and Production, SDG 14: Life Below Water, and SDG 15: Life on Land by promoting food security, economic growth, and ecosystem conservation. These innovations serve as a blueprint for developing sustainable aquaculture systems that respect both human and environmental needs.
|
1. Introduction
Aquaculture, the practice of cultivating aquatic organisms such as fish, crustaceans, mollusks and aquatic plants, plays a pivotal role in pisciculture, which focuses specifically on breeding and rearing fish. The sector has become a crucial component of the global food system, providing a significant source of protein and essential nutrients. The benefits of aquaculture extend beyond food production; it contributes to the economy by creating jobs, supporting livelihoods, and fostering economic development in rural areas. Additionally, aquaculture serves a critical function in the research and development (R&D) sector, particularly in the study of aquatic ecosystems, fish biology, and the improvement of breeding techniques.1 Aquatic water bodies, such as ponds, lakes, rivers, and coastal areas, are integral to these research endeavors. They provide natural environments where scientists can study the interactions between aquatic organisms and their habitats, enhancing our understanding of ecosystem productivity and sustainability.2 Efforts have been made to improve and expand the capacity and efficacy of aquatic bodies by using synthetic materials as their substratum. This study explores a novel strategy of using synthetic materials like plastic as a substratum to enhance the efficacy of aquaculture for sustainable production.
Plastics, widely used in various industries due to their versatility, durability, and cost-effectiveness, play a significant role in aquaculture. Different types of plastics, including poly vinyl chloride (PVC) and poly acrylic acid (PAC), are commonly used in constructing tanks, nets, and other aquaculture infrastructure. PVC, a synthetic plastic polymer, is known for its chemical resistance, durability, and ease of fabrication, making it ideal for water pipes, liners, and containment structures in aquaculture.3 Acrylic sheets, known for their clarity, light weight, and resistance to weathering, are used to create observation windows in tanks and aquariums, facilitating better monitoring of aquatic organisms.4 It can be hypothesized that using these plastic sheets within an eco-compatible range can be helpful in enhancing the aquaculture capacity.
Periphyton, a complex mixture of algae, cyanobacteria, heterotrophic microbes, and detritus that attach to submerged surfaces in aquatic environments, plays a significant role in aquaculture.5 These biosystems serve as a primary food source for many aquatic organisms and contribute to the overall productivity of aquatic ecosystems. Periphyton enhances water quality by absorbing nutrients and pollutants, thus preventing harmful algal blooms and promoting a healthier environment for fish and other aquatic species.6 In aquaculture systems, the presence of periphyton can enhance the growth and survival rates of cultured species by providing a natural and nutritious food source. Additionally, periphyton helps in stabilizing sediments and reducing erosion, thereby maintaining the structural integrity of aquatic habitats. Owing to the properties of periphytons and plastics, it can be hypothesized that embedding periphytons on the surface of plastic layers in aquatic bodies can be a smart strategy for higher aquaculture production, given the condition of efficacy of biocompatibility.
This study evaluates the eco-compatibility of periphyton-inhabited polymer sheets, specifically poly vinyl chloride (PVC) and poly acrylic acid sheets (PAC), to assess their impact on the developmental and cellular physiological phenomena of aquatic biota using embryonic zebrafish as a model organism. The fish model has been recognized as one of the popular models to determine the toxicological impact of different compounds used in daily activities owing to their utility in food consumption.7 Many fish models like fathead minnow, rainbow fish, and guppies have been recommended as model organisms in the acute toxicity assay.8 In recent years, zebrafish, scientifically known as Danio rerio, have been widely used in scientific research due to their transparent embryos, rapid development, and genetic similarity to humans.9 It has been recognized as one of the emerging in vivo models in different biomedical and environmental research. Numerous literature reports have mentioned their utility in the determination of the toxicological impact of different compounds,10 emerging contaminants,11,12 and nanoparticles.13,14 The model has been fruitful in drug discovery, as disease models in biomedical research.9
The presence of periphyton on these polymer surfaces creates microhabitats that are hypothesized to facilitate the recruitment and growth of desirable species.15 This could contribute to overall cellular and molecular biocompatibility. In this study, we evaluated the developmental and morphological phenomena of zebrafish. We conducted assessments at the cellular and molecular levels to uncover mechanistic insights into the eco-biocompatibility of these polymer sheets. By investigating the interaction between periphyton, polymer surfaces, and zebrafish development, this research aims to provide a comprehensive understanding of how these materials impact aquatic environments.15 The findings could have significant implications for the design and selection of materials used in aquaculture infrastructure, ensuring that they support sustainable and eco-friendly practices. The study's outcomes are expected to contribute to the development of more sustainable aquaculture systems that not only enhance the productivity and health of cultured species but also protect and preserve aquatic ecosystems. By focusing on the eco-compatibility of commonly used polymers, this research addresses a critical need in the aquaculture industry for materials that balance functionality with environmental stewardship.16
2. Materials and methods
2.1. Chemicals
Poly vinyl chloride (PVC) with an average Mw ∼ 233
000 and average Mn ∼ 99
000, poly acrylic acid (PAC) with an average Mw ∼ 450
000 and 2′,7′-dichlorodihydrofluorescin diacetate (H2DCFDA, purity ≥ 99%) were procured from Sigma-Aldrich. Acridine Orange (AO) and BODIPY stain were supplied by Thermo Scientific. All chemicals and solvents used were of analytical grade. Commercial PAC and PVC sheets (5 × 5 cm2) were obtained from certified vendors.
2.2. Zebrafish and embryo maintenance
All animal procedures adhered to the guidelines of the Institutional Animal Ethics Committee (IAEC) of KIIT University. The experiments were conducted in accordance with the guidelines set by the OECD. Adult zebrafish were kept in a system with an overflow container supplied by Aquaneering, USA. Fish water, prepared with 18 g sea salt, 75 g NaHCO3, and 8.4 g CaSO4 per 1000 mL, was used to equilibrate the system. For breeding, males and females were separated by a divider and kept in a breeding tank at a ratio of 1
:
2, respectively, under a photoperiod of 14/10 hours of dark and light.16 The divider was removed at dawn to allow breeding. The eggs obtained were collected and thoroughly washed with embryo medium.17
2.3.
In vivo toxicity analysis
In vivo toxicological evaluation of periphyton-inhabited polyacrylic acid (PAC) and polyvinyl chloride (PVC) was performed using zebrafish (Danio rerio) embryos as a model organism. Commercially sourced PAC (1 g m−2V/A) and PVC sheets (1 g m−2V/A) were utilized for the study. Embryos were exposed to periphyton-inhabited PAC and PVC samples in 500 μL of egg water within a well plate, containing 20 embryos per well. The exposure commenced at 24 hours post-fertilization (hpf) and continued until 72 hpf. Experimental conditions were maintained at 28 ± 1 °C with a photoperiod of 14/10 hours of dark and light. Untreated embryos served as a control group to facilitate comparisons of morphological abnormalities and mortality rates against treated groups. Morphological and developmental anomalies were observed using microscopy, and their frequencies were documented relative to the control group. The embryo survival rate was determined by the ratio of live embryos to the total number of embryos at 24, 48, and 72 hours post-exposure. The hatching rate was calculated as the proportion of hatched embryos to the total number of embryos after 72 hours. The heart rate was measured in beats per minute. All experimental runs were performed in triplicate and repeated on three different occasions. This research was endorsed by the Institutional Animal Ethics Committee (IAEC) of KIIT University, complying with all IAEC protocols.
2.4. Cellular ROS analysis
The reactive oxygen species quantification of PAC and PVC effects in zebrafish (Danio rerio) embryos was performed by assessing the mean fluorescence analysis of the exposed medium using flow cytometry.18 Cellular suspensions were prepared from both control (unexposed) and treated (exposed) embryos via sonication for 10 minutes at 10 second intervals. To evaluate the cytotoxic effects of PAC and PVC sheets, oxidative stress induction in the zebrafish embryos was analyzed by measuring reactive oxygen species (ROS) levels. This was achieved using both fluorescence microscopy and flow cytometry, employing the ROS indicator H2DCFDA. For fluorescence microscopy, embryos were exposed to PAC and PVC for 72 hours and then rinsed with sterilized egg water. Subsequently, they were stained with 20 μg per mL H2DCFDA and incubated in the dark for 20 minutes. After incubation, the excess stain was removed by washing the embryos with egg water. The oxidative stress induced by PAC and PVC exposure was visualized by capturing images with an EVOS-inverted fluorescence microscope (Thermo Scientific, USA). For flow cytometry analysis, cellular suspensions from both control and exposed embryos were stained with H2DCFDA to facilitate ROS detection. This allowed for a comprehensive assessment of the cytotoxic impact of PAC and PVC on zebrafish embryos. The samples were subsequently analyzed using an Attune Acoustic Focusing Cytometer (Applied Biosystems, Life Technologies), which is equipped with a 488 nm argon laser. Data analysis and visualization were conducted using FCS Express 7.19
2.5. Apoptosis and steatosis analysis
The mechanistic evaluation of PVC and PAC toxicity was conducted by assessing apoptosis and steatosis induction in zebrafish embryos. Apoptosis was identified using acridine orange,18 while steatosis was detected using BODIPY dye,20 with both assessments performed via fluorescence microscopy and flow cytometry. For fluorescence microscopy, unexposed and exposed embryos were stained with 10 μg per mL acridine orange for apoptosis detection and 5 μg per mL BODIPY for steatosis analysis. After a 20 minute incubation period in the dark, excess stain was washed off, and images were captured using an EVOS inverted fluorescence microscope (Thermo Scientific, USA) with a green filter.21 For flow cytometry, cellular suspensions from both unexposed and exposed embryos were similarly stained with acridine orange and BODIPY. These samples were then analyzed with an Attune Acoustic Focusing Cytometer (Applied Biosystems, Life Technologies) equipped with a 488 nm argon laser. Data analysis and visualization were performed using FCS Express 7.
2.6.
In silico analysis
To uncover the mechanism of the molecular state of the embryonic zebrafish protein, the hatching enzyme (Zhe1) and the two polymer, PAC and PVC, methods were employed. For the protein (Zhe1) and ligand (PAC and PVC) interaction analysis, molecular docking was conducted individually. Before molecular docking, the protein and ligand files were prepared. The 3D structures of PAC and PVC were generated from CHARMM. Further, the geometry and energy optimization was done using the UFF forcefield Avogadro. PMV was used for energy minimization in receptor protein Zhe1. AutoDock 4.2.6/AutoDock Tools 1.5.6 was used to perform molecular docking of the Zhe1 with PAC and PVC. The parameters for PAC and PVC were set in Autodock 4.2.6. The grid dimensions for Zhe1 were set to 40 × 54 × 44, having a spacing of 1 Å. The docking was performed for the ligand–receptor complex (PAC–Zhe1 and PVC–Zhe1). Subsequently, post-docking analysis was performed by the identification of optimal binding sites, characterized by the lowest binding energy and 0 rmsd value. Post-docking analysis was done with the help of conformational clustering and visualized using PyMol, Discovery Studio Visualizer, and ligplot+.
2.7. Statistical analysis
Statistical analysis was conducted using GraphPad Prism version 8.0.1 (San Diego, California). Data were analyzed using one-way ANOVA followed by Tukey's post hoc test, with significance set at P < 0.05. Results were assessed for each concentration individually. Additionally, a non-parametric Spearman correlation analysis was performed to evaluate the relationship between ROS and apoptosis data.
3. Results and discussion
3.1.
In vivo biocompatibility
The aquatic biotoxicity of periphyton-inhabited PVC and PAC was assessed by analyzing their cellular and molecular effects on in vivo biocompatibility of zebrafish embryos. As shown in Fig. 1A, the survival rate of the embryos was found to be unaffected by exposure duration and exposure material. The findings suggested the negligible effect of both the periphyton-inhabited PAC and PVC plastic sheets on the embryos. Further, as shown in Fig. 1B the hatching rate was assessed to understand the developmental effects on embryos due to contact with plastic sheets. The results showed a significant increase in the hatching rate in embryos exposed to PVC and PAC sheets compared to the control. The result can be attributed to the interaction of surface proteins of the chorion with PAC and PVC on the chorion surface during exposure. Fig. 1C reveals the heart rate of the embryos exposed to periphyton-inhabited PAC and PVC sheets. The heart rate was found to be decreased in both cases; however, the decline was higher in the case of PVC sheet exposure. This decline is likely due to cellular changes at the molecular level, such as induced oxidative stress and its effects on the circulatory system.22
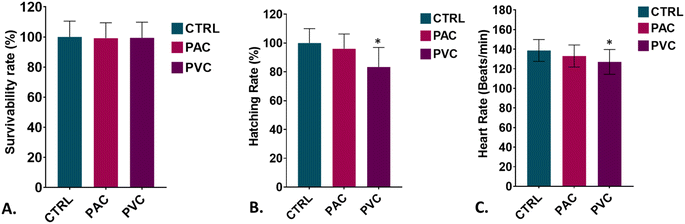 |
| Fig. 1 Biotoxicity examination of PAC and PVC in embryonic zebrafish in vivo. (A) The survival rate of zebrafish embryos was evaluated upon exposure to polyvinyl chloride (PVC) and acrylic (PAC) sheets. (B) The hatching rate was assessed at 72 hours post-exposure for embryos treated with certain concentrations of PVC and PAC. (C) Heart rate was determined for zebrafish embryos following 72 hours of exposure to these materials. All experimental analyses were conducted in triplicate and repeated three times independently. Data are presented as mean ± SD, based on observations from 20 embryos per replicate. Statistical significance was determined using post hoc analysis following a one-way ANOVA, with significance thresholds set at *P > 0.5 and **P > 0.01, indicating notable changes to exposure concentration. | |
The changes in physiological parameters observed in embryos exposed to PAC and PVC suggested the induction of overall morphological abnormalities. Fig. 2 illustrates the morphology of embryos exposed for 24, 48, and 72 hours to periphyton-inhabited PAC and PVC sheets. Morphological abnormalities, such as pericardial edema and abnormal notochord development, were observed in relation to exposure duration. Embryos appeared healthy after 24 hours of exposure. However, after 48 hours, there was a noticeable increase in the frequency of pericardial edema. By 72 hours, distinct abnormalities in the notochord were apparent, with a higher frequency of these abnormalities corresponding to increased exposure duration of periphyton-inhabited PAC and PVC sheets. These findings are consistent with literature reports on the effects of other chemicals and xenobiotic compounds.23 The observed morphological abnormalities can be attributed to the cellular and molecular effects that occurred due to durational exposure to periphyton-inhabited PAC and PVC sheets, which likely interfere with developmental processes.18
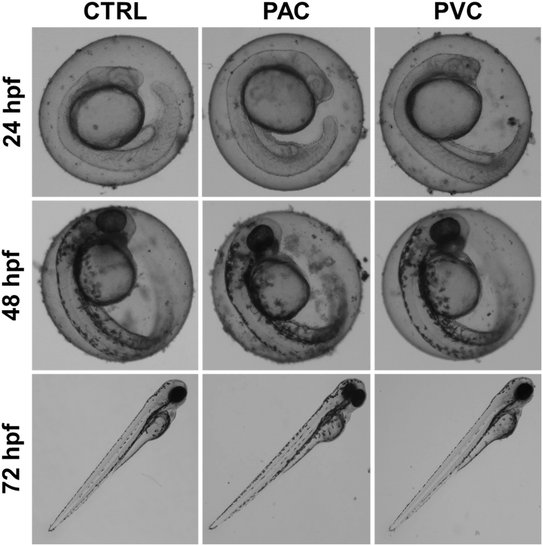 |
| Fig. 2 Morphological changes induced in zebrafish embryos exposed to PAC & PVC sheets at different exposure times. | |
The experimental results, coupled with the observed abnormal hatching rates, suggest that PAC and PVC interact with surface proteins of the chorion, e.g. hatching proteins Zhe1a.24 To understand this in detail, computational analysis was done to comprehend the interaction of PAC and PVC with Zhe1 at the molecular level. PAC and PVC were found to interact with Zhe1 through different amino acids.25 The computational analysis illustrated the Zhe1 protein and PAC interaction through various amino acids, including His106, Tyr155, Leu136, Gln138, and Ala74, demonstrating an average binding affinity of −5.9 kcal mol−1 (Fig. 3 and Table 1). Additionally, the interaction of the Zhe1 protein with PVC involved amino acids such as Lys187, Gly150, Gln181, and Gln178, exhibiting an average binding affinity of −1.2 kcal mol−1 (Fig. 4 and Table 1). The residues participated in hydrophobic and hydrogen bonds in the case of PAC, whereas only hydrophobic interactions were observed in the case of PVC. Post-docking results showed that the binding affinity of PVC was −1.2 kcal mol−1, and that of PAC was −5.9 kcal mol−1 (Table 2). This indicates that PAC has more binding affinity towards Zhe1, and PVC has negligible to significantly less binding affinity with Zhe1.The in silico investigation results predicted an interaction of proteins with PAC and PVC at the molecular level; however, the experimental results showed a higher intensity of changes. The phenomenon can be related to a wholesome phenotypic expression of the results to the complex metabolic system in the zebrafish embryos taken as an in vivo model for investigation.
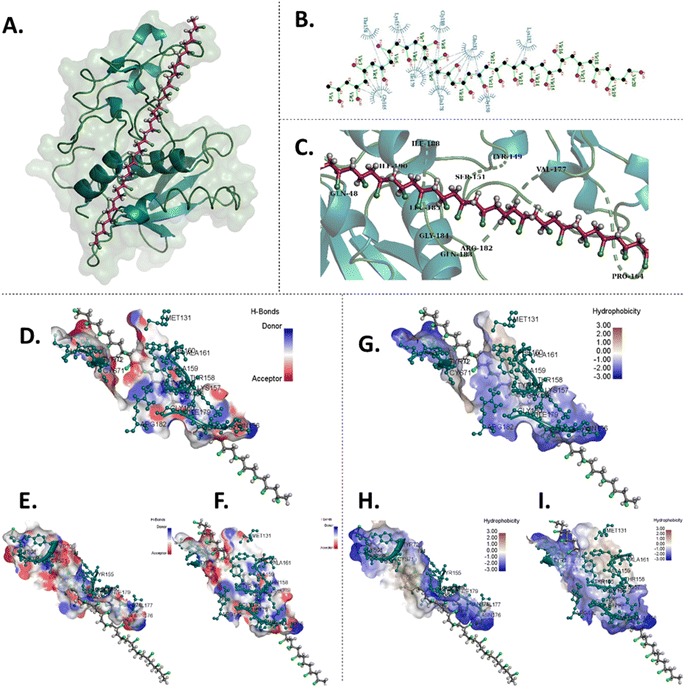 |
| Fig. 3
In silico molecular docking analysis of the interaction between PVC and Zhe1 enzyme displaying amino acids involved in the interaction and the Zhe1 surface characteristic. (A) 3D representation of docked PVC with Zhe1. (B) 2D representation of PVC with interacting amino acids. (C) 3D and zoomed-in image showcasing amino acids of Zhe1 participating in interaction with PVC. (D) Zhe1-H-bond surface display, (E) left side of the Zhe1 H-bond surface display and (F) right side of the Zhe1 H-bond surface display. (G) Zhe1 hydrophobic surface display, (H) left side of the Zhe1 hydrophobic surface display and (I) right side of the Zhe1 hydrophobic surface display. | |
Table 1 Post-molecular docking binding energies of PAC and PVC with various zebrafish receptor proteins, displaying the interacting residues
Sl. no. |
Ligand |
Protein |
Binding affinity (kcal mol−1) |
Residues participating in hydrophobic interactions |
Residues forming hydrogen bonds (bond length in Å) |
1 |
PAC |
Zhe1 |
−5.9 |
Ala159, Gly156, Phe160, Ala161, Ser128, His109, Ile96, Arg182, Tyr155, Leu136, Gln138, Ala74, Thr78, Val83, Gly70, His99 |
Residues |
Bond length in Å |
Tyr158 |
3.32 |
Gly180 |
3.26 |
Cys71 |
3.24 |
Tyr72 |
3.22 |
Ser73 |
3.29 |
Tyr133 |
3.02 |
Asn134 |
3.21 |
2 |
PVC |
Zhe1 |
−1.2 |
Lys187, Gly150, Gln181, Gln178, Gly180, Ile179, Lys157, Gly165, Thr158 |
|
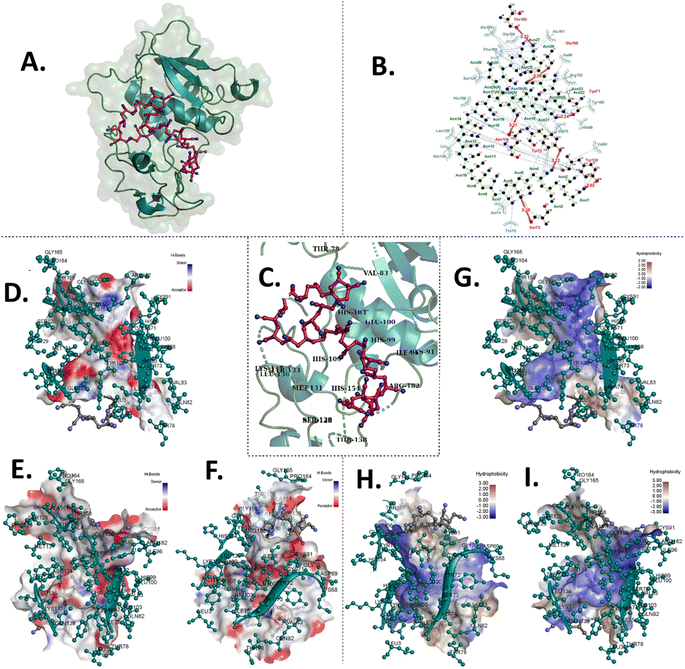 |
| Fig. 4
In silico molecular docking analysis illustrating the interaction between PAC and the Zhe1 enzyme, highlighting the involved amino acids and the Zhe1 surface features. (A) 3D depiction of PAC docked with Zhe1. (B) 2D diagram showing PAC and Zhe1 interacting amino acids. (C) 3D zoomed-in view of Zhe1 amino acids interacting with PAC. (D) Comprehensive Zhe1 hydrogen bond surface display. (E) Left view of the Zhe1 hydrogen bond surface display. (F) Right view of the Zhe1 hydrogen bond surface display. (G) Comprehensive Zhe1 hydrophobic surface display. (H) Right view of the Zhe1 hydrophobic surface display. (I) Left view of the Zhe1 hydrophobicity surface display. | |
Table 2 Binding affinity (kcal mol−1) of different conformational modes of PAC and PVC with Zhe1a protein of zebrafish
Modes |
Binding affinity (kcal mol−1) |
PAC |
PVC |
1 |
−5.9 |
−1.2 |
2 |
−5.9 |
−0.9 |
3 |
−5.9 |
−0.9 |
4 |
−5.7 |
−0.9 |
5 |
−5.6 |
−0.9 |
6 |
−5.6 |
−0.8 |
7 |
−5.6 |
−0.8 |
8 |
−5.6 |
−0.8 |
9 |
−5.6 |
−0.8 |
10 |
−5.4 |
−0.8 |
11 |
−5.4 |
−0.8 |
12 |
−5.4 |
−0.8 |
13 |
−5.4 |
−0.8 |
14 |
−5.4 |
−0.7 |
15 |
−5.3 |
−0.7 |
3.2. Cellular toxicity of PAC and PVC
The experimental results elucidated the in vivo impact of periphyton-inhabited PAC and PVC sheet exposure on zebrafish embryos, highlighting the induction of physiological and morphological abnormalities due to the induced metabolic changes. It was hypothesized that the interaction of the chorion surface with the sheet may induce hypoxic conditions inside the embryos due to periphyton blocking the pores which can further lead to metabolic disturbances like oxidative stress and apoptosis in embryos.23 Previous studies have reported the in vivo biotoxicity of xenobiotic compounds, concluding that hypoxic conditions can induce oxidative stress and apoptosis-like phenomena. Additionally, the literature has suggested that xenobiotic compounds can disrupt lipid molecule transport and transformation, playing a crucial role in the development of steatosis. Given these findings, PAC and PVC were hypothesized to exert distinct effects, necessitating a comprehensive experimental and computational investigation to uncover the cellular mechanisms underlying periphyton-inhabited PAC and PVC biocompatibility.26
To assess oxidative stress induced by periphyton-inhabited PAC and PVC sheet exposure in embryonic cells, H2DCFDA staining was employed as a biomarker. H2DCFDA produces green fluorescence upon reaction with reactive oxygen species (ROS). The green fluorescence in zebrafish embryos exposed to PAC and PVC was evaluated using fluorescence microscopy and flow cytometry. As shown in Fig. 5A and B, fluorescence microscopy revealed a differentiated green fluorescence of H2DCFDA with exposure to PAC and PVC sheets, with higher intensity in the case of PAC compared to PVC sheets. These findings were corroborated by flow cytometry results from cellular suspensions of PAC and PVC-exposed zebrafish embryos. As illustrated in Fig. 5C, a significant rightward shift in mean fluorescence intensity of H2DCFDA was observed in cellular suspensions of zebrafish embryos exposed to PAC, indicating increased ROS levels with PAC exposure. However, upon PVC exposure, the shift trended leftward. The increase in ROS is likely due to increased ROS production by cells compensating for reduced oxygen availability caused by chorion pore blockage and chorion hardening. However, the subsequent decrease in ROS due to PVC exposure may be attributed to the ROS-scavenging properties of PVC. Oxidative stress is known to play a pivotal role in cell death processes. Toxicologists have demonstrated that xenobiotic compounds exhibit toxicity in zebrafish by inducing abnormal apoptosis through high oxidative stress and reactive oxygen species (ROS) generation caused by molecular irregularities. It was hypothesized that both PVC and PAC induce irregular apoptosis due to ROS dysregulation. This hypothesis was tested through experimental evaluations. As shown in Fig. 6A and B, the green fluorescence of acridine orange, used as a marker for apoptosis analysis, was found to be differentiated with PVC and PAC exposure compared to the unexposed embryos. These microscopy results were corroborated by flow cytometry, which showed similar variations in acridine orange fluorescence in embryos exposed to PAC and PVC, as depicted in Fig. 6C. The results suggest differential upregulation and downregulation of apoptosis induction. This may be attributed to dysregulation in the structural and functional activities of apoptosis-related metabolic proteins due to intrinsic atomic interactions with internalized PAC and PVC.
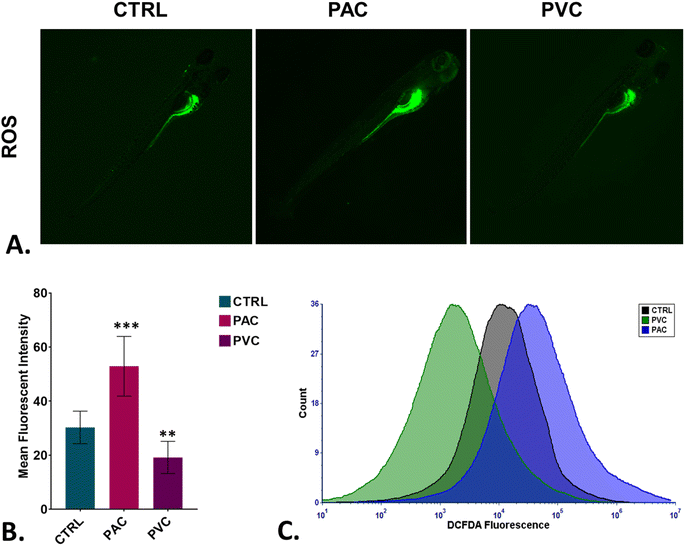 |
| Fig. 5 Cellular toxicity assessment of PAC and PVC using embryonic zebrafish. (A) Detection of green DCFDA fluorescence indicating ROS production in zebrafish embryos treated with certain concentrations of PAC and PVC. (B) Mean fluorescence intensity of DCFDA in zebrafish embryos exposed to different levels of PAC and PVC quantified using fluorescence microscopy; mean ± SD values derived from three independent trials. Statistical significance with *P > 0.5, **P > 0.01, and *P > 0.001 reflects differences from control concentrations, determined by post hoc analysis following one-way ANOVA. The experimental procedures were replicated three times independently, with each analysis conducted in triplicate. (C) A graph illustrating green DCFDA fluorescence indicates ROS generation in zebrafish embryo cells treated with certain PAC and PVC concentrations, which were analyzed by flow cytometry. | |
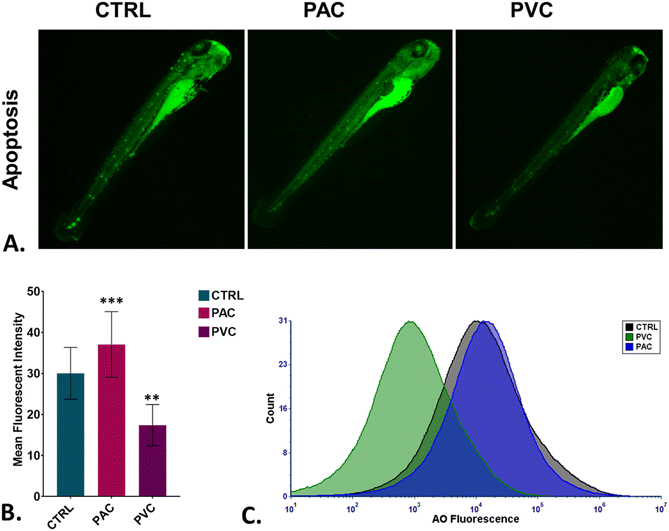 |
| Fig. 6
In vivo toxicity assessment of PAC and PVC using embryonic zebrafish. (A) Detection of green AO fluorescence indicating apoptosis production in zebrafish embryos treated with periphyton-inhabited PAC and PVC sheets. (B) Mean AO fluorescence intensity in zebrafish embryos exposed to different PAC and PVC sheets quantified using fluorescence microscopy; mean ± SD values derived from three independent trials. Statistical significance with *P > 0.5, **P > 0.01, and *P > 0.001 indicates significant differences relative to control levels, identified through post hoc analysis following one-way ANOVA. (C) The graph shows green AO fluorescence signalling apoptosis induction in zebrafish embryo cells treated with periphyton-inhabited PAC and PVC and assessed via flow cytometry. | |
An imbalance in ROS production has been demonstrated to play an important role in cellular metabolic functions such as lipid metabolism. Alteration in lipid metabolism, known as “steatosis,” has been reported in embryonic zebrafish exposed to various xenobiotics.23 Different types of lipid molecules and lipoproteins are involved in the physiological processes of zebrafish embryos. Apolipoproteins, such as apoa1a, are synthesized by the syncytial layer of the yolk during embryonic development. These apolipoproteins facilitate the formation of cytoplasmic lipid droplets and very-low-density lipoprotein (VLDL) from the embryo's yolk lipids. The formed VLDL and low-density lipoprotein (LDL) are subsequently delivered to different tissues via the circulatory system. Experimental verification was conducted using flow cytometry and fluorescence microscopy to analyse the fluorescence intensity of BODIPY in zebrafish embryos exposed to PAC and PVC. BODIPY is known to stain neutral lipid droplets, such as LDL and VLDL, in cells. As shown in Fig. 7A and B, the mean fluorescence intensity of green fluorescence from BODIPY increased in zebrafish embryos with PAC and PVC, as observed through fluorescence microscopy images. Flow cytometry data corroborated the microscopic analysis results shown in Fig. 7C. These findings indicate an increase in neutral lipid concentration in zebrafish embryo cells exposed to PAC and a decrease in those exposed to PVC. This effect can be attributed to the influence of PAC and PVC on the structural and functional activity of the apoa1a protein, leading to increased LDL and VLDL transport to the circulatory system and subsequent tissue transference. Additionally, this phenomenon can be correlated with abnormalities in other metabolic processes, such as ROS induction and apoptosis. Based on the obtained data, it can be inferred that the uptake and accumulation of PAC and PVC in zebrafish embryos, due to their relative exposure, influence the structural and functional activity of Zhe1 proteins, leading to abnormalities in metabolic phenomena such as oxidative stress, apoptosis, and steatosis. Previous reports have shown the effects of PAC and PVC on oxidative stress, apoptosis, and steatosis in cells of different origins. Therefore, based on the experimental results and previous reports, it can be concluded that PAC and PVC exhibit cytotoxicity by altering cellular metabolic processes at an intrinsic level.
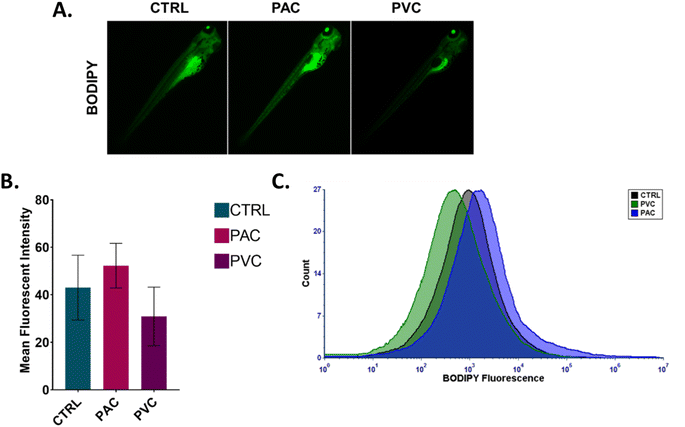 |
| Fig. 7
In vivo toxicity of PAC and PVC with embryonic zebrafish mediated by steatosis. (A) Green fluorescence of BODIPY dye indicating induced steatosis in zebrafish embryos treated with a certain concentration of PAC and PVC. (B) Mean fluorescence intensity of BODIPY in zebrafish embryos exposed to a certain concentration of PAC and PVC estimated by fluorescence microscopy. The values represent the mean ± SD of three independent experiments. *P > 0.5, **P > 0.01, and ***P > 0.001 denote the compared significant change at each exposed concentration obtained from post hoc analysis after one-way ANOVA. All the experimental analyses were done independently in triplicate and thrice. (C) Histogram presenting green fluorescence of BODIPY to indicate ROS induction in zebrafish embryo cells exposed to certain concentrations of PAC and PVC determined by flow cytometry. | |
4. Mechanism
The mechanism underlying the biotoxicity of PVC and PAC can be outlined as follows: when exposed to zebrafish embryos, PVC and PAC come into contact with the surface of the chorion. This interaction leads to interference with the chorion pores and interactions with the chorion hardening and hatching protein Zhe1a. As a result, the normal hardening of the chorion and the hatching rate become irregular. These irregularities in hardening and pore blockage create hypoxic conditions within the embryo sac, which trigger an increased induction of reactive oxygen species (ROS). Furthermore, PAC and PVC molecules interact with the Zhe1 protein on a fundamental level, causing structural and functional abnormalities that result in abnormal ROS production. Additionally, PAC and PVC interact with other metabolic proteins, disrupting the transfer of LDL and VLDL to their target tissues, which leads to disruptions in neutral lipid metabolism. The irregular induction of ROS further leads to abnormal programmed cell death (apoptosis). Moreover, PAC and PVC intrinsically interact with other apoptotic proteins like Zhe1, contributing to dysfunctional processes that also lead to abnormal apoptosis. The cumulative impact of these metabolic disturbances disrupts the normal cellular apoptosis process, resulting in cytotoxic effects due to concentration-based exposure of both PAC and PVC to zebrafish embryos. Therefore, the study elucidated and clarified the molecular mechanism of PAC and PVC biotoxicity. This information emphasizes the need for controlled and cautious use of PAC and PVC-based materials to safeguard environmental and aquatic health sustainably.
5. Conclusion
The study conducted on the biotoxicity of periphyton-inhabited PAC and PVC sheets in aquaculture, using the zebrafish embryonic model, has provided critical insights into the biological effects of these materials on aquatic organisms. Our findings reveal that exposure to periphyton-inhabited PAC and PVC leads to significant cellular and molecular abnormalities, which manifest as physiological and developmental disturbances in zebrafish embryos. The primary mechanism of toxicity was identified as the accumulation of PAC and PVC on the chorion surface, resulting in hypoxic conditions within the embryo sac. Additionally, the internalization of these materials and their intrinsic interaction with the Zhe1 protein disrupted its functionality, leading to oxidative stress, apoptosis, and steatosis. These results underscore the potential risks associated with the widespread use of PAC and PVC in aquaculture and other aquatic environments. The induced oxidative stress and cellular damage observed in the study suggest that the biotoxicity of these materials can have far-reaching implications for aquatic life and ecosystem health. Therefore, it is imperative to implement controlled and judicious use of PAC and PVC to mitigate their adverse effects. This study advocates for the development of safer alternatives and stricter regulatory measures to ensure sustainable and environmentally responsible aquaculture practices.
Data availability
The data supporting this article have been included in the ESI.†
Author contributions
Dr Suresh K. Verma and Dr Ch.Vinod designed the research, supervised the experiments, analyzed the data, and edited the manuscript. All the experiments were implemented by Mitali Sahoo, Sudakshya S. Lenka, Snehasmita Jena, Aishee Ghosh, and Adrija Sinha. In silico analysis was done by Sudakshya S. Lenka. The manuscript was compiled by Mitali Sahoo, Sudakshya S. Lenka, Snehasmita Jena, and Adrija Sinha.
Conflicts of interest
The authors declare that they have no known competing financial interests or personal relationships that could have appeared to influence the work reported in this paper.
Acknowledgements
We acknowledge the infrastructure support available through the DBT-BUILDER program (BT/INF/22/SP42155/2021) at KIIT University.
References
- G. S. Araujo, J. W. A. da Silva, J. Cotas and L. Pereira, J. Mar. Sci. Eng., 2022, 10, 1598 CrossRef.
- A. T. Mansour, M. Ashour, A. E. Alprol and A. S. Alsaqufi, Sustainability, 2022, 14, 3257 CrossRef CAS.
- K. Lewandowski and K. Skórczewska, Polymers., 2022, 14, 3035 CrossRef CAS PubMed.
-
A. Akelah, Funct. Polym. Mater. Agric. Food Ind., 2013, pp. 65–131 Search PubMed.
- M. Meerhoff and M. d. l. Á. González-Sagrario, Hydrobiologia, 2022, 849, 3737–3760 Search PubMed.
- H. Milhazes-Cunha and A. Otero, Algal Res., 2017, 24, 416–424 CrossRef.
-
O. Guideline, F. O. R. Testing and O. F. Chemicals, Test No. 212: Fish, Short-term Toxicity Test on Embryo and Sac-Fry Stages, OECD, 1998, DOI:10.1787/9789264070141-en.
- D. Zhu, T. T. Li, S. S. Zheng, L. C. Yan, Y. Wang, L. Y. Fan, C. Li and Y. H. Zhao, Chemosphere, 2018, 213, 414–422 CrossRef CAS PubMed.
- S. K. Verma, A. Nandi, A. Sinha, P. Patel, S. Mohanty, E. Jha, S. Jena, P. Kumari, A. Ghosh, I. Jerman, R. S. Chouhan, A. Dutt, S. K. Samal, Y. K. Mishra, R. S. Varma, P. K. Panda, N. K. Kaushik, D. Singh and M. Suar, Biomed. Pharmacother., 2024, 171, 116160, DOI:10.1016/j.biopha.2024.116160.
- S. K. Verma, A. Nandi, A. Sinha, P. Patel, E. Jha, S. Mohanty, P. K. Panda, R. Ahuja, Y. K. Mishra and M. Suar, Precis. Nanomed., 2021, 4, 750–782 Search PubMed.
- U. Saha, A. Ghosh, A. Sinha, A. Nandi, S. S. Lenka, A. Gupta, S. Kumari, A. Yadav, M. Suar, N. K. Kaushik, V. Raina and S. K. Verma, Mater. Today Bio, 2025, 31, 101466, DOI:10.1016/j.mtbio.2025.101466.
- A. Sinha, S. S. Lenka, A. Gupta, D. Singh, A. Choudhury, S. S. Naser, A. Ghosh, F. Z. Simnani, A. Nandi, R. Mishra, S. K. Verma and M. Suar, Environ. Sci.:Nano, 2025, 12, 1592–1608, 10.1039/D4EN00558A.
- B. Sarkar, S. K. Verma, J. Akhtar, S. P. Netam, S. K. Gupta, P. K. Panda and K. Mukherjee, Chemosphere, 2018, 206, 560–567 CrossRef CAS PubMed.
- S. SinghDeo, S. S. Naser, A. Nandi, A. Sinha, S. A. Shaikh, S. K. Mohapatra, M. Suar, S. K. Verma and J. Tripathy, Colloids Surf., B, 2025, 245, 114212, DOI:10.1016/j.colsurfb.2024.114212.
- T. Y. Choi, T. I. Choi, Y. R. Lee, S. K. Choe and C. H. Kim, Exp. Mol. Med., 2021, 53, 310 CrossRef CAS PubMed.
- M. Troell, B. Costa-Pierce, S. Stead, R. S. Cottrell, C. Brugere, A. K. Farmery, D. C. Little, Å. Strand, R. Pullin, D. Soto, M. Beveridge, K. Salie, J. Dresdner, P. Moraes-Valenti, J. Blanchard, P. James, R. Yossa, E. Allison, C. Devaney and U. Barg, J. World Aquacult. Soc., 2023, 54, 251–342 CrossRef.
- S. K. Verma, A. Nandi, A. Sinha, P. Patel, S. Mohanty, E. Jha, S. Jena, P. Kumari, A. Ghosh, I. Jerman, R. S. Chouhan, A. Dutt, S. K. Samal, Y. K. Mishra, R. S. Varma, P. K. Panda, N. K. Kaushik, D. Singh and M. Suar, Biomed. Pharmacother., 2024, 171, 116160 CrossRef CAS PubMed.
- P. Patel, P. K. Panda, P. Kumari, P. K. Singh, A. Nandi, M. A. Mallick, B. Das, M. Suar and S. K. Verma, Ecotoxicol. Environ. Saf., 2020, 192, 110321, DOI:10.1016/j.ecoenv.2020.110321.
- P. K. Panda, P. Kumari, P. Patel, S. K. Samal, S. Mishra, M. M. Tambuwala, A. Dutt, K. Hilscherová, Y. K. Mishra, R. S. Varma, M. Suar, R. Ahuja and S. K. Verma, Green Chem., 2022, 24, 1190–1210 RSC.
- A. Kamkaew, S. H. Lim, H. B. Lee, L. V. Kiew, L. Y. Chung and K. Burgess, Chem. Soc. Rev., 2013, 42, 77–88 RSC.
- S. K. Verma, E. Jha, P. K. Panda, A. Thirumurugan, S. Patro, S. K. S. Parashar and M. Suar, Mater. Sci. Eng. C, 2018, 92, 807–818 CrossRef CAS PubMed.
- K. Singh, S. K. Verma, P. Patel, P. K. Panda, A. Sinha, B. Das, V. Raina, M. Suar and L. Ray, Environ. Res., 2022, 212(D), 113496, DOI:10.1016/j.envres.2022.113496.
- A. Choudhury, S. S. Lenka, A. Gupta, D. Mandal, A. Sinha, U. Saha, S. S. Naser, D. Singh, F. Z. Simnani, A. Ghosh, S. Kumari, A. Kirti, T. Parija, R. S. Chauhan, N. K. Kaushik, M. Suar and S. K. Verma, Sci. Total Environ., 2024, 949, 175243, DOI:10.1016/j.scitotenv.2024.175243.
- S. K. Verma, A. Nandi, A. Sinha, P. Patel, E. Jha, S. Mohanty, P. K. Panda, R. Ahuja, Y. K. Mishra and M. Suar, Precis. Nanomed., 2021, 4(1), 750–781, DOI:10.33218/001c.21978.
- N. M. Mahmoodi, F. Najafi and A. Neshat, Ind. Crops Prod., 2013, 42, 119–125 CrossRef CAS.
- A. Nakajima, H. Hamada and S. Hayashi, Precision Nanomedicine, 1966, 95, 40–51 CAS.
|
This journal is © The Royal Society of Chemistry 2025 |
Click here to see how this site uses Cookies. View our privacy policy here.