A multi-interaction conductive double-network polyelectrolyte hydrogel with high stretchability, self-adhesion, and tunable transparency for bioelectronic sensing and information encryption†
Received
30th July 2024
, Accepted 12th November 2024
First published on 19th November 2024
Abstract
Polyelectrolyte hydrogels, combining the conductivity of polyelectrolytes with the flexibility of hydrogels, have become a popular candidate for flexible sensors, soft robotics, and electronic skins due to their remarkable stability in electrical conductivity. However, their mechanical properties and adhesive strength are limited because of their single-type bonding interaction. This work introduces a double-networked (DN) polyelectrolyte hydrogel formed through polymer chain entanglements, chemical crosslinking, and the incorporation of multiple strong and/or weak bonding interactions. The first network is a chemically crosslinked polyacrylamide (PAAm). The second network consists of polyelectrolytes (poly(diallyldimethylammonium chloride) (PDDA) and poly(methacrylic acid sodium salt) (PMAANa)), which form numerous weak and/or strong ionic bonds. Weak and/or strong hydrogen bonds are present within and between two networks. The mechanical properties and adhesive strength of the polyelectrolyte DN hydrogel can be tailored through modulating the content of PAAm, polyelectrolytes, and co-solvents. The optimal compositions have a tensile modulus of 10.8 kPa, tensile fracture strain of 1000%, and adhesive strength of 37.8 kPa. The hydrogel sensors are successfully applied to flexible electrodes for various devices for detecting human motion, handwriting recognition, and continuous monitoring of electrophysiological signals. The distinctive solvent-adjustable transparency of the gel also allows for its utilization in information encryption and decryption.
1 Introduction
Flexible conductive materials have garnered significant interest due to their broad applications in wearable electronic devices, especially in health monitoring,1 sensing devices,2,3 electronic skin,4,5 and soft robotics.6,7 The preparation strategies involve the integration of soft materials including polymer films, elastomers, or hydrogels with conductive components such as metal nanoparticles,8 liquid metals,9 carbon-based nanomaterials (graphene oxide,10 carbon nanotubes11 and carbon dots12,13), MXenes,14,15 conjugated conductive polymers,16,17 and ionic compounds.18,19 Electrically conductive hydrogels that utilize electrically conductive materials20,21 as fillers often encounter several issues. These include incomplete conductive pathways at low filler concentrations and insufficient conductivity due to aggregation at high concentrations, as well as high costs, complex synthesis, and opacity, which hinder their applications in wearable electronics.22–24 Ionic hydrogels offer superior ionic conductivity,25,26 a broad strain detection range,27 excellent biocompatibility,28 and unique transparency,29 thus presenting notable advantages in wearable flexible electronics. Common methods for preparing ionic hydrogels include adding soluble salts to precursor solutions30,31 or immersing the hydrogel in external salt solutions,32,33 both of which are prone to ion leakage. It compromises conductive stability and increases ecotoxicity to organisms.34
In contrast, low-cost polyelectrolyte hydrogels integrate the intrinsic conductivity of polyelectrolytes with the flexibility of hydrogels, offering advantages35 such as strong and stable conductivity, high biocompatibility, adjustable mechanical properties, and dynamic cross-linking. They can transmit electrical signals through suppressed counterions within the hydrogel matrix without leakage and are unaffected by external salts,36 highlighting their excellent conductive stability.37 Electrostatic interactions between oppositely charged groups in polyelectrolytes form reversible physical ionic bonds within the hydrogel network,38,39 enhancing the self-healing and self-adhesion properties of polyelectrolyte hydrogels.40–42 However, conventional single-network polyelectrolyte gels, lacking covalent bonds for toughening mechanisms, still demonstrate limited mechanical strength, presenting challenges for their adaptation in complex environments and hindering their practical applications.43 Immersing these hydrogels in salt solutions leads to rapid degradation because salt ions disrupt electrostatic interactions by binding with positively and negatively charged groups.44 The supramolecular double networked (DN) hydrogels (PVA–PAANaPAH) was developed by combining a PVA network and a polyelectrolyte network comprised of polyacrylic acid sodium (PAANa) and polyallylamine (PAH). It exhibited exceptional mechanical and conductive properties. However, the gel preparation, involving freeze–thaw or borax crosslinking, consumes a significant number of hydroxyl groups in the PVA chain, resulting in insufficient self-adhesiveness (4.6 kPa) of the hydrogel.37 Additional adhesive aids, such as tape, are necessary to secure the hydrogel in the desired position on the human body when used in wearable sensors. Interface separation between the hydrogel and the skin, caused by low adhesive strength during usage, compromises the accuracy and reproducibility of sensing signals.45 Therefore, it is still imperative to develop polyelectrolyte hydrogels with strong adhesion, mechanical strength, and high stability.
In this work, we aim to develop a DN polyelectrolyte hydrogel, integrating the PAAm network and the polyelectrolyte network composed of poly (methacrylic acid sodium) (PMAANa) and poly (diallyldimethylammonium chloride) (PDDA) (Scheme 1A), by incorporating multiple strong and/or physical interactions, polymer chain entanglement, and chemical crosslinking. This hydrogel was synthesized via one-step random polymerization of AAm to PAAm in cosolvent (H2O and isopropanol (IPA)), forming the first network, and the second network was spontaneously formed between PDDA and PMAANa. The hydrogel is denoted as xPAAm–yPMa–yPDA–zIPA, where x, y and z represent the content of AAm, polyelectrolytes, and IPA, respectively. This DN polyelectrolyte hydrogel involves inner-network hydrogen bonds and covalent bonds in the first PAAm network, multiple ionic bonds within the second polyelectrolyte network formed by cationic and anionic groups, and inter-network hydrogen bonds and polymer entanglement formed between these two networks. The added IPA plays a pivotal role in adjusting mechanical properties by modulating the strength of ionic bonds through polymer conformational reorganization. It also enhances the adhesive properties by attenuating the hydration effect of polymer chains. We used a high-throughput technology (HTT) to synthesize and optimize a series of hydrogels according to the preset x, y, and z values and screen their mechanical properties. The best DN polyelectrolyte hydrogel exhibits superior mechanical properties, self-adhesion, good ionic conductivity, and high sensing stability without requiring external salts. It is well-suited for multifunctional sensing and human health monitoring applications. Finally, the stability of the cross-linked first-layer PAAm network, combined with the unique properties of the polyelectrolyte that induce phase separation, ensures that the hydrogel remains stable even in saline solutions and exhibits solvent-tunable transparency (Scheme 1B). This feature has the advantages of information encryption and decryption applications.
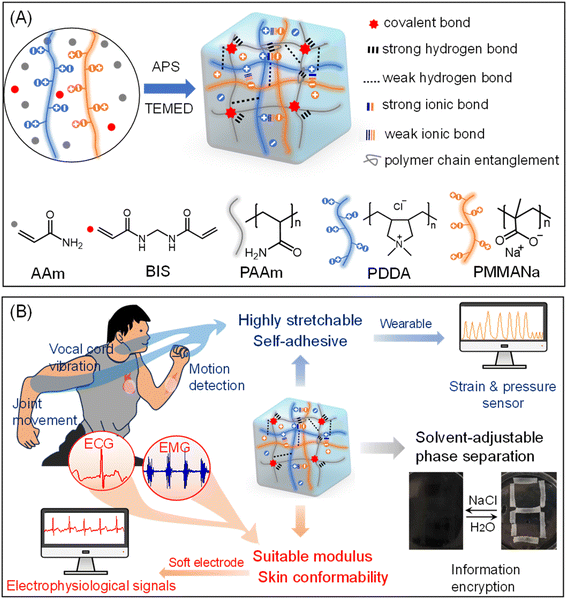 |
| Scheme 1 (A) Schematic diagram of the hydrogel preparation and structure, and (B) its applications as flexible bio-electronic sensors and information encryption. | |
2 Experimental section
2.1 Materials
Acrylamide (AAm), N,N′-methylene-bis-acrylamide (BIS), ammonium persulfate (APS), and N,N,N′,N′-tetramethyl-ethylenediamine (TEMED) were purchased from Aladdin. Poly(methacrylic acid, sodium salt) (PMAANa, Mw 4000–6000, 40 wt%) solution and poly(diallyldimethylammonium chloride) (PDDA, Mw < 100
000, 35 wt%) solution were purchased from Macklin. All chemicals were used as received. Deionized and purified water (18.2 MΩ at 25 °C) from a Milli-Q Plus water purification system (Millipore) was used throughout the experiments.
2.2 High throughput synthesis of the DN polyelectrolyte hydrogel
The stock solutions of PMAANa (40 wt%), PDDA (35 wt%), AAm (50 wt%), BIS (3.0 wt%), APS (40 wt%), TEMED (100 wt%), IPA (100 wt%), and deionized water were injected into glass vials according to the preset proportions of each component via computer-controlled syringes. The solution mixtures were mixed under stirring to form a homogeneous precursor solution. The obtained solution was injected into a customized mold, sealed with the aid of glass slides and clamps. The reaction was maintained at room temperature for 24 h. Each sample differs in PMAANa, PDDA, AAm, and IPA concentrations. Both APS and TEMED contents are 0.3 wt% to the total precursor solution for all hydrogels. The obtained DN polyelectrolyte hydrogel was denoted as xPAAm–yPMa–yPDA–zIPA, where x, y and z mean the weight percentages of AAm, polyelectrolytes and IPA, respectively.
2.3 Physical measurements
The mechanical and adhesion properties of the hydrogels were assessed using an Instron series 68TM-30 universal testing machine equipped with a 250 N force sensor, operated at room temperature and a stretching speed of 50 mm−1. For the tensile test, dumbbell-shaped hydrogels (gauge length × width × thickness = 35 × 4 × 4 mm) were prepared and securely fixed for uniaxial tensile testing. Their fracture toughness and tensile modulus were determined by calculating the area and slope of the linear portion of the stress–strain curves up to 10% strain. Lap shear strength tests were conducted with hydrogels attached to the predetermined surface for adhesion measurement. Pancake-shaped hydrogels (diameter × thickness = 10 × 2 mm) were prepared and attached to the substrate surface by overlapping the gel for 5 minutes. At least three specimens were tested for each hydrogel sample. The FT-IR spectra were obtained on a TP 920 ATR-FTIR spectrometer. Dynamic rheological measurements were performed using a TA Instruments HR 10 rheometer with a parallel plate geometry of 20 mm diameter and a gap distance set to 1000 μm. The storage modulus (G′) and loss modulus (G′′) were recorded at a frequency of 1 Hz at room temperature.
The swelling ratio (Q) values for hydrogels were determined gravimetrically. Hydrogel samples were immersed in H2O and NaCl(aq.), respectively, and the weights were recorded. The Q values for each sample could be calculated using the following formula:
where
Qm is the ratio of the swollen gel mass to the dry mass. The parameters
ρp and
ρs are the densities of polymer (1.2 g mL
−1) and water, respectively. As for the swelling test of hydrogels in NaCl(aq.) and H
2O alternatively, the hydrogels were initially immersed in NaCl(aq.) for 24 hours to reach equilibrium. After that, they were immersed in H
2O and NaCl(aq.) alternatively and the weights were recorded accordingly.
The real-time resistance change (ΔR/R0) during the stretching process was determined by the connection of the universal testing machine with the electrochemical workstation. The ΔR/R0 values were calculated using the equation ΔR/R0 = (R − R0)/R0, where R and R0 are the resistance at a certain strain and 0% strain, respectively.
3 Results and discussion
3.1 Preparation of xPAAm–yPMa–yPDA–zIPA hydrogels
Conductive double-network hydrogels, denoted as xPAAm–yPMa–yPDA–zIPA hydrogels, were synthesized by polymerizing acrylamide (AAm) in a dispersion mixture of two opposite polyelectrolytes, poly(methacrylic acid sodium) (PMAANa) and poly(diallyldimethylammonium chloride) (PDDA), along with a co-solvent (water and isopropanol (IPA)) (Scheme 1A). We employed a high-throughput technology (HTT) to precisely create a series of hydrogels according to predetermined x, y, and z values (Table S1†). Traditional hydrogel polymerization requires individual synthesis, leading to slow speed, high costs, and significant experimental errors due to manual reagent measurement. Remarkably, our HTT approach allows for the preparation of a series of samples in a short time, enhancing accuracy. In this work, we utilized HTT to prepare 24 different composited precursor dispersions within one hour, whereas the traditional method requires at least three days to achieve the same outcome.
The as-prepared hydrogel appeared opaque white, indicating structural heterogeneity within the polymer network, attributed to the non-uniform distribution of physical bonds and/or polymer chain entanglements.42,46 The hydrogel exhibits multiple physical and chemical interactions. Weak and/or strong intra-network hydrogen bonds and covalent bonds are present within the first PAAm network. In the second PMAANa–PDDA network, there are weak and/or strong electrostatic interactions between anion and cation pairs. Between the two networks, there are weak and/or strong hydrogen bonds and polymer entanglements connecting the polyelectrolytes and PAAm chains. Within the hydrogel network, polymer chains are entangled through interchain cohesion with local parallel alignment of neighboring segments47,48 and interactions between different chains in three-dimensional space during polymerization.49 It has been demonstrated that in DN hydrogels, intra- and inter-polymer chain entanglements occur both within the second network and between the first and second polymer networks.50 These entanglements form during the polymerization of the second network due to the non-uniform structure of the first network. It plays a significant role in energy dissipation, enhancing the toughness of the hydrogel. The mechanism of entanglement is as follows: during polymerization, short PAAm chains diffuse through the system, getting past the obstacle of the first network (PMa–PDA) and long PAAm chains. Two short PAAm chains with radical ends, located near long PAAm chains or the polymer chains of the first network, polymerize to generate a long chain that entangles with nearby long polymer chains.51 The formation of entanglements depends on whether the PAAm fraction exceeds its critical entanglement concentration, which is 0.5 M.52 In this study, the PAAm fraction exceeds this value. Therefore, we conclude that entanglements are present within our hydrogel.
The modulus (G′ and G′′) of the 7PMa–7PDA dispersion is higher than that of 7PMa or 7PDA alone, providing evidence for the physical interactions between oppositely charged pairs in polyelectrolytes (Fig. S1†). In the FTIR spectrum, the N–H characteristic peaks at 1608 cm−1 in pure PAAm shift to 1614 cm−1 in the 20PAAm–7PDA and 20PAAm–7PMa–7PDA hydrogels, indicating the formation of hydrogen bonds between the first PAAm network and second polyelectrolyte network (Fig. S2†). Notably, the covalent bond and polymer entanglement serve as permanent crosslinks. The strong physical interactions (ionic bonds and hydrogen bonds), stabilized by nearby polymer entanglement, serve as quasi-permanent crosslinks. Both of them impart the elasticity of gels.53 In contrast, the weak physical bonds, situated away from entangled sites, undergo reversible breaking and re-formation, dissipating energy to enhance material toughness.53
3.2 Mechanical investigation of the xPAAm–yPMa–yPDA–zIPA hydrogel
Two controlled hydrogels, a 20PAAm hydrogel based on intramolecular hydrogen bonds within the PAAm network and a 20PAAm–7PDA hydrogel consisting of both intra- and inter-network interactions, were also synthesized. The 20PAAm–7PMa–7PDA hydrogel exhibited an enhanced modulus and fracture stress attributed to multiple physical interactions and polymer chain entanglement (Fig. 1A, D, and G). The mechanical properties of hydrogels with varying PAAm contents (10 wt%, 15 wt%, and 20 wt%) were investigated to understand the influence of PAAm on hydrogel properties (Fig. S3A†). The result indicated that the hydrogel with a higher PAAm content exhibited enhanced mechanical properties, including tensile modulus, fracture strain, fracture stress, and toughness (Fig. S3B and C†). We attribute it to the high monomer concentration leading to rich polymer entanglement and pronounced strong physical interactions (hydrogen bonds) at entangled sites during the polymerization. Hence, all the hydrogels were synthesized with a PAAm concentration of 20 wt%.
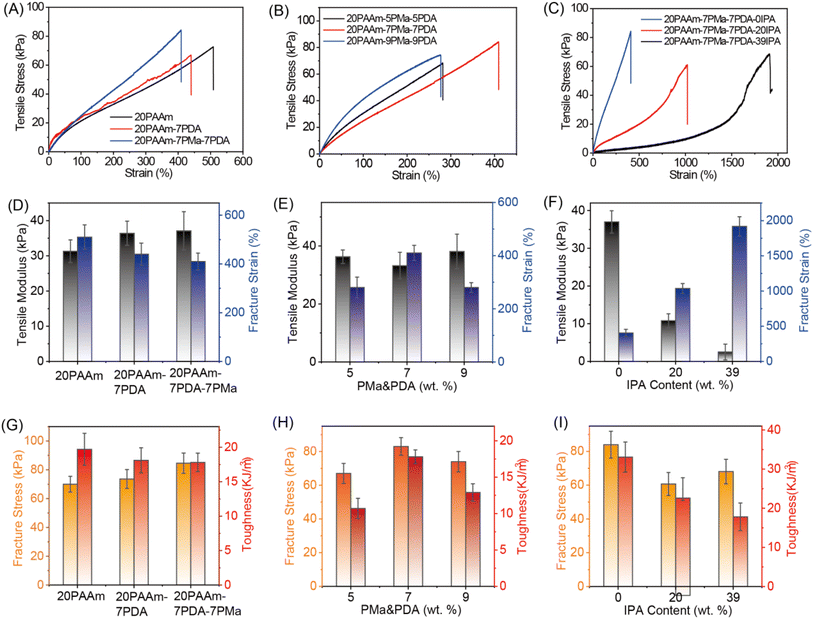 |
| Fig. 1 Tensile stress–strain curves of the hydrogels with or without a polyelectrolyte network (A), the 20PAAm–yPMa–yPDA–0IPA hydrogels with various polyelectrolyte contents (B), and 20PAAm–7PMa–7PDA–zIPA hydrogels with different IPA contents (C). (D–F) The calculated tensile modulus and fracture strain of hydrogels from (A–C). (G–I) The calculated fracture stress and toughness hydrogels from (A–C). | |
Given the critical role of hydrogen bonds and polymer chain entanglement in the PAAm network and electrostatic interaction in the polyelectrolyte network for energy dissipation, various ratios between the first and second polymer networks were explored (Fig. 1B). Normally, the gels prepared with a higher polyelectrolyte content displayed more physical interactions,53 acting as effective crosslinkers that hinder chain movement and ultimately reduce the stretchability of the gel. However, when the mass ratio of PAAm and polyelectrolytes was 20
:
7, the 20PAAm–7PMa–7PDA hydrogel demonstrated the optimal mechanical performance, with a modulus of 33.2 kPa and the largest strain value of 410% (Fig. 1E). The fracture stress and toughness of the 20PAAm–7PMa–7PDA hydrogel were higher than those of the 20PAAm–5PMa–5PDA and 20PAAm–9PMa–9PDA hydrogels (Fig. 1H). This may be because less chain entanglement results in physical interactions (ionic and hydrogen bonds) being dominated by weak bonds in this composition of gels. Similar trends were observed in hydrogels prepared with two different molecular weights of PDDA (Mw 10–20 W and Mn > 40 W, respectively) (Table S1†).
To further enhance the stretchability of the hydrogel, we used IPA as an ionic bond regulator, which acts as a poor solvent for polyelectrolytes, to adjust the energy of the ionic bonds in the network. It has been proven that a flexible chain of random coil conformation occurs in a good solvent, whereas in a poor solvent, it tends to form a globular conformation.54 Weak ionic bonds tend to form in globule conformations, while strong ionic bonds tend to form in coil conformations.53 Besides, polyelectrolytes have a higher affinity for water, and their ionization capacity will reduce in organic solvents (e.g., IPA).55,56 The hydrogel becomes softer with weaker electrostatic interactions. The N–H peaks at 1614 cm−1 and quaternary ammonium groups at 966 cm−1 of 20PAAm–7PMa–7PDA hydrogels shift to 1608 cm−1 and 973 cm−1, respectively, in the 20PAAm–7PMa–7PDA–20IPA hydrogels, indicating that IPA affects the formation of hydrogen bonds and ionic bonds (Fig. S2†). The weaker physical interaction leads to a decrease in both the G′ and G′′ values of 20PAAm–7PMa–7PDA–20IPA hydrogels compared to those of the 20PAAm–7PMa–7PDA hydrogels (Fig. S4†). It is observed that as the IPA content increases, the gel becomes softer, with a decrease in modulus, fracture stress, and toughness, and an increase in fracture strain for the 20PAAm–7PMa–7PDA–zIPA (Fig. 1C, F and I). This trend is also observed in the 20PAAm–5PMa–5PDA–zIPA hydrogel (Fig. S5†). The 20PAAm–7PMa–7PDA–39IPA hydrogel can be stretched to nearly 20 times its original length. Considering the appropriate modulus for soft electronics, the hydrogels in this study were prepared with an IPA content of 20 wt%. The modulus and fracture strain of the 20PAAm–7PMa–7PDA–20IPA hydrogel are 10.8 kPa and 1000%, respectively.
3.3 Adhesion performance of the xPAAm–yPMa–yPDA–zIPA hydrogel
The adhesive properties of the hydrogels were investigated, with a primary focus on studying their adhesion strength on glass surfaces to assess their overall adhesiveness. The results suggest a strong correlation between the adhesive properties of the hydrogels and the concentrations of polyelectrolytes and IPA. Notably, the adhesion properties of the 20PAAm–7PMa–7PDA hydrogel outperformed those of the 20PAAm–5PMa–5PDA and 20PAAm–9PMa–9PDA hydrogels, attributed to the synergistic effects of hydrogen bonding and electrostatic interaction (Fig. 2A and S6A†). The detrimental effect of IPA on ionic interaction resulted in a significantly lower adhesion strength for the 20PAAm–5PMa–5PDA–zIPA hydrogel containing IPA (z < 39 wt%) compared to that without IPA. Interestingly, the adhesive capability of the hydrogels improved when the IPA content ranged between 15 and 39 wt% (Fig. 2B and S6B†). The adhesiveness of our hydrogels mainly stems from the following factors: the abundance of ionic groups in polyelectrolytes facilitating electrostatic interactions with substrates (such as the ionic bond between –COO− and metal ions from iron, as well as –N+ and –O− on glass or –COO− of tissue), metal complexation between the –C
O group from the polymer and the metal ions, and hydrogen bonding between the gel surface (both the polymer chain and IPA) and the groups on the substrates (Fig. 2F). The other factor is the presence of a large number of hydroxyl groups in IPA, which reduces the hydration effect around polar groups in polymer chains, such as carboxyl and amide groups.57 In this 20PAAm–5PMa–5PDA–39IPA hydrogel, the impact of IPA exceeds that of ionic groups, resulting in the highest adhesion strength of 65.27 kPa on glass slides. Benefiting from the synergistic effect of ionic bonds from polyelectrolytes and hydrogen bonds from IPA, the 20PAAm–7PMa–7PDA–20IPA hydrogels demonstrated strong adhesion to various solid surfaces, including rubber gloves (Nitrile Butadiene Rubber (NBR)), iron, paper, pigskin, and glasses, with excellent adhesion strength values mostly exceeding 30 kPa (Fig. 2C–E). Particularly noteworthy is the adhesion strength of this composite hydrogel on pigskin (19.2 kPa), proving sufficient for applications in wearable flexible electronic sensing. In comparison with other polyelectrolyte hydrogels,37,58–62 our 20PAAm–7PMa–7PDA–20IPA hydrogel exhibited suitable mechanical properties (high tensile strain and a modulus similar to those of human skin) and superior adhesion performance for bioelectronic sensing.
 |
| Fig. 2 The adhesion strength of the 20PAAm–yPMa–yPDA–0IPA hydrogels with various polyelectrolyte contents (A) and the 20PAAm–5PMa–5PDA–zIPA hydrogels with different IPA contents on the glass surface (B). Lap shear adhesive curve (C), adhesion strength (D), and photograph (E) of the 20PAAm–7PMa–7PDA–20IPA hydrogels on various substrates. The scale bar in (E) is applied to all images. (F) Schematic illustration of the adhesive mechanism between 20PAAm–7PMa–7PDA–20IPA hydrogels and various substrates. | |
3.4 Dynamic changes in the conductivity of 20PAAm–7PMa–7PDA–20IPA hydrogels
The 20PAAm–7PMa–7PDA–20IPA hydrogel displays excellent conductivity due to the inclusion of NaCl. The inherent features of stretchability and high ionic conductivity render these hydrogels highly suitable for flexible applications, such as strain sensors. The soft performance improves sensing sensitivity and enables low detection limits for flexible sensors.63 The observed resistance change (ΔR/R0) in the 20PAAm–7PMa–7PDA–20IPA hydrogels during stretching is attributed to alterations in the radial structure of the hydrogel, which elongates the channels for ions within the gel network during the stretching process. Initial strain sensing experiments were conducted during uniaxial stretching to evaluate the sensitivity of resistance variation.
As depicted in Fig. 3A, the conductive hydrogel can stretch up to 1100% strain before reaching the breaking point. The gauge factor (GF), defined as the slope of the resistance versus tensile strain curve, ranges from 1.29 to 3.77 for strain ranges of 0–150%, 150–400%, and 400–1000%, respectively. The resistance of the hydrogel increases upon stretching, returning quickly to the initial state after release. Fig. 3B–D demonstrate the sensing reversibility and stability of gels across various strain ranges (from 0.5% to 450%). The soft flexibility of the 20PAAm–7PMa–7PDA–20IPA hydrogel enables high sensitivity even at super-small strain levels (0.5%). Noteworthy for its minimal hysteresis and rapid self-recovery, the hydrogel maintains stable sensing performance in the cyclic loading–unloading process, regardless of constant or varying strain rates (10–400 mm min−1) (Fig. 3E). Importantly, the conductive hydrogels exhibit good stability and repeatability, showing negligible signal attenuation with approximately 250 stretching–releasing cycles at 100% strain (Fig. 3F). After undergoing this cyclic process, signal fluctuations were observed, likely due to the hysteresis of the gels during the loading–unloading process (Fig. S7†). These performances demonstrate the accuracy and reliability of 20PAAm–7PMa–7PDA–20IPA hydrogels as strain sensors. It is noteworthy that the gel consistently produced stable signals even after exposure to water and high temperature (40 °C) (Fig. S8†). The excellent underwater conductive stability of our hydrogels is attributed to the internal ion of polyelectrolytes preventing severe leakage of free ions from the hydrogel network when immersed in water or at high temperatures. Although the hydrogel is inevitably prone to dehydration and may lose stability in the long term due to high water content, an effective method has been offered to prevent dehydration by modifying or covering the hydrogel surface with a thin elastomer layer.64
 |
| Fig. 3 (A) Tensile strain induced relative resistance changes (ΔR/R0) of the 20PAAm–7PMa–7PDA–20IPA hydrogels. The ΔR/R0 values were collected with different strain levels at a speed of 50 mm min−1 (B–D) and different frequencies at 100% strain (E). (F) Cyclic measurement of the hydrogels at 100% strain and a speed of 200 mm min−1. | |
3.5 The 20PAAm–7PMa–7PDA–20IPA hydrogels applied on a flexible sensor to monitor human motions, EMGs, and ECGs
Due to their excellent adhesive properties, the 20PAAm–7PMa–7PDA–20IPA hydrogels can be directly affixed to various locations such as fingers and wrists, enabling the detection of human motions through observed conductivity changes during multiple deformations. The resistance of the hydrogel sensor changed as the finger or wrist bent to different angles (20°, 45°, and 90°), and it efficiently restored to its original levels as the finger or wrist gradually straightened (Fig. 4A and B). Moreover, the output signal intensities varied noticeably with changes in motion frequency, indicating real-time acquisition of electrophysiological signals (Fig. 4B). Based on the soft compliance feature, the 20PAAm–7PMa–7PDA–20IPA hydrogels exhibited high sensitivity to subtle electrical resistance changes caused by weak strains detected at the throat. This sensitivity enables the recording of minute muscle movements during speech, as demonstrated by differentiable and repeatable characteristic signals observed when the volunteer pronounced various words such as “hydrogel”, “good paper”, and “welcome” (Fig. 4C). Next, the conductive hydrogel was applied to the neck to capture the varying electrical signals reflecting neck movement and head bowing, facilitating health monitoring. Given the prevalent use of mobile phones and prolonged desk work, maintaining a prolonged downward head posture is common and may lead to severe cervical spine diseases. Bending the neck stretches the hydrogel, elongating the ion transmission pathway and consequently increasing resistance (Fig. 4D). Applying a conductive hydrogel to the neck for continuous output of reliable signals can serve as a reminder for individuals to take breaks, thus preventing unforeseen neck injuries. Hence, the 20PAAm–7PMa–7PDA–20IPA hydrogel-based sensor demonstrates great sensitivity and rapid response, meeting the requirements for human monitoring. In addition, a flexible writing pad for English letter recognition was designed by assembling the hydrogel with VHB tape and connecting it to the experimental apparatus with wires on both sides (Fig. 4E, insert). As depicted in Fig. 4E, various letters (C, A, and T) produced unique signals reflected in the resistance change, showcasing the hydrogel's potential in information encryption and transmission.
 |
| Fig. 4 Real-time monitoring of the finger (A) and wrist (B) bending, identification of different words by the hydrogel sensor through micro-vibrations in throat muscles (C), and detection of neck motion (D) using strain sensing with 20PAAm–7PMa–7PDA–20IPA hydrogels. The scale bar in (A) is applied to all images. (E) The 20PAAm–7PMa–7PDA–20IPA hydrogels were assembled into pressure sensors for recognizing the handwriting of different letters. | |
Electrocardiograms (ECGs) and electromyograms (EMGs) are crucial electrophysiological signals in humans for diagnosing potential cardiovascular-/muscle-associated diseases.65–67 We believe that the prepared 20PAAm–7PMa–7PDA–20IPA hydrogel shows potential as an ideal epidermal electrode for detecting electrophysiological signals. Commercial EMG electrodes were replaced with a conductive 220PAAm–7PMa–7PDA–20IPA hydrogel (diameter = 20 mm). Due to its excellent flexibility and adhesiveness, the hydrogel electrode seamlessly adhered to the volar and elbow of the volunteer's left forearm even after multiple uses, while commercial electrodes, suffering from deformation and reduced adhesion, cannot fit the skin completely (Fig. 5A and B). Muscle signals were detected when clenching the right fist, disappearing immediately upon releasing the fist (Fig. 5C). We observed that the EMG signals from the hydrogel electrode exhibited a better signal-to-noise ratio compared to commercial electrodes, possibly due to its superior skin conformability and lower interface impedance68 (Fig. S9†). Furthermore, the EMG signals exhibit high sensitivity to muscle movement, enabling differentiation of specific finger actions. Fig. 5D and E demonstrate that different finger movements generate distinct signals, which facilitate the remote identification of robotic hands in the future.
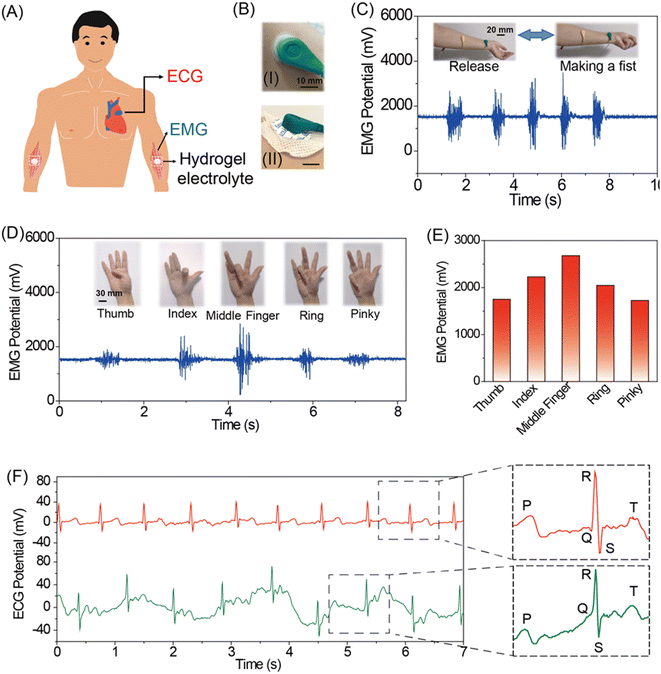 |
| Fig. 5 (A) Schematic illustration of monitoring ECG and EMG signals using a portable sensor with hydrogel electrolyte. (B) Photos demonstrating the attachment of 20PAAm–7PMa–7PDA–20IPA hydrogel electrolyte (i) and commercial electrolyte (ii) on the skin for signal detection after multiple uses. (C) The recorded EMG signals during fist clenches and releases. EMG signals (D) and their intensity (E) produced by the flexion/extension of different fingers. (F) The ECG signals collected from the hydrogel electrolyte pad during periods of rest and exercise. | |
Heart monitoring is vital for health tracking, yet traditional measuring devices are frequently bulky or costly. Here, we developed a portable sensor based on the 20PAAm–7PMa–7PDA–20IPA hydrogel, capable of real-time tracking and transmission of electrocardiographic (ECG) signals to a computer, eliminating the need for cumbersome auxiliary equipment. The detected characteristic peaks (P, Q, R, S, and T waves) closely match standard signals, suggesting a healthy cardiac condition in the volunteers. The sensor underwent evaluation during various human activities, including resting and exercising (Fig. 5F). The obtained ECG signals varied but remained stable, demonstrating the reliability of the 20PAAm–7PMa–7PDA–20IPA hydrogel electrode. Furthermore, it consistently recorded electrocardiogram signals even after being reapplied 10 times (Fig. S10†), proving the suitability of the gel pad for long-term wear. These findings imply that the 20PAAm–7PMa–7PDA–20IPA hydrogel electrode pad is capable of acquiring high-quality ECG signals.
3.6 The 20PAAm–7PMa–7PDA–20IPA hydrogels for information encryption and decryption
The optical properties of 20PAAm–7PMa–7PDA–20IPA hydrogels have been studied. The 20PAAm–7PMa–7PDA–20IPA hydrogels exhibited solvent-adjustable transparency due to the heterogeneous structure within the matrix.42 The heterogeneous structure in the opaque white as-prepared hydrogels arises from the uneven distribution of COO−–N+ bonds and polymer chain entanglements. Upon immersion in 10 wt% NaCl(aq.), the COO− and N+ groups coupled with Na+ and Cl− respectively, disrupting the ionic bonds and heterogeneous structure, gradually leading to hydrogel transparency (Fig. 6A). Upon re-immersion in water, paired ions permeate the exterior of the hydrogel due to the osmotic effect, leading to the reformation of COO−–N+ ionic bonds. The hydrophilic ionic bonds in the side groups of the polyelectrolyte chain promote the aggregation of hydrophobic main polymer chains, resulting in phase separation. Transparency changes are reversible and detected through transmittance measurements (Fig. 6B). Initially, an opaque white layer forms on the surface, gradually penetrating the interior of the gel over extended immersion, indicating an increase in the content of the phase-separated structure during dialysis (Fig. 6C).
 |
| Fig. 6 (A) Images of 20PAAm–7PMa–7PDA–20IPA hydrogels in opaque white and transparent colors during immersion in NaCl(aq.) and H2O, respectively, and the schematic diagram of dynamic changes of ionic bonds. (B) Transmittance variation at 500 nm of 20PAAm–7PMa–7PDA–20IPA hydrogels during cyclic treatment with NaCl(aq.) and H2O measured by UV-vis. (C) Images showing the time-dependent transparency of 20PAAm–7PMa–7PDA–20IPA hydrogels immersed in NaCl(aq.) (first row) and H2O (second row). (D) Application in information encryption and decryption. The scale bar is 5 mm and applied to all images. | |
Swelling tests provide evidence of the formation and deformation of the ionic network. Higher cross-linked polymer networks exhibit lower swelling ratios (Q), and vice versa. The Q values of 20PAAm–7PMa–7PDA–20IPA hydrogels in NaCl(aq.) are significantly higher than that in deionized water, reflecting the dissociation of the COO−–N+ bonds of the gel network in NaCl(aq.). The ionically cross-linked sites re-form in water, resulting in a smaller Q value. A cyclic swelling test of the 20PAAm–7PMa–7PDA–20IPA hydrogel alternating between NaCl(aq.) and H2O was conducted (Fig. S11A†). The gel retains its original shape even after multiple cycles due to the elasticity of the non-dissociative chemical crosslinking, and the Q value in NaCl(aq.) is higher than that in H2O in each cycle, demonstrating that the disrupting and recovery of ionic bonds can occur at least 5 times (Fig. S11B†). This inspired us to design a strategy using solvent modulation to reveal information hidden within the hydrogel, thereby realizing information encryption and decryption. As a conceptual verification, an “8” shape of the 20PAAm–7PMa–7PDA–20IPA hydrogel appears white and observable. During encryption, the digit “8” is entirely concealed in NaCl(aq.), and the information reappears in water. This information processing process can be easily accomplished by changing the solvent, showing its potential application in information anti-counterfeiting and transmission.
We conducted a comprehensive performance comparison between our hydrogel sensor and previously reported conductive hydrogels (Table S2†). Current methods for fabricating conductive hydrogels involve introducing electrically conductive materials, salt ions, or polyelectrolytes into the gel matrix. Most electrically conductive materials require pre-synthesis and often rely on complex modifications to enhance dispersion uniformity and address the issue of low GF values. The GF value can be improved easily for gels containing salt ions, as it is positively correlated with ion concentration. However, such ionic gels suffer from ion leakage issues. Additionally, both of the above approaches require the introduction of adhesive groups (such as catechol15,69) to impart adhesion and lack solvent-induced phase transition capabilities. Although currently developed polyelectrolyte hydrogels offer advantages such as simple preparation, low cost, excellent conductivity, and solvent responsiveness, their adhesive strength and conductive performance (GF values and working range) remain limited. The hydrogels developed in this study demonstrate superior overall performance in terms of mechanical properties, adhesion, conductivity, and transparency transition, making them well-suited for applications in bioelectronic sensing and information encryption.
4 Conclusion
In this study, we developed a DN polyelectrolyte hydrogel characterized by multiple types of interactions, including chain entanglement, strong or/and weak physical bonds (hydrogen bonds and ionic bonds), and chemical bonds. Leveraging this unique network architecture and intricate intermolecular interactions, we systematically adjusted the content of polymer chains in the two-layer network and the dispersion phase (IPA) to fine-tune the strength of physical bonds. The fabricated hydrogels show exceptional mechanical properties (a tensile modulus of 10.8 kPa and a tensile fracture strain of 1000%) and adhesive strength (adhesive strengths of 37.8 kPa and 19.2 kPa to glass and pigskin, respectively). Moreover, due to the inherent conductivity of polyelectrolytes, the hydrogels can be applied as highly sensitive mechanical sensors to sensitively monitor human joint movements or subtle throat vibrations for word recognition with remarkable stability. The hydrogels can also function as soft electrodes to assemble wearable sensors for continuous monitoring of the electro-physiological signals, including EMG and ECG. It is noteworthy that their solvent-adjustable transparency further renders them suitable for information encryption and decryption. Overall, the developed DN polyelectrolyte hydrogel represents a versatile platform for various multifaceted applications.
Data availability
The authors confirm that the data supporting the findings of this study are available within the article and its ESI.†
Author contributions
Xiaodong Xiang and Dongdong Lu designed the experiments and guided the article writing – review & editing. Zilong Zhu and Dongdong Lu conducted the experiments and analyzed the data related to hydrogel synthesis and physical characterization. Mingning Zhu assisted with the experiments monitoring human motions, EMGs, and ECGs. Peng Zhang helped with data analysis. All authors reviewed the manuscript.
Conflicts of interest
The authors declare no competing financial interest.
Acknowledgements
This work was supported by the Shenzhen Fundamental Research Funding (JCYJ20220530113802006, JCYJ20220818100613028, and JCYJ20220818100612027), the Guangdong Basic and Applied Basic Research Foundation (2023A1515110012), the Guangdong Natural Science Foundation (2023A1515012680), the National Natural Science Foundation of China (22305047 and 12204220) and the Major Science and Technology Infrastructure Project of the Material Genome Big-Science Facilities Platform supported by the Municipal Development and Reform Commission of Shenzhen.
References
- Y. Liu, M. Pharr and G. A. Salvatore, Lab-on-skin: a review of flexible and stretchable electronics for wearable health monitoring, ACS Nano, 2017, 11(10), 9614–9635 CrossRef CAS PubMed.
- W. Gao, H. Ota, D. Kiriya, K. Takei and A. Javey, Flexible electronics toward wearable sensing, Acc. Chem. Res., 2019, 52(3), 523–533 CrossRef CAS PubMed.
- X. Wang, Z. Liu and T. Zhang, Flexible sensing electronics for wearable/attachable health monitoring, Small, 2017, 13(25), 1602790 CrossRef PubMed.
- U. STERN, Electronic skin: From flexibility to a sense of touch, Nature, 2021, 591, 685 CrossRef.
- G. Schwartz, B. C.-K. Tee, J. Mei, A. L. Appleton, D. H. Kim, H. Wang and Z. Bao, Flexible polymer transistors with high pressure sensitivity for application in electronic skin and health monitoring, Nat. Commun., 2013, 4(1), 1859 CrossRef PubMed.
- Y. Lee, W. Song and J.-Y. Sun, Hydrogel soft robotics, Mater. Today Phys., 2020, 15, 100258 CrossRef.
- J. Li, J. Cao, B. Lu and G. Gu, 3D-printed PEDOT: PSS for soft robotics, Nat. Rev. Mater., 2023, 8(9), 604–622 CrossRef.
- S. Naghdi, K. Y. Rhee, D. Hui and S. J. Park, A review of conductive metal nanomaterials as conductive, transparent, and flexible coatings, thin films, and conductive fillers: Different deposition methods and applications, Coatings, 2018, 8(8), 278 CrossRef.
- S. Liu, M. C. Yuen, E. L. White, J. W. Boley, B. Deng, G. J. Cheng and R. Kramer-Bottiglio, Laser sintering of liquid metal nanoparticles for scalable manufacturing of soft and flexible electronics, ACS Appl. Mater. Interfaces, 2018, 10(33), 28232–28241 CrossRef CAS PubMed.
- A. Razaq, F. Bibi, X. Zheng, R. Papadakis, S. H. M. Jafri and H. Li, Review on graphene-, graphene oxide-, reduced graphene oxide-based flexible composites: From fabrication to applications, Materials, 2022, 15(3), 1012 CrossRef CAS PubMed.
- S. Park, M. Vosguerichian and Z. Bao, A review of fabrication and applications of carbon nanotube film-based flexible electronics, Nanoscale, 2013, 5(5), 1727–1752 RSC.
- Y. Yu, Y. Feng, F. Liu, H. Wang, H. Yu, K. Dai, G. Zheng and W. Feng, Carbon Dots-Based Ultrastretchable and Conductive Hydrogels for High-Performance Tactile Sensors and Self-Powered Electronic Skin, Small, 2023, 19(31), 2204365 CrossRef CAS PubMed.
- Z. Wang, F. Cheng, H. Cai, X. Li, J. Sun, Y. Wu, N. Wang and Y. Zhu, Robust versatile nanocellulose/polyvinyl alcohol/carbon dot hydrogels for biomechanical sensing, Carbohydr. Polym., 2021, 259, 117753 CrossRef CAS PubMed.
- S. P. Sreenilayam, I. U. Ahad, V. Nicolosi and D. Brabazon, MXene materials based printed flexible devices for healthcare, biomedical and energy storage applications, Mater. Today, 2021, 43, 99–131 CrossRef CAS.
- Y. Feng, H. Liu, W. Zhu, L. Guan, X. Yang, A. V. Zvyagin, Y. Zhao, C. Shen, B. Yang and Q. Lin, Muscle-inspired MXene conductive hydrogels with anisotropy and low-temperature tolerance for wearable flexible sensors and arrays, Adv. Funct. Mater., 2021, 31(46), 2105264 CrossRef CAS.
- H. Lu, X. Li and Q. Lei, Conjugated conductive polymer materials and its applications: a mini-review, Front. Chem., 2021, 9, 732132 CrossRef CAS PubMed.
- S. Inal, J. Rivnay, A.-O. Suiu, G. G. Malliaras and I. McCulloch, Conjugated polymers in bioelectronics, Acc. Chem. Res., 2018, 51(6), 1368–1376 CrossRef CAS PubMed.
- C. Larson, B. Peele, S. Li, S. Robinson, M. Totaro, L. Beccai, B. Mazzolai and R. Shepherd, Highly stretchable electroluminescent skin for optical signaling and tactile sensing, Science, 2016, 351(6277), 1071–1074 CrossRef CAS PubMed.
- X. Wang, X. Wang, M. Pi and R. Ran, High-strength, highly conductive and woven organic hydrogel fibers for flexible electronics, Chem. Eng. J., 2022, 428, 131172 CrossRef CAS.
- Y.-X. Qu, Q.-Q. Xia, L.-T. Li, C.-F. Cao, G.-D. Zhang, P. Castignolles, J. Bae, P. Song, J.-F. Gao and L.-C. Tang, Rational Design of Oil-Resistant and Electrically Conductive Fluorosilicone Rubber Foam Nanocomposites for Sensitive Detectability in Complex Solvent Environments, ACS Nano, 2024, 18(33), 22021–22033 CrossRef CAS PubMed.
- Z. F. Zeng, Y. Q. Yang, X. W. Pang, B. Jiang, L. X. Gong, Z. Liu, L. Peng and S. N. Li, Lignin Nanosphere-Modified MXene Activated-Rapid Gelation of Mechanically Robust, Environmental Adaptive, Highly Conductive Hydrogel for Wearable Sensors Application, Adv. Funct. Mater., 2024, 2409855 CrossRef.
- L. Han, L. Yan, M. Wang, K. Wang, L. Fang, J. Zhou, J. Fang, F. Ren and X. Lu, Transparent, adhesive, and conductive hydrogel for soft bioelectronics based on light-transmitting polydopamine-doped polypyrrole nanofibrils, Chem. Mater., 2018, 30(16), 5561–5572 CrossRef CAS.
- X. Liu, Q. Zhang and G. Gao, Solvent-resistant and nonswellable hydrogel conductor toward mechanical perception in diverse liquid media, ACS Nano, 2020, 14(10), 13709–13717 CrossRef CAS PubMed.
- X. Pei, H. Zhang, Y. Zhou, L. Zhou and J. Fu, Stretchable, self-healing and tissue-adhesive zwitterionic hydrogels as strain sensors for wireless monitoring of organ motions, Mater. Horiz., 2020, 7(7), 1872–1882 RSC.
- C. Wang, Y. Liu, X. Qu, B. Shi, Q. Zheng, X. Lin, S. Chao, C. Wang, J. Zhou and Y. Sun, Ultra-stretchable and fast self-healing ionic hydrogel in cryogenic environments for artificial nerve fiber, Adv. Mater., 2022, 34(16), 2105416 CrossRef CAS PubMed.
- J. Yin, C. Lu, C. Li, Z. Yu, C. Shen, Y. Yang, X. Jiang and Y. Zhang, A UV-filtering, environmentally stable, healable and recyclable ionic hydrogel towards multifunctional flexible strain sensor, Composites, Part B, 2022, 230, 109528 CrossRef CAS.
- M. Kaltenbrunner, T. Sekitani, J. Reeder, T. Yokota, K. Kuribara, T. Tokuhara, M. Drack, R. Schwödiauer, I. Graz, S. Bauer-Gogonea, S. Bauer and T. Someya, An ultra-lightweight design for imperceptible plastic electronics, Nature, 2013, 499(7459), 458–463 CrossRef CAS PubMed.
- L. Geng, S. Hu, M. Cui, J. Wu, A. Huang, S. Shi and X. Peng, Muscle-inspired double-network hydrogels with robust mechanical property, biocompatibility and ionic conductivity, Carbohydr. Polym., 2021, 262, 117936 CrossRef CAS PubMed.
- S. Pan, M. Xia, H. Li, X. Jiang, P. He, Z. Sun and Y. Zhang, Transparent, high-strength, stretchable, sensitive and anti-freezing poly (vinyl alcohol) ionic hydrogel strain sensors for human motion monitoring, J. Mater. Chem. C, 2020, 8(8), 2827–2837 RSC.
- X. Zhang, K. Wang, J. Hu, Y. Zhang, Y. Dai and F. Xia, Role of a high calcium ion content in extending the properties of alginate dual-crosslinked hydrogels, J. Mater. Chem. A, 2020, 8(47), 25390–25401 RSC.
- D. Silvaraj, S. Bashir, M. Hina, J. Iqbal, S. Gunalan, S. Ramesh and K. Ramesh, Tailorable solid-state supercapacitors based on poly (N-hydroxymethylacrylamide) hydrogel electrolytes with high ionic conductivity, J. Energy Storage, 2021, 35, 102320 CrossRef.
- S. Wu, M. Hua, Y. Alsaid, Y. Du, Y. Ma, Y. Zhao, C. Y. Lo, C. Wang, D. Wu and B. Yao, Poly (vinyl alcohol) hydrogels with broad-range tunable mechanical properties via the Hofmeister effect, Adv. Mater., 2021, 33(11), 2007829 CrossRef CAS.
- M. Hua, S. Wu, Y. Ma, Y. Zhao, Z. Chen, I. Frenkel, J. Strzalka, H. Zhou, X. Zhu and X. He, Strong tough hydrogels via the synergy of freeze-casting and salting out, Nature, 2021, 590(7847), 594–599 CrossRef CAS PubMed.
- Z. Lei and P. Wu, A highly transparent and ultra-stretchable conductor with stable conductivity during large deformation, Nat. Commun., 2019, 10(1), 3429 CrossRef PubMed.
- Y. Zhao, J. Kang and T. Tan, Salt-, pH-and temperature-responsive semi-interpenetrating polymer network hydrogel based on poly (aspartic acid) and poly (acrylic acid), Polymer, 2006, 47(22), 7702–7710 CrossRef CAS.
- Q. Wang, X. Pan, C. Lin, X. Ma, S. Cao and Y. Ni, Ultrafast gelling using sulfonated lignin-Fe3+ chelates to produce dynamic crosslinked hydrogel/coating with charming stretchable, conductive, self-healing, and ultraviolet-blocking properties, Chem. Eng. J., 2020, 396, 125341 CrossRef CAS.
- W. J. Yang, R. Zhang, X. Guo, R. Ma, Z. Liu, T. Wang and L. Wang, Supramolecular polyelectrolyte hydrogel based on conjoined double-networks for multifunctional applications, J. Mater. Chem. A, 2022, 10(44), 23649–23665 RSC.
- A. B. Ihsan, T. L. Sun, T. Kurokawa, S. N. Karobi, T. Nakajima, T. Nonoyama, C. K. Roy, F. Luo and J. P. Gong, Self-healing behaviors of tough polyampholyte hydrogels, Macromolecules, 2016, 49(11), 4245–4252 CrossRef.
- E. Su and O. Okay, Polyampholyte hydrogels formed via electrostatic and hydrophobic interactions, Eur. Polym. J., 2017, 88, 191–204 CrossRef CAS.
- T. L. Sun, T. Kurokawa, S. Kuroda, A. B. Ihsan, T. Akasaki, K. Sato, M. A. Haque, T. Nakajima and J. P. Gong, Physical hydrogels composed of polyampholytes demonstrate high toughness and viscoelasticity, Nat. Mater., 2013, 12(10), 932–937 CrossRef CAS PubMed.
- K. Cui, T. L. Sun, X. Liang, K. Nakajima, Y. N. Ye, L. Chen, T. Kurokawa and J. P. Gong, Multiscale energy dissipation mechanism in tough and self-healing hydrogels, Phys. Rev. Lett., 2018, 121(18), 185501 CrossRef PubMed.
- K. Cui, Y. N. Ye, T. L. Sun, C. Yu, X. Li, T. Kurokawa and J. P. Gong, Phase separation behavior in tough and self-healing polyampholyte hydrogels, Macromolecules, 2020, 53(13), 5116–5126 CrossRef CAS.
- F. Luo, T. L. Sun, T. Nakajima, T. Kurokawa, Y. Zhao, K. Sato, A. B. Ihsan, X. Li, H. Guo and J. P. Gong, Oppositely charged polyelectrolytes form tough, self-healing, and rebuildable hydrogels, Adv. Mater., 2015, 27(17), 2722–2727 CrossRef CAS PubMed.
- S. Wu, M. Zhu, D. Lu, A. H. Milani, Q. Lian, L. A. Fielding, B. R. Saunders, M. J. Derry, S. P. Armes and D. Adlam, Self-curing super-stretchable polymer/microgel complex coacervate gels without covalent bond formation, Chem. Sci., 2019, 10(38), 8832–8839 RSC.
- S. Li, H. Zhou, Y. Li, X. Jin, H. Liu, J. Lai, Y. Wu, W. Chen and A. Ma, Mussel-inspired self-adhesive hydrogels by conducting free radical polymerization in both aqueous phase and micelle phase and their applications in flexible sensors, J. Colloid Interface Sci., 2022, 607, 431–439 CrossRef CAS PubMed.
- J. Wang, K. Cui, B. Zhu, J. P. Gong, C.-Y. Hui and A. T. Zehnder, Load transfer between permanent and dynamic networks due to stress gradients in nonlinear viscoelastic hydrogels, Extreme Mech. Lett., 2023, 58, 101928 CrossRef.
- R. Qian, L. Wu, D. Shen, D. H. Napper, R. A. Mann and D. F. Sangster, Single-chain polystyrene glasses, Macromolecules, 1993, 26(11), 2950–2953 CrossRef CAS.
- R. Qian, The concept of cohesional entanglement, Macromol. Symp., 1997, 15–26 CrossRef CAS.
- K. Iwata, Topological Theory of Entanglement: A Polymer Chain and a Fixed Barrier. I. Diffusion Equation, J. Phys. Soc. Jpn., 1974, 37(5), 1413–1422 CrossRef.
- H. Tsukeshiba, M. Huang, Y.-H. Na, T. Kurokawa, R. Kuwabara, Y. Tanaka, H. Furukawa, Y. Osada and J. P. Gong, Effect of polymer entanglement on the toughening of double network hydrogels, J. Phys. Chem. B, 2005, 109(34), 16304–16309 CrossRef CAS PubMed.
- M. Huang, H. Furukawa, Y. Tanaka, T. Nakajima, Y. Osada and J. P. Gong, Importance of entanglement between first and second components in high-strength double network gels, Macromolecules, 2007, 40(18), 6658–6664 CrossRef CAS.
- W.-M. Kulicke, R. Kniewske and J. Klein, Preparation, characterization, solution properties and rheological behaviour of polyacrylamide, Prog. Polym. Sci., 1982, 8(4), 373–468 CrossRef CAS.
- T. L. Sun, F. Luo, T. Kurokawa, S. N. Karobi, T. Nakajima and J. P. Gong, Molecular structure of self-healing polyampholyte hydrogels analyzed from tensile behaviors, Soft Matter, 2015, 11(48), 9355–9366 RSC.
-
M. Rubinstein and R. Colby, Polymer Physics, Oxford University Press, New York, 2003 Search PubMed.
- G. Liu, S. Liu, K. Ma, H. Wang, X. Wang, G. Liu and W. Jin, Polyelectrolyte functionalized ti2ct x mxene membranes for pervaporation dehydration of isopropanol/water mixtures, Ind. Eng. Chem. Res., 2020, 59(10), 4732–4741 CrossRef CAS.
- Y. Wibisono, E. T. Anggraeni, B. D. Argo, W. A. Nugroho, I. K. Maharsih and M. R. Bilad, Environmentally-safe anion exchange membranes of PVA/PDDA/SiO2composite for reverse electrodialysis, Int. J. Thermofluids, 2023, 18, 100350 CrossRef CAS.
- H. Zhou, J. Lai, B. Zheng, X. Jin, G. Zhao, H. Liu, W. Chen, A. Ma, X. Li and Y. Wu, From glutinous-rice-inspired adhesive organohydrogels to flexible electronic devices toward wearable sensing, power supply, and energy storage, Adv. Funct. Mater., 2022, 32(1), 2108423 CrossRef CAS.
- W. Wang, H. Song, Z. Lu, Q. Zeng, Q. Wang, C. Ma, H. Jin, J. Qi, T. Wu and S. Gao, Mussel Byssus Inspired Ionic Skin with Damage-Resistant Signal for Human–Machine Interaction, Adv. Mater. Interfaces, 2022, 9(34), 2201367 CrossRef CAS.
- Z. Zhang, Z. Gao, Y. Wang, L. Guo, C. Yin, X. Zhang, J. Hao, G. Zhang and L. Chen, Eco-friendly, self-healing hydrogels for adhesive and elastic strain sensors, circuit repairing, and flexible electronic devices, Macromolecules, 2019, 52(6), 2531–2541 CrossRef CAS.
- C. Cui, C. Shao, L. Meng and J. Yang, High-strength, self-adhesive, and strain-sensitive chitosan/poly (acrylic acid) double-network nanocomposite hydrogels fabricated by salt-soaking strategy for flexible sensors, ACS Appl. Mater. Interfaces, 2019, 11(42), 39228–39237 CrossRef CAS PubMed.
- Y. Tang, S. Chen and X. Xiao, Tough, ion-conductive poly (vinyl alcohol)/polyelectrolyte hydrogels based on a double network for use as strain sensors, ACS Appl. Mater. Interfaces, 2023, 5(10), 7982–7993 CAS.
- W. Li, R. Feng, R. Wang, D. Li, W. Jiang, H. Liu, Z. Guo, M. J. Serpe and L. Hu, Polyelectrolyte-based physical adhesive hydrogels with excellent mechanical properties for biomedical applications, J. Mater. Chem. B, 2018, 6(29), 4799–4807 RSC.
- X. Yu, H. Zhang, Y. Wang, X. Fan, Z. Li, X. Zhang and T. Liu, Highly stretchable, ultra-soft, and fast self-healable conductive hydrogels based on polyaniline nanoparticles for sensitive flexible sensors, Adv. Funct. Mater., 2022, 32(33), 2204366 CrossRef CAS.
- H. Yuk, T. Zhang, G. A. Parada, X. Liu and X. Zhao, Skin-inspired hydrogel–elastomer hybrids with robust interfaces and functional microstructures, Nat. Commun., 2016, 7(1), 12028 CrossRef.
- J. W. Jeong, M. K. Kim, H. Cheng, W. H. Yeo, X. Huang, Y. Liu, Y. Zhang, Y. Huang and J. A. Rogers, Capacitive epidermal electronics for electrically safe, long-term electrophysiological measurements, Adv. Healthcare Mater., 2014, 3(5), 642–648 CrossRef CAS PubMed.
- Y. Chen, Y. Zhang, Z. Liang, Y. Cao, Z. Han and X. Feng, Flexible inorganic bioelectronics, npj Flexible Electron., 2020, 4(1), 2 CrossRef.
- Y. Liu, J. J. Norton, R. Qazi, Z. Zou, K. R. Ammann, H. Liu, L. Yan, P. L. Tran, K.-I. Jang and J. W. Lee, Epidermal mechano-acoustic sensing electronics for cardiovascular diagnostics and human-machine interfaces, Sci. Adv., 2016, 2(11), e1601185 CrossRef PubMed.
- C. Lim, Y. J. Hong, J. Jung, Y. Shin, S.-H. Sunwoo, S. Baik, O. K. Park, S. H. Choi, T. Hyeon and J. H. Kim, Tissue-like skin-device interface for wearable bioelectronics by using ultrasoft, mass-permeable, and low-impedance hydrogels, Sci. Adv., 2021, 7(19), eabd3716 CrossRef CAS PubMed.
- C. Xie, X. Wang, H. He, Y. Ding and X. Lu, Mussel-inspired hydrogels for self-adhesive bioelectronics, Adv. Funct. Mater., 2020, 30(25), 1909954 CrossRef CAS.
|
This journal is © The Royal Society of Chemistry 2025 |
Click here to see how this site uses Cookies. View our privacy policy here.