Facile engineering of CoS/rGO heterostructures on carbon cloth for efficient all-pH hydrogen evolution reaction and alkaline water electrolysis†
Received
20th September 2024
, Accepted 15th November 2024
First published on 15th November 2024
Abstract
Developing a cost-effective and commercially viable catalyst from non-noble metals that exhibits superior performance in both the hydrogen evolution reaction (HER) and oxygen evolution reaction (OER) presents a significant challenge due to the distinct electrocatalytic mechanisms involved in each process. Herein, we engineered a three-dimensional, self-supporting heterostructure on carbon cloth (CC) using a facile two-step electrodeposition, consisting of reduced graphene oxide (rGO) and cobalt sulfide (CoS), aiming at enhancing the efficiency of overall water electrolysis. The porous rGO network promoted the anchoring and vertical growth of CoS nanosheets, while the heterojunction between rGO and CoS enhanced the catalyst's stability remarkably. The CoS/rGO@CC catalyst exhibited extremely low overpotentials for both the HER (η10 = 76 mV) and OER (η10 = 290 mV), maintaining these stable overpotentials for more than 24 hours, matching the performance of leading electrocatalysts based on noble metals. Moreover, by utilizing CoS/rGO@CC as both the cathode and anode, we achieved overall water splitting with just 744 mV @ 10 mA cm−2. Theoretical calculations validated the synergistic effect of rGO and CoS nanosheets on enhancing HER and OER processes. Additionally, experimental data highlighted the CoS/rGO@CC catalyst's exceptional HER catalytic ability across varied pH levels, which provides a promising strategy to design low-cost and high-performance electrocatalysts for other energy-related applications.
1. Introduction
In recent years, the worsening energy crises and environmental pollution have escalated the demand for clean and renewable fuel resources.1,2 Hydrogen is regarded as a viable energy source to supplant fossil fuels and address environmental challenges.3 Nonetheless, the production, storage, and transportation of hydrogen face persistent challenges.4 Electrochemical water splitting, which includes the oxygen evolution reaction (OER) and hydrogen evolution reaction (HER), has emerged as a premier technology for hydrogen production. However, its efficiency is still limited by slow reaction kinetics and significant overpotentials across various energy systems.5–8 To improve catalytic efficiency, advanced water electrolysis catalysts largely depend on precious metals, which demonstrate remarkable catalytic performance, low overpotentials, and positive catalytic kinetics. Despite these advantages, their broad adoption is hindered by the high cost and limited durability.9 Furthermore, while noble metal catalysts show effective catalytic activity for specific reactions within water splitting, the creation of efficient bifunctional noble metal-based catalysts that enhance both the HER and OER is limited, restricting their extensive use in industrial water electrolysis.10,11 The OER, compared to the more advanced HER catalysts, presents greater complexity and challenge due to its four-step proton-coupled electron transfer mechanism and oxygen–oxygen bond formation, underscoring the necessity for developing high-performance bifunctional catalysts.12,13
Presently, non-precious transition metal compounds, such as Mo, W, Ni, Co, and Fe, are being extensively investigated as bifunctional electrocatalysts for water splitting.14–17 These include sulfides, phosphides, nitrides, carbides, oxides, and hydroxides, with transition metal sulfides (TMS) attracting significant attention due to their distinctive structural properties, ample active sites, adjustable electronic characteristics, and varied compositions.18–24 The appeal of cobalt-based chalcogenides stems from the abundant presence of cobalt in the Earth's crust. Due to its promising applications in catalysis, the cost-effective and widely accessible semiconductor material cobalt sulfide (CoS) has attracted substantial scientific interest.25 Nonetheless, its inherent poor conductivity as a semiconductor material diminishes charge transfer efficiency, consequently impeding its catalytic effectiveness.26 Moreover, the synthesis of these catalysts often relies on solution-based hydrothermal methods, which are labor-intensive and impractical for mass production.27–29 Attempts to employ electrodeposition for cobalt sulfides in the HER face challenges, frequently causing catalyst particle aggregation and weak substrate interactions, resulting in the reduction of catalytic efficiency.30
Electrocatalysts require the support of three-dimensional (3D) substrates that possess both a high specific surface area and conductivity, not merely to expose a greater number of active sites, but also to significantly expedite the electron transfer rate. In comparison to metal-based substrates, carbon-based substrates, such as carbon cloth (CC), carbon fiber paper (CFP), and carbon paper (CP), are highly esteemed due to their abundant availability, eco-friendliness, superior electrical conductivity, and exceptional stability under harsh acidic or alkaline conditions, which render them ideally suited to fulfill the rigorous requirements of industrial applications. Furthermore, the hydrophilic characteristics of carbon substrates not only play a crucial role in facilitating the desorption of gas bubbles and the penetration of electrolytes, but also in providing a distinct advantage for loading other low-dimensional carbon materials.31,32 Additionally, intricate synthesis processes involving metal-based substrates often result in considerable environmental degradation. The emission of metal ions from electrodes during these procedures may pose potential environmental hazards and increase costs in large-scale manufacturing endeavors.33,34 Considering the ease of manufacturing, coupled with the aforementioned advantageous properties, carbon-based substrates have emerged as sustainable and economically viable alternatives for the advancement of industrial electrocatalytic applications.35,36
In this study, we introduce a simple, eco-friendly two-step electrodeposition method to fabricate a high-performance bifunctional electrocatalyst (depicted in Scheme 1). This composite catalyst consists of vertically aligned CoS nanosheets and three-dimensional reduced graphene oxide (rGO) networks on carbon cloth (CC), which offer numerous catalytic sites and enhance the efficient diffusion of electrolytes, hydrogen, and oxygen bubbles. Furthermore, the synergy between CoS and rGO is validated through density functional theory (DFT) analysis, which indicates that its superior binding energies arise from the unique electronic configuration and coordination environment at Co-bonded C sites. This electrocatalyst showcases outstanding HER and OER performance across all pH levels, rivaling the most sophisticated water electrolysis catalysts. Notably, in an alkaline electrolyte, it displays exceptionally low overpotentials at 10 mA cm−2, with only 76.3 mV for the HER and 290.4 mV for the OER, significantly outperforming the CoS@CC and rGO@CC catalysts. Ultimately, a water electrolysis cell utilizing this bifunctional CoS/rGO@CC catalyst is developed, exhibiting a low operational voltage of 744 mV at 10 mA cm−2 and demonstrating satisfactory durability, making it a strong candidate for advanced and sustainable hydrogen energy applications.
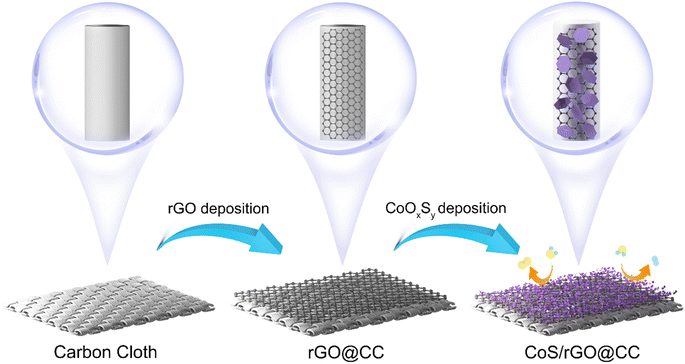 |
| Scheme 1 Schematic of the synthetic route for the CoS/rGO@CC electrocatalyst. | |
2. Experimental section
2.1 Chemicals
Carbon cloth (WOS1009 10 × 10 cm2) was purchased from Suzhou Yi Longsheng Energy Tech Co. Ltd (China). Anhydrous ethanol (CH3CH2OH, AR), thiourea (CH4N2S, AR), cobaltous nitrate hexahydrate (Co(NO3)2·6H2O, AR), potassium hydroxide (KOH, GR) and potassium hydride (KCl, GR) were obtained from Sinopharm Group Chemical Reagent Co., Ltd (China). In addition, graphene oxide (GO) dispersion (GO, 2 mg ml−1) was purchased from Nanjing XFNANO Materials Tech Co., Ltd. Ammonium tetrathiomolybdate ((NH4)2MoS4, 99.95%) was acquired from Alfa Aesar (China) Chemical Co., Ltd.
2.2 Treatment of carbon cloth
Before electrodeposition, the carbon cloth (CC) underwent pretreatment. Specifically, it was cut into small pieces (1 × 1 cm2) and sequentially cleaned in acetone, ethanol, and deionized water for 10 minutes each. Following the cleaning process, the carbon cloth was dried in a vacuum oven at 80 °C until fully dry. Finally, the prepared carbon cloth was stored in a sterile environment. Contact angle measurements were conducted on the carbon cloth before and after pretreatment. As shown in Fig. S1,† the hydrophilicity significantly increased after pretreatment.
2.3 Synthesis of the rGO@CC substrate
The synthesis of the substrate was intricately governed by the precise modulation of variables, including the concentration of the reduced graphene oxide (rGO) solution, the applied voltage, and the duration of deposition. Firstly, graphene oxide (GO) was prepared through a modified Hummers' method, tailored to confer diminished defect density and monolayer attributes. The resulting GO nanosheets manifested excellent dispersibility in aqueous media and exhibited remarkable stability, capable of maintaining their homogeneous state within the temperature range of 0 to 6 °C for a prolonged duration, while adeptly evading agglomeration or precipitation phenomena. Subsequently, employing the technique of amperometry under a steady potential of −1.2 V, a substrate comprising carbon cloth was immersed into a solution of GO and subjected to an electrochemical deposition protocol extending over a span of 1200 seconds. The concentration of rGO was optimally set at 2 mg ml−1, a choice that demonstrated superior performance when contrasted against concentrations of 3 mg ml−1, 2 mg ml−1, and 1 mg ml−1, respectively.
2.4 Synthesis of the CoS/rGO@CC electrocatalyst
The cobalt sulfide (CoS) component played a crucial role in this composite electrocatalyst. For electrodeposition, an electrolyte solution containing 5 mM Co(NO3)2 and 5 mM CH4N2S was carefully mixed to achieve a total volume of 1000 ml. The electrodeposition was carried out using cyclic voltammetry (CV), with a potential scan range from −1.2 V to −0.2 V. As shown in Fig. S2,† scanning rates of 5 mV s−1, 10 mV s−1 and 20 mV s−1 were used during electrodeposition, with 10 deposition cycles for effective CoS deposition. The CoS/rGO@CC electrocatalyst underwent successful synthesis, including a purification step of rinsing with deionized water and drying in a vacuum oven at 50 °C for 2 hours.
2.5 Characterization
Scanning electron microscope (SEM) analyses were performed using a Quanta 200 FEI (FEI Company, USA). The morphology, chemical composition, and elemental mapping were examined using a transmission electron microscope (TEM, JEM-2000EX) with an energy dispersive X-ray spectrometer (EDS). Raman spectra were acquired using an Alpha300 RA (WITEC Group, Germany). The crystal structure of the electrodeposits was characterized by X-ray diffraction (XRD) patterns obtained from an Ultima IV diffractometer (Rigaku Corporation, Japan). Surface composition and chemical states were analyzed via X-ray photoelectron spectroscopy (XPS, Thermo Scientific ESCALAB 250Xi). Contact angle measurements on the carbon cloth surface were conducted using a contact angle goniometer (SDC-200).
2.6 Electrochemical tests
Electrochemical analyses were performed using a CHI760E electrochemical analyzer (Shanghai Chenhua Instrument Co., Ltd). In this detailed study, carbon cloth served as the working electrode, and a carbon rod functioned as the counter electrode, with Ag/AgCl as the reference electrode. Measurements of the hydrogen evolution reaction (HER) were conducted in solutions containing 0.5 M H2SO4, 1.0 M KOH, and 1 M PBS, while experiments for the oxygen evolution reaction (OER) were performed in 1.0 M KOH. The extensive set of experiments included Linear Sweep Voltammetry (LSV), Tafel analysis, Cyclic Voltammetry (CV), Electrochemical Impedance Spectroscopy (EIS), and current–time (I–t) method analysis.
2.7 Theoretical simulations
The Vienna Ab initio Simulation Package (VASP)37,38 software was used to accomplish the density functional theory (DFT) calculations. The projector augmented wave (PAW) method39 with the Perdew–Burke–Ernzerhof (PBE) functional was selected and the plane-wave cutoff energy was set to 500 eV.40 Geometric structures were fully relaxed in cells with a fixed volume until the energy and total forces were converged to 10−3 eV and 0.01 eV Å−1, respectively. The Brillouin zone was sampled by the Γ-centered Monkhorst–Pack method with a 2 × 2 × 1 k-point grid. VASPKIT code41 was used for zero point energy and entropy analysis. The CoS/rGO model was constructed by embedding CoS clusters in the surface of rGO. The vacuum spacing was set to 20 Å along the surface normal to avoid the interaction between layers.
3. Results and discussion
Initially, the synthesized CoS/rGO@CC nanoarchitecture's three-dimensional, self-supported microstructure and morphology were examined using field-emission scanning electron microscopy (FE-SEM) and transmission electron microscopy (TEM). High-magnification SEM images (Fig. S3a†) of the carbon cloth (CC) reveal a dense, smooth carbon network without visible defects or particles. The reduced graphene oxide (rGO) uniformly coats the carbon cloth as pleated films, increasing the specific surface area (Fig. S3b†). After the electrodeposition of cobalt sulfide (CoS), SEM images (Fig. 1a and b) show the CoS/rGO@CC morphology with vertically aligned hexagonal CoS nanosheets covering the rGO network. High-resolution TEM analysis provided crystallographic details of the CoS/rGO@CC nanostructure, with CoS anchored onto the rGO nanosheets (Fig. 1c and d) and displaying hexagonal geometry (Fig. 1e). Clear lattice fringes in Fig. 1f indicate interplanar distances of 2.56 and 1.67 Å, corresponding to the (101) and (110) planes of CoS, respectively. The specific lattice spacing of 3.20 Å in Fig. 1g matches that of the (002) plane of graphene. The selected area electron diffraction (SAED) pattern (Fig. 1h) confirms the (100), (110), (102), and (101) CoS crystal planes, identified by XRD. High-angle annular dark-field scanning transmission electron microscopy (HAADF-STEM) and elemental mapping (Fig. 1i–l) show the CoS/rGO@CC composition mainly includes Co, O, S, and C, confirming CoS's successful anchoring onto the rGO network.
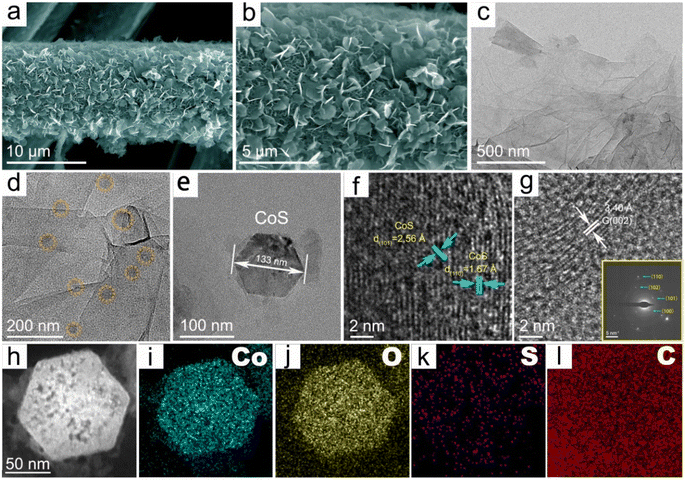 |
| Fig. 1 Morphological and microstructural analysis of the 3D CoS/rGO@CC electrocatalyst. Representative (a and b) FE-SEM images, (c–e) TEM images, and (f) HR-TEM image revealing the lattice fringes of CoS nanosheets. (g) HR-TEM image disclosing the lattice fringes of graphene nanosheets and the SAED pattern of CoS. (h) The HAADF-STEM image and the corresponding elemental mapping analysis, which provide evidence that the 3D hybrid nanoarchitecture is composed of (i) Co, (j) O, (k) S, and (l) C elements. | |
X-ray diffraction (XRD) and Raman analysis provided further insights into the CoS/rGO@CC nanoarchitecture's crystal and chemical structures. XRD patterns (Fig. 2a) show diffraction peaks at 32.57°, 38.05°, 43.75°, and 51.55°, corresponding to the hexagonal CoS's (100), (101), (102), and (110) planes (JCPDS card no. 65-3418). Peak shifts suggest increased lattice defects due to CoS and rGO integration. The broad peak near 25.2° indicates rGO's (002) plane. The Raman spectra, as shown in Fig. 2b, feature CoS peaks at 286 cm−1 (E12g), 375 cm−1 (F2g), and 483 cm−1 (A1g), which corroborate the presence of CoS nanolayers. Notably, the D-band and G-band, characteristic of graphene, are observed at 1346 cm−1 and 1592 cm−1, respectively. The intensity ratio of the D to G band (ID/IG) for the CoS/rGO@CC composite stands at 1.26, which is distinctly higher than the 1.03 ratio observed for rGO@CC, which indicates a higher level of lattice defects in the CoS/rGO@CC composite.42,43 XPS analysis (Fig. S4† and 2c–e) identified C, O, S, and Co as main elements, aligning with EDX data (Fig. S5†). In the high-resolution Co 2p spectrum shown in Fig. 2c, the deconvoluted peaks have been precisely identified, including the Co–S bond at 778.3 and 794 eV, Co3+ species at 780.8 and 796.4 eV, Co2+ species at 782.6 and 797.7 eV, and satellite peaks at 786.2 and 803.1 eV.44 Peaks appearing at 162.8, 165.2, and 168.4 eV within the S 2p fine structure spectrum are attributed to S 2p1/2, S 2p3/2, and Co–S–O groups, respectively. Further, the O 1s spectrum showcases prominent peaks at 531.25 eV and 532.16 eV, linked to Co–O and C–O configurations.45 Similarly, depicted in Fig. S4b,† the C 1s spectrum presents peaks at 284.7, 285.9, 286.1, 287.8, and 288.8 eV, each aligning with distinct functional groups, specifically C–N/C–S, C–C/C
C, C–O, C
O, and O–C
O.
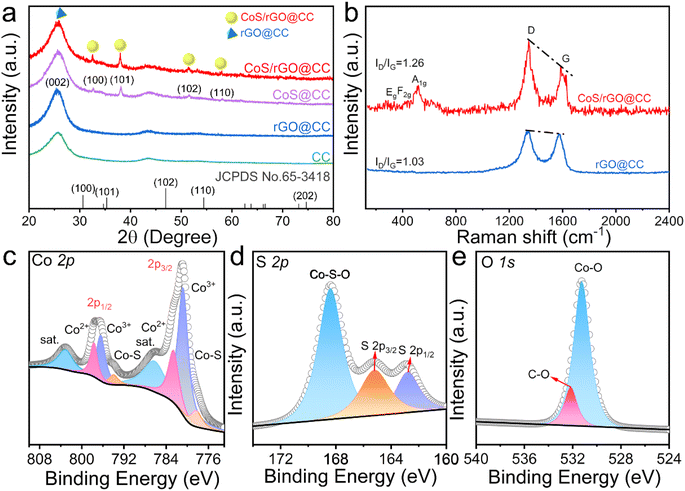 |
| Fig. 2 (a) The XRD patterns of CoS/rGO@CC, CoS@CC, rGO@CC, and pure CC. (b) Raman spectra of CoS/rGO@CC and rGO@CC; XPS analysis of the CoS/rGO@CC nanoarchitecture. High-resolution (c) Co 2p, (d) S 2p and (e) O 1s spectra disclosing the detailed chemical valences for these elements. | |
The electrochemical performance of the CoS/rGO@CC electrocatalyst for both the hydrogen evolution reaction (HER) and oxygen evolution reaction (OER) was evaluated in a 1.0 M KOH alkaline solution. Results indicate that hexagonal cobalt sulfide significantly enhances HER activity, as demonstrated by linear sweep voltammetry (LSV) for various electrocatalysts (carbon cloth, rGO@CC, CoS/rGO@CC, and 20% Pt/C) in 1.0 M KOH (Fig. 3a). Remarkably, the CoS/rGO@CC electrocatalyst achieved a current density of 10 mA cm−2 at an overpotential of 76 mV for the HER, outperforming CoS@CC (441 mV) and rGO@CC (573 mV), and closely matching 20% Pt/C (47 mV), underscoring its superior catalytic efficiency (Fig. 3b and Table S1†). This enhancement is attributed to the synergistic effects of electrodeposited reduced graphene oxide and cobalt sulfide. Moreover, the CoS/rGO@CC electrode's Tafel slope is 69 mV dec−1, indicating rapid HER kinetics compared to CoS@CC (194 mV dec−1), rGO@CC (237 mV dec−1), and 20% Pt/C (31.2 mV dec−1) (Fig. 3c). This performance is competitive with recent cobalt-based catalysts, positioning it at a high level (Fig. 3d and Table S2†).46–52 Cyclic voltammetry (CV) tests, performed at scan rates ranging from 20 to 120 mV s−1, quantified the electrodes' electrochemical active surface area (ECSA), illustrated in . The CoS/rGO@CC electrode's average double-layer capacitance (Cdl) was 8.84 mF cm−2, significantly surpassing that of CoS@CC (2.92 mF cm−2) and rGO@CC (1.62 mF cm−2) (Fig. 3f), indicating an abundance of effective active centers. Durability, vital for practical applications, was assessed through chronoamperometry and continuous LSV, showing the CoS/rGO@CC electrode maintains its initial current density over 24 hours and exhibits stable performance over 10
000 cycles without notable changes (Fig. 3g and h), confirming its exceptional cycling stability.
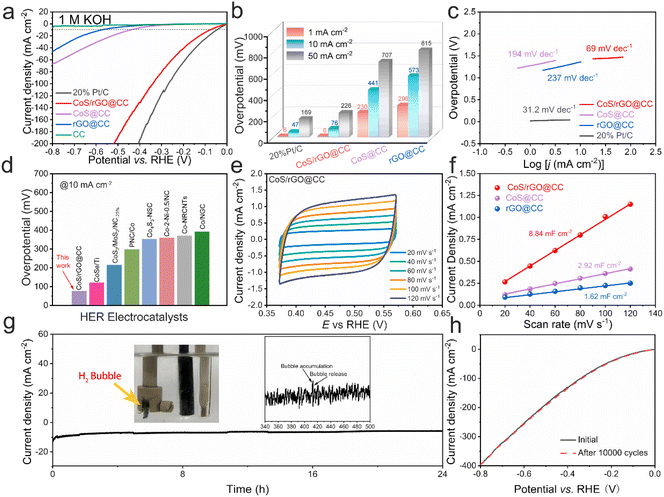 |
| Fig. 3 Electrocatalytic performance of the CoS/rGO@CC electrocatalyst for the HER. (a) Typical LSV polarization curves in 1 M KOH solution, (b) overpotentials at 1 mA cm−2, 10 mA cm−2, and 50 mA cm−2vs. RHE, and (c) corresponding Tafel plots of the CoS/rGO@CC, CoS@CC, rGO@CC, and 20% Pt/C electrodes in 1 M KOH solution, demonstrating that the CoS/rGO@CC electrode exhibits the highest HER activity. (d) Comparison of overpotentials at 10 mA cm−2 between the CoS/rGO@CC catalyst and recent state-of-the-art cobalt-based catalysts. (e) CV curves of the CoS/rGO@CC electrode recorded at various scan rates. (f) Capacitive currents of CoS/rGO@CC, CoS@CC, and rGO@CC electrodes as a function of scan rate. (g) The chronopotentiometric curve of the CoS/rGO@CC electrode over 24 hours. (h) Cycling performance of the CoS/rGO@CC electrode. | |
Additionally, we assessed the electrocatalytic activity of CoS/rGO@CC for the oxygen evolution reaction (OER) in a 1.0 M KOH solution. LSV curves (Fig. 4a) for carbon cloth, CoS@CC, rGO@CC, CoS/rGO@CC, and RuO2 on CC 20% Pt/C reveal that CoS/rGO@CC exhibits enhanced OER activity, demonstrating the significant role of CoS deposition in improving the catalyst's OER activity. At a current density of 10 mA cm−2, overpotentials for CoS@CC and rGO@CC were 525 mV and 605 mV, respectively, whereas CoS/rGO@CC showed an overpotential of 290 mV, closely approaching the 400 mV of RuO2 on CC (Fig. 4b and Table S3†). Compared to CoS@CC (254 mV dec−1), rGO@CC (332 mV dec−1), and RuO2 on CC (106.7 mV dec−1), Tafel slope analysis indicates rapid OER kinetics for CoS/rGO@CC (71 mV dec−1) (Fig. 4c), highlighting its dominant advantage in electron transport and water oxidation. A comparative analysis with existing cobalt-based OER electrocatalysts confirms CoS/rGO@CC's outstanding performance in alkaline electrolytes (Fig. 4d, e and Table S4†).53–60 Additionally, electrochemical impedance spectroscopy (EIS) results demonstrate a significant enhancement in charge transfer resistance. As shown in Fig. 4f, S6 and Table S5,† the CoS/rGO@CC electrode possesses the smallest Nyquist semicircle diameter, confirming the lowest charge transfer resistance compared to other reference samples. The EIS result clarifies that the CoS/rGO@CC electrode enables rapid charge and electron transport due to the large surface area of the novel vertically aligned three-dimensional structure, promoting contact with the electrolyte and facilitating the release of gas bubbles from the electrode surface. Apart from excellent electrocatalytic performance, durability is considered a crucial parameter for evaluating the developed catalyst. In long-term chronoamperometric measurements under a constant current (Fig. 4g), the CoS/rGO@CC electrode can sustain operation for 24 hours, indicating its outstanding mechanical strength and substantial mass transfer performance in an alkaline medium. Furthermore, continuous LSV analysis also reveals that the polarization curve of the CoS/rGO@CC electrode remains almost identical to the initial curve after 10
000 cycles, with only a minimal and imperceptible decrease in the OER current (Fig. 4h).
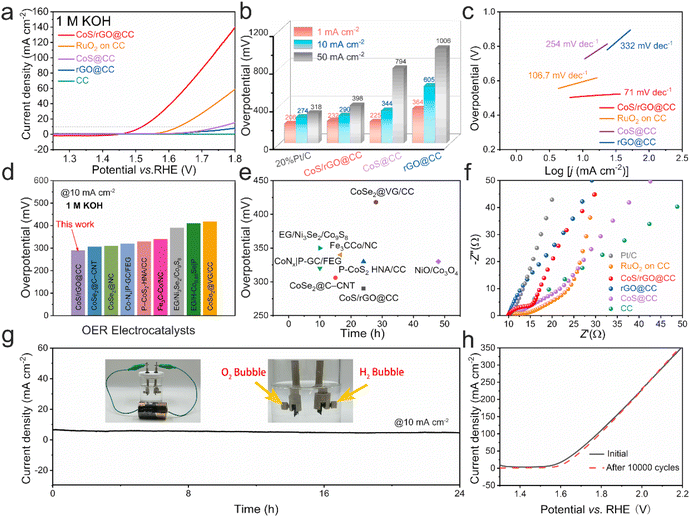 |
| Fig. 4 OER performance of the CoS/rGO@CC electrocatalyst. (a) Representative LSV curves, (b) histograms of required overpotentials, and (c) Tafel plots of the CoS/rGO@CC, CoS@CC, rGO@CC, and RuO2 on CC electrodes in 1 M KOH solution. (d) Comparison of overpotentials at 10 mA cm−2 between CoS/rGO@CC and previously reported cobalt-based catalysts. (e) Comparative analysis of oxygen evolution performance and stability of the CoS/rGO@CC catalyst and state-of-the-art cobalt-based catalysts in 1 M KOH solution. (f) EIS Nyquist plots AC impedance spectra of the CoS/rGO@CC, rGO@CC, and RuO2 on CC electrodes. (g) The chronopotentiometric curves of the CoS/rGO@CC catalyst at 10 mA cm−2. (h) Cycling performance of the CoS/rGO@CC electrode. | |
Inspired by the excellent HER and OER activity of CoS/rGO@CC, we explored the capability of this material as a bifunctional catalyst for overall water splitting in a 1.0 M KOH solution. A two-electrode system was constructed, where both the anode and cathode were made of CoS/rGO@CC. As shown in Fig. 5a and b, in an alkaline environment at room temperature, a current density of 10 mA cm−2 was achieved with just 744 mV, outperforming the setups where CoS/rGO@CC served solely as the anode catalyst (CoS/rGO@CC‖20% Pt/C) or solely as the cathode catalyst (CoS/rGO@CC‖RuO2 on CC). This performance surpasses that of most advanced catalysts previously reported (Fig. 5c and Table S6†).37,61–66 Moreover, the stability of the CoS/rGO@CC‖CoS/rGO@CC cell was tested, and the I–t curve clearly demonstrated its excellent durability after 14 hours (Fig. 5d). These findings further affirm that the developed CoS/rGO@CC catalyst is a promising candidate for the development of efficient and cost-effective water-splitting electrolyzers as an attractive bifunctional electrocatalyst.
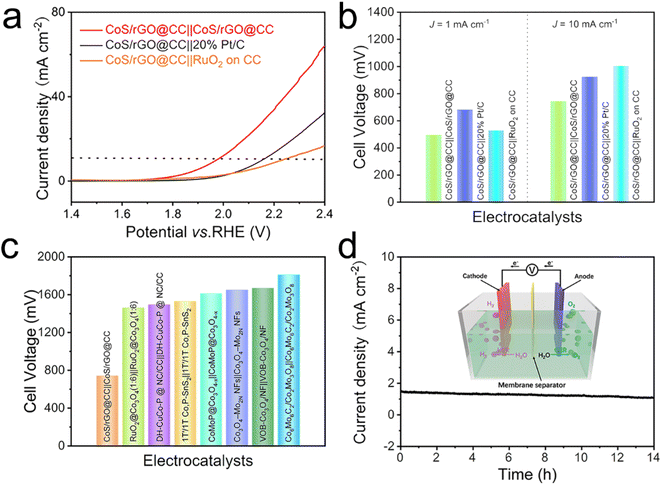 |
| Fig. 5 Overall water splitting performance of the CoS/rGO@CC electrocatalyst in a two-electrode system. (a) Representative LSV curves and (b) histograms of required overpotentials of the CoS/rGO@CC‖CoS/rGO@CC, CoS/rGO@CC‖20% Pt/C and CoS/rGO@CC‖RuO2 on CC electrocatalysts in 1 M KOH solution. (c) Comparison of overpotentials at 10 mA cm−2 between CoS/rGO@CC and previously reported cobalt-based catalysts. (d) The chronopotentiometric curves of the CoS/rGO@CC catalyst at 10 mA cm−2. | |
The density functional theory (DFT) calculations were further performed to understand the synergistic effect of CoS and rGO on the performance of the HER and OER. The energies of *H adsorbed on different atoms were calculated to figure out the active sites. Fig. 6a shows the structure of the possible stable adsorption configuration of the HER, and Fig. 6b shows the structure of the stable adsorption site of OER intermediates (*O, *OH, *OOH) on the substrate. The free energies of the HER and OER are shown in Fig. 6c–e, respectively, showing that the combination of CoS and rGO could effectively exhibit properties conducive to both HER and OER activities.
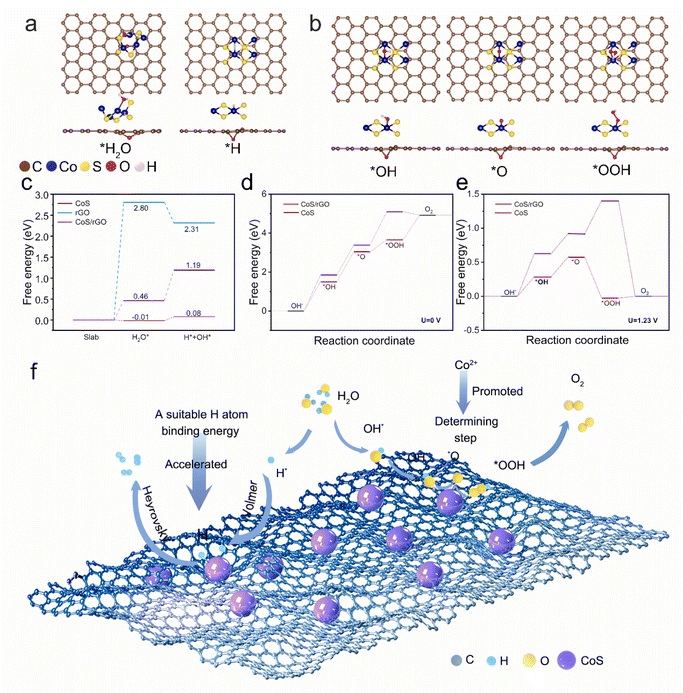 |
| Fig. 6 DFT calculations for explaining the mechanisms of the HER and OER. (a) HER process in the combined CoS/rGO model. (b) OER process of the CoS/rGO combined model. (c) Free energy paths of the HER and (d and e) OER processes. U = 0 V vs. RHE, U = 1.23 V vs. RHE. (f) Schematic illustration of the HER and OER processes on the CoS/rGO@CC catalyst. | |
For the HER process, active sites of pure CoS and rGO were first studied (Fig. S7–S9†). The potential barrier of Co sites was lower than that of S sites on the CoS surface. Therefore, Co atoms prefer to act as the active sites of CoS. Different active sites also exist in rGO due to C atoms being under an unequal chemical environment which can be defined by the different distances between C and O atoms. As shown in Fig. S9,† C1, C2 and C3 are defined based on their positions. As the nearest site from the O atom, the C1 atom has the lowest Gibbs free energy (ΔG) which indicates the oxidation–reduction of graphene is beneficial for HER performance. But the lowest ΔG of rGO is still significantly higher than those of CoS and CoS/rGO, which indicates that rGO itself does not act as an active site for the HER, but regulates the active sites in CoS to reduce the potential barrier indirectly. Fig. S10† gives the final design idea of DFT computational modeling, and the HER structure of the CoS/rGO combined model is shown in Fig. S11.† Fig. S12–S14† show the water ion adsorption configurations of pure rGO, pure CoS, and CoS/rGO during the hydrogen evolution reaction (HER) under alkaline conditions. Compared with those adsorbed on the pure CoS substrate, all adsorbates have lower ΔG on CoS/rGO. The negative ΔG value means that water tends to bind with CoS/rGO. The strategy of incorporating graphene results in a significant decrease in the potential barrier of the HER (from 0.73 eV to about 0.1 eV), which confirms the synergistic effect of CoS and rGO on boosting the HER performance (Fig. 6c).
Regarding the OER process, there's no stable adsorption configuration of each reaction step based on the pure rGO substrate and CoS with S atoms as the active sites. Therefore, Co atoms act as the active sites of both the HER and OER. Fig. 6b demonstrates the OER process of the CoS/rGO combined model. As shown in Fig. 6d and e, the calculated potential barrier values for the OER of CoS/rGO are 1.52 eV and 0.287 eV, respectively, under 0 V and 1.23 V vs. RHE. These values are notably lower than the corresponding potential barriers of 1.85 eV and 0.62 eV observed for pure CoS under the same conditions. This enhancement in activity is attributed to the synergistic interaction between CoS and rGO, which is facilitated by a reduction in the adsorption energy at each step of the reaction.
The recombination of these Had atoms then occurs through two possible pathways, either the Volmer or the Heyrovsky mechanisms, culminating in the generation of H2 gas. In the case of the OER process under alkaline conditions, the active cobalt species within the CoS/rGO@CC catalyst undergo partial oxidation to form the CoOOH species, which in turn facilitates the formation of CoOOH/CoS heterostructures. These heterostructures act as active sites for oxidizing the adsorbed OH− species into molecular oxygen. Upon the application of higher potentials, the CoOOH/CoS intermediate phase undergoes further oxidation to transition into CoO2/CoS, serving as an adsorbed secondary transitional phase active species crucial for efficient OER activity. Consequently, the establishment of a thin layer of CoO2/CoOH shells over CoS is identified as a key active site for OER activity.
Fig. 6f illustrates the catalytic mechanism of the CoS/rGO@CC catalyst in the HER and OER processes. The HER process in an alkaline solution involves the adsorption of H2O molecules and the decomposition of adsorbed H2O into hydroxyl ions (OH−) and adsorbed hydrogen atoms (Had), followed by the recombination of Had through two pathways (Volmer or Heyrovsky mechanisms) to generate H2. The unique three-dimensional structure of CoS/rGO@CC, characterized by its vast surface area, plays a pivotal role in enhancing its catalytic performance for the HER. In the OER process, when operating under alkaline conditions, the active Co species in the CoS/rGO@CC catalyst are partially oxidized to form active CoOOH species, ultimately forming CoOOH/CoS heterostructures as active sites. These heterostructures serve as the roots to oxidize the adsorbed OH− species into molecular oxygen. At higher applied potentials, the CoOOH/CoS intermediate phase formed is further oxidized to create CoO2/CoS, an adsorbed secondary transitional phase active species for effective OER activity.67 Therefore, the formation of a thin layer of CoO2/CoOH shells on CoS is recognized as the constructive active site for OER activity.68 Finally, additional oxidation includes the desorption of molecular oxygen as bubbles. This mechanism underscores the CoS/rGO@CC catalyst's ability to offer superior conductivity, thanks to the in situ growth of CoS on rGO@CC, which significantly enhances electron transport throughout the catalytic process. The exemplary bifunctional catalytic activity observed in CoS/rGO@CC for overall water splitting can be attributed to the synergistic effects harnessed between CoS and rGO, showcasing its potential in energy conversion applications.
Furthermore, to reveal the changes in chemical valence states of Co, O, and S during the OER process and to explore the formation of possible reactive species, we performed XPS and SEM analysis on the CoS/rGO@CC after testing. The SEM images and EDX spectra obtained after the I–t testing are shown in Fig. S15 and S16,† indicating that the CoS and rGO nanosheets remain firmly attached to the carbon cloth. The high-resolution Co 2p spectrum indicated a significant reduction or disappearance of the Co–S bond (Co0 species) peaks and a shift to lower binding energies, demonstrating partial oxidation of the CoS/rGO@CC during the OER. The more intense peaks at 795.4 eV and 780.3 eV can be classified as Co3+ in the form of Co3+ 2p3/2 and Co3+ 2p1/2 in CoOOH, substantiating the transformation of active Co into CoOOH species during OER catalysis. These findings confirm that active Co undergoes a change in its chemical valence state to form CoOOH, eventually leading to the formation of CoO2 as part of the OER process (Fig. S17†).
Currently, water electrolysis is conducted through three primary methods: microbial electrolysis cells in neutral environments, proton exchange membrane electrolyzers under acidic conditions, and alkaline electrolysis cells in alkaline settings. Consequently, there is a heightened focus on developing a cost-effective and highly efficient electrocatalyst capable of facilitating the hydrogen evolution reaction across a wide pH range. Building on our analysis of the CoS/rGO@CC catalyst's catalytic performance in an alkaline electrolyte for overall water splitting, we have extended our evaluation to its hydrogen evolution efficiency in both acidic (as illustrated in Fig. 7a–f; detailed in Tables S7 and S8†) and neutral electrolytes (shown in Fig. 7g–l; described in Tables S9 and S10†). In these experiments, the CoS/rGO@CC catalyst demonstrated superior catalytic performance and stability under both acidic and neutral conditions, with low overpotentials of 41 and 315 mV at 10 mA cm−2 respectively, and long stability periods of 24 hours and 21 hours, owing to its innovative heterogeneous structure and unique vertically aligned structural features.68–81
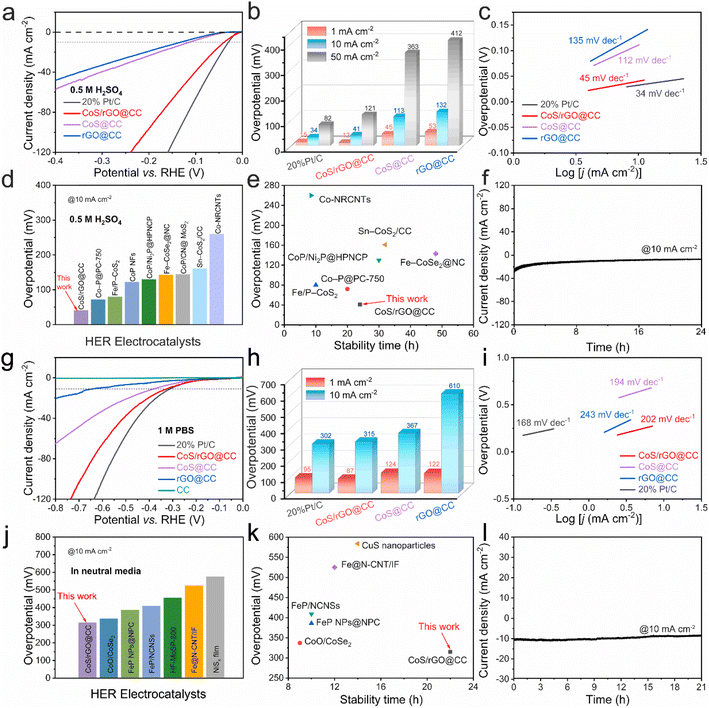 |
| Fig. 7 Hydrogen evolution performance of CoS/rGO@CC nanostructures in acidic and neutral solutions. (a) Typical LSV curves in 0.5 M H2SO4 solution, (b) histograms of required overpotentials, and (c) typical Tafel plots, comparing CoS/rGO@CC with CoS@CC, rGO@CC, and Pt/C electrodes in 0.5 M H2SO4 solution. (d) Comparison of overpotentials at 10 mA cm−2 between the CoS/rGO@CC catalyst and recent state-of-the-art cobalt-based catalysts. (e) Comparative analysis of hydrogen evolution performance and stability between the CoS/rGO@CC catalyst and recent state-of-the-art cobalt-based catalysts in 0.5 M H2SO4 solution. (f) The chronoamperometric curve of the CoS/rGO@CC electrode over 24 hours. (g) Typical Linear Sweep Voltammetry (LSV) curves in a 1 M PBS solution. (h) Histograms illustrating the requisite overpotentials. (i) Typical Tafel plots providing a comparative perspective between CoS/rGO@CC, CoS@CC, rGO@CC, and Pt/C electrodes in a 1 M PBS solution. (j) Comparative analysis of overpotentials at 10 mA cm−2, highlighting the distinction between the CoS/rGO@CC catalyst and recent advancements in neutral medium hydrogen evolution catalysts. (k) Comparative assessment of hydrogen evolution performance and stability, juxtaposing the CoS/rGO@CC catalyst against the latest developments in neutral medium hydrogen evolution catalysts. (l) The chronoamperometric curve depicting the performance of the CoS/rGO@CC electrode over a 21 h duration in a 1 M PBS environment. | |
4. Conclusions
In conclusion, we have successfully developed a three-dimensional CoS/rGO@CC electrocatalyst for overall water electrolysis through a facile two-step electrodeposition technique. CoS/rGO@CC demonstrates excellent bifunctional electrocatalytic activity and stability in alkaline solutions, achieving an overpotential of just 76 mV for the hydrogen evolution reaction (HER) and 290 mV for the oxygen evolution reaction (OER). The catalyst maintains a stable overpotential for 24 hours at a current density of 10 mA cm−2. Furthermore, by using CoS/rGO@CC as both the cathode and anode, overall water splitting can be achieved with just 744 mV @ 10 mA cm−2. The improved catalytic performance and cycling stability of the CoS/rGO@CC electrocatalyst result from the synergistic effect between CoS and rGO heterojunctions. Additionally, the CoS/rGO@CC catalyst also demonstrates considerable HER catalytic ability across varied pH levels, with the potentials at 10 mA cm−2 being low at 41 mV and 315 mV in 0.5 M H2SO4 and 1 M PBS, respectively, which are comparable to that of commercial Pt/C. Due to its unique catalytic activity, stability, and straightforward production technology, this catalyst emerges as a promising noble-metal-free alternative for overall water electrolysis and other energy-related applications.
Data availability
All relevant data are provided within the manuscript and its ESI.†
Conflicts of interest
There are no conflicts of interest to declare.
Acknowledgements
This work was supported by the National Natural Science Foundation of China (grant no. 52305596 and 52375564), Carbon Peak and Carbon Neutrality Technology Innovation Special Project of Jiangsu Province (grant no. BE2023853), Natural Science Foundation of Jiangsu Province (grant no. BK20231424), and Open Foundation of Jiangsu Key Laboratory of Precision and Microfabrication Technology (grant no. JSKL2223K04).
References
- N. Abas, A. Kalair and N. Khan, Review of fossil fuels and future energy technologies, Futures, 2015, 69, 31–49 CrossRef.
- Z. P. Cano, D. Banham and S. Ye,
et al., Batteries and fuel cells for emerging electric vehicle markets, Nat. Energy, 2018, 3, 279–289 CrossRef.
- H. Huang, M. Yan and C. Yang,
et al., Graphene nanoarchitectonics: recent advances in graphene-based electrocatalysts for hydrogen evolution reaction, Adv. Mater., 2019, 31, e1903415 CrossRef.
- B. Shen, Y. Feng and Y. Wang,
et al., Holey MXene nanosheets intimately coupled with ultrathin Ni-Fe layered double hydroxides for boosted hydrogen and oxygen evolution reactions, Carbon, 2023, 212, 118141 CrossRef CAS.
- Q. Sun, Y. Tong and P. Chen,
et al., Universal strategy of bimetal heterostructures as superior bifunctional catalysts for electrochemical water splitting, ACS Sustain. Chem. Eng., 2021, 9, 4206–4212 CrossRef CAS.
- J. Y. Wang, T. Ouyang and N. Li,
et al., S, N co-doped carbon nanotube-encapsulated core–shelled CoS2@Co nanoparticles: efficient and stable bifunctional catalysts for overall water splitting, Sci. Bull., 2018, 63, 1130–1140 CrossRef CAS.
- R. Bose, V. R. Jothi and K. Karuppasamy,
et al., High performance multicomponent bifunctional catalysts for overall water splitting, J. Mater. Chem. A, 2020, 8, 13795–13805 RSC.
- T. Kou, M. Chen and F. Wu,
et al., Carbon doping switching on the hydrogen adsorption activity of NiO for hydrogen evolution reaction, Nat. Commun., 2020, 11, 590 CrossRef CAS.
- Y. Zhou, Q. Wang and X. Tian,
et al., Electron-enriched Pt induced by CoSe2 promoted bifunctional catalytic ability for low carbon alcohol fuel electro-reforming of hydrogen evolution, J. Energy Chem., 2022, 75, 46–54 CrossRef CAS.
- W. Yu, Z. Chen and Y. Fu,
et al., Superb all-pH hydrogen evolution performances powered by ultralow Pt-decorated hierarchical Ni–Mo porous microcolumns, Adv. Funct. Mater., 2022, 33(4), 2210855 CrossRef.
- Q. He, Y. Zhou and H. Shou,
et al., Synergic reaction kinetics over adjacent ruthenium sites for superb hydrogen generation in alkaline media, Adv. Mater., 2022, 34, e2110604 CrossRef.
- S. Jin, Are metal chalcogenides, nitrides, and phosphides oxygen evolution catalysts or bifunctional catalysts?, ACS Energy Lett., 2017, 2, 1937–1938 CrossRef CAS.
- R. Anand, A. S. Nissimagoudar and M. Umer,
et al., Late transition metal doped MXenes showing superb bifunctional electrocatalytic activities for water splitting via distinctive mechanistic pathways, Adv. Energy Mater., 2021, 11(48), 2102388 CrossRef CAS.
- J. Xie, F. Wang, Y. Zhou and Y. Dong,
et al., Internal polarization field induced hydroxyl spillover effect for industrial water splitting electrolyzers, Nano-Micro Lett., 2023, 16, 39 CrossRef PubMed.
- Y. N. Zhou, W. L. Yu and H. J. Liu,
et al., Self-integration exactly constructing oxygen-modified MoNi alloys for efficient hydrogen evolution, EcoEnergy, 2023, 1, 425–436 CrossRef.
- Y. N. Zhou, Y. W. Dong and Y. Wu,
et al., Nitrate induced precise atom substitution and vacancies for overall water splitting, Chem. Eng. J., 2023, 463, 142380 CrossRef CAS.
- H. J. Liu, S. Zhang and Y. M. Chai,
et al., Ligand modulation of active sites to promote cobalt-doped 1T-MoS2 electrocatalytic hydrogen evolution in alkaline media, Angew. Chem., Int. Ed., 2023, 62, e202313845 CrossRef CAS.
- M. Wang, L. Zhang and Y. He,
et al., Recent advances in transition-metal-sulfide-based bifunctional electrocatalysts for overall water splitting, J. Mater. Chem. A, 2021, 9, 5320–5363 RSC.
- J. Joo, T. Kim and J. Lee,
et al., Morphology-controlled metal sulfides and phosphides for electrochemical water splitting, Adv. Mater., 2019, 31, e1806682 CrossRef PubMed.
- C. Hu, L. Zhang and J. Gong, Recent progress made in the mechanism comprehension and design of electrocatalysts for alkaline water splitting, Energy Environ. Sci., 2019, 12, 2620–2645 RSC.
- S. Anantharaj, S. R. Ede and K. Sakthikumar,
et al., Recent trends and perspectives in electrochemical water splitting with an emphasis on sulfide, selenide, and phosphide catalysts of Fe, Co, and Ni: a review, ACS Catal., 2016, 6, 8069–8097 CrossRef CAS.
- F. Yu, L. Yu and I. K. Mishra,
et al., Recent developments in earth-abundant and non-noble electrocatalysts for water electrolysis, Mater. Today Phys., 2018, 7, 121–138 CrossRef.
- J. Wang, X. Yue and Y. Yang,
et al., Earth-abundant transition-metal-based bifunctional catalysts for overall electrochemical water splitting: a review, J. Alloys Compd., 2020, 819, 153346 CrossRef CAS.
- Z. Kang, H. Guo and J. Wu,
et al., Engineering an earth-abundant element-based bifunctional electrocatalyst for highly efficient and durable overall water splitting, Adv. Funct. Mater., 2019, 29(9), 1807031 CrossRef.
- S. Gupta, R. Fernandes and R. Patel,
et al., A review of cobalt-based catalysts for sustainable energy and environmental applications, Appl. Catal., A, 2023, 661, 119254 CrossRef CAS.
- Y. Chen, S. Xu and S. Zhu,
et al., Millisecond synthesis of CoS nanoparticles for highly efficient overall water splitting, Nano Res., 2019, 12, 2259–2267 CrossRef CAS.
- K. V. G. Raghavendra, K. M. Rao and N. T. U. Kumar, Hydrothermal synthesis of CuS/CoS nano composite as an efficient electrode for the supercapattery applications, J. Energy Storage, 2021, 40, 102749 CrossRef.
- F. Huang, R. Meng and Y. Sui,
et al., One-step hydrothermal synthesis of a CoS2@MoS2 nanocomposite for high-performance supercapacitors, J. Alloys Compd., 2018, 742, 844–851 CrossRef CAS.
- S. Surendran, S. Shanmugapriya and H. Ramasamy,
et al., Hydrothermal deposition of CoS nanostructures and its multifunctional applications in supercapattery and water electrolyzer, Appl. Surf. Sci., 2019, 494, 916–928 CrossRef CAS.
- B. Liu, S. Qu and Y. Kou,
et al., In Situ Electrodeposition of Cobalt Sulfide Nanosheet Arrays on Carbon Cloth as a Highly Efficient Bifunctional Electrocatalyst for Oxygen Evolution and Reduction Reactions, ACS Appl. Mater. Interfaces, 2018, 10, 30433–30440 CrossRef CAS.
- X. Shang, J. Q. Chi and S. S. Lu,
et al., Novel CoxSy/WS2 nanosheets supported on carbon cloth as efficient electrocatalyst for hydrogen evolution reaction, Int. J. Hydrogen Energy, 2017, 42, 4165–4173 CrossRef CAS.
- T. Y. Ma, S. Dai and S. Z. Qiao, Self-supported electrocatalysts for advanced energy conversion processes, Mater. Today, 2016, 19, 265–273 CrossRef CAS.
- J. Han, N. Deng and H. Chi,
et al., Recent advances of carbon fiber-based self-supported electrocatalysts in oxygen electrocatalysis, J. Energy Chem., 2024, 98, 334–363 CrossRef CAS.
- F. M. Yap, J. Y. Loh and S. F. Ng,
et al., Self-supported earth-abundant carbon-based substrates in electrocatalysis landscape: unleashing the potentials toward paving the way for water splitting and alcohol oxidation, Adv. Energy Mater., 2023, 14, 2303614 CrossRef.
- Q. Xiang, J. Wang and Q. Miao,
et al., Recent progress in self-supported nanoarrays with diverse substrates for water splitting and beyond, Mater. Today Nano, 2021, 15, 100120 CrossRef CAS.
- H. Shi, G. Wen and Y. Nie,
et al., Flexible 3D carbon cloth as a high-performing electrode for energy storage and conversion, Nanoscale, 2020, 12, 5261–5285 RSC.
- A. A. Gravely, A. Cutting and S. Nugent,
et al., Validity of PTSD diagnoses in VA administrative data: comparison of VA administrative PTSD diagnoses to self-reported PTSD checklist scores, J. Rehabil. Res. Dev., 2011, 48, 21–30 CrossRef PubMed.
- G. Kresse and J. Furthmüller, Efficient iterative schemes for ab initio total-energy calculations using a plane-wave basis set, Phys. Rev. B: Condens. Matter Mater. Phys., 1996, 54(16), 11169 CrossRef CAS PubMed.
- P. E. Blochl, Projector augmented-wave method, Phys. Rev. B: Condens. Matter Mater. Phys., 1994, 50, 17953–17979 CrossRef.
- J. P. Perdew, K. Burke and M. Ernzerhof, Generalized gradient approximation made simple, Phys. Rev. Lett., 1996, 77(18), 3865 CrossRef CAS.
- V. Wang, N. Xu and J. C. Liu,
et al., VASPKIT: a user-friendly interface facilitating high-throughput computing and analysis using VASP code, Comput. Phys. Commun., 2021, 267, 108033 CrossRef CAS.
- X. Wang, J. Zhang and P. Wang,
et al., Terbium-induced cobalt valence-band narrowing boosts electrocatalytic oxygen reduction, Energy Environ. Sci., 2023, 16, 5500–5512 RSC.
- Z. Li, X. Wu and X. Jiang,
et al., Surface carbon layer controllable Ni3Fe particles confined in hierarchical N-doped carbon framework boosting oxygen evolution reaction, Adv. Powder. Mater., 2022, 1, 100020 CrossRef.
- Y. Wang, X. Wu and X. Jiang,
et al., Citrulline-induced mesoporous CoS/CoO heterojunction nanorods triggering high-efficiency oxygen electrocatalysis in solid-state Zn–air batteries, Chem. Eng. J., 2022, 434, 134744 CrossRef CAS.
- R. Y. Fan, J. Y. Xie and Y. Ma,
et al., Structure optimization and electronic modulation of sulfur-incorporated cobalt nanocages for enhanced oxygen evolution, Int. J. Hydrogen Energy, 2021, 46, 28537–28544 CrossRef CAS.
- T. Liu, Q. Liu and A. M. Asiri,
et al., An amorphous CoSe film behaves as an active and stable full water-splitting electrocatalyst under strongly alkaline conditions, Chem. Commun., 2015, 51, 16683–16686 RSC.
- K. Ji, K. Matras-Postolek and R. Shi,
et al., MoS2/CoS2 heterostructures embedded in N-doped carbon nanosheets towards enhanced hydrogen evolution reaction, J. Alloys Compd., 2022, 891, 161962 CrossRef CAS.
- X. Li, Z. Niu and J. Jiang,
et al., Cobalt nanoparticles embedded in porous N-rich carbon as an efficient bifunctional electrocatalyst for water splitting, J. Mater. Chem. A, 2016, 4, 3204–3209 RSC.
- Y. Liu, X. Luo and C. Zhou,
et al., A modulated electronic state strategy designed to integrate active HER and OER components as hybrid heterostructures for efficient overall water splitting, Appl. Catal., B, 2020, 260, 118197 CrossRef CAS.
- X. Shen, W. Tan and Z. Wei,
et al., Co-modified Ni/N-doped carbon as electrocatalysts for HER and OER, J. Mater. Sci.: Mater. Electron., 2021, 32, 22974–22983 CrossRef CAS.
- X. Zou, X. Huang and A. Goswami,
et al., Cobalt-embedded nitrogen-rich carbon nanotubes efficiently catalyze hydrogen evolution reaction at all pH values, Angew. Chem., Int. Ed., 2014, 53, 4372–4376 CrossRef CAS PubMed.
- J. Li, Y. Kang and D. Liu,
et al., Nitrogen-doped graphitic carbon-supported ultrafine Co nanoparticles as an efficient multifunctional electrocatalyst for HER and rechargeable Zn–air batteries, ACS Appl. Mater. Interfaces, 2020, 12, 5717–5729 CrossRef CAS.
- M. Yuan, M. Wang and P. Lu,
et al., Tuning carbon nanotube-grafted core–shell-structured cobalt selenide@carbon hybrids for efficient oxygen evolution reaction, J. Colloid Interface Sci., 2019, 533, 503–512 CrossRef CAS PubMed.
- J. Lu, S. Wang and C. Ding,
et al., Metal organic frameworks derived CoSe2@N-doped-carbon-nanorods as highly efficient electrocatalysts for oxygen evolution reaction, J. Alloys Compd., 2019, 778, 134–140 CrossRef CAS.
- Y. Hou, M. Qiu and T. Zhang,
et al., Efficient electrochemical and photoelectrochemical water splitting by a 3D nanostructured carbon supported on flexible exfoliated graphene foil, Adv. Mater., 2017, 29, 1604480 CrossRef.
- Y. Li, Z. Mao and Q. Wang,
et al., Hollow nanosheet array of phosphorus-anion-decorated cobalt disulfide as an efficient electrocatalyst for overall water splitting, Chem. Eng. J., 2020, 390, 124556 CrossRef CAS.
- Y. Hou, M. Qiu and G. Nam,
et al., Integrated hierarchical cobalt sulfide/nickel selenide hybrid nanosheets as an efficient three-dimensional electrode for electrochemical and photoelectrochemical water splitting, Nano Lett., 2017, 17, 4202–4209 CrossRef CAS.
- Y. Hou, M. Qiu and T. Zhang,
et al., Ternary porous cobalt phosphoselenide nanosheets: an efficient electrocatalyst for electrocatalytic and photoelectrochemical water splitting, Adv. Mater., 2017, 29(35), 1701589 CrossRef.
- Z. Xia, H. Sun and X. He,
et al., In situ construction of CoSe2@vertical-oriented graphene arrays as self-supporting electrodes for sodium-ion capacitors and electrocatalytic oxygen evolution, Nano Energy, 2019, 60, 385–393 CrossRef CAS.
- M. Tahir, L. Pan and R. Zhang,
et al., High-valence-state NiO/Co3O4 nanoparticles on nitrogen-doped carbon for oxygen evolution at low overpotential, ACS Energy Lett., 2017, 2, 2177–2182 CrossRef CAS.
- Y. Jiang, H. Liu and Y. Jiang,
et al., Adjustable heterointerface-vacancy enhancement effect in RuO2@Co3O4 electrocatalysts for efficient overall water splitting, Appl. Catal., B, 2023, 324, 122294 CrossRef CAS.
- M. Singh, T. T. Nguyen and A. P. Muthu,
et al., Metallic metastable hybrid 1T′/1T phase triggered Co, P-SnS2 nanosheets for high efficiency trifunctional electrocatalyst, Small, 2023, 19, e2206726 CrossRef.
- Y. Hao, G. Du and Y. Fan,
et al., Mo/P dual-doped Co/oxygen-deficient Co3O4 core–shell nanorods supported on Ni foam for electrochemical overall water splitting, ACS Appl. Mater. Interfaces, 2021, 13, 55263–55271 CrossRef CAS.
- T. Wang, P. Wang and W. Zang,
et al., Nanoframes of Co3O4–Mo2N heterointerfaces enable high-performance bifunctionality toward both electrocatalytic HER and OER, Adv. Funct. Mater., 2021, 32(7), 2107382 CrossRef.
- H. Yuan, S. Wang and Z. Ma,
et al., Oxygen vacancies engineered self-supported B doped Co3O4 nanowires as an efficient multifunctional catalyst for electrochemical water splitting and hydrolysis of sodium borohydride, Chem. Eng. J., 2021, 404, 126474 CrossRef CAS.
- R. Liu, M. Anjass and S. Greiner,
et al., Bottom-up design of bimetallic cobalt-molybdenum carbides/oxides for overall water splitting, Chem.–Eur. J., 2020, 26, 4157–4164 CrossRef CAS.
- J. Lin, H. Wang and X. Zheng,
et al., Controllable synthesis of core-branch Ni3S2/Co9S8 directly on nickel foam as an efficient bifunctional electrocatalyst for overall water splitting, J. Power Sources, 2018, 401, 329–335 CrossRef CAS.
- P. Chen, K. Xu and Z. Fang,
et al., Metallic Co4N porous nanowire arrays activated by surface oxidation as electrocatalysts for the oxygen evolution reaction, Angew. Chem., Int. Ed., 2015, 54, 14710–14714 CrossRef CAS PubMed.
- J. Wu, D. Wang and S. Wan,
et al., An efficient cobalt phosphide electrocatalyst derived from cobalt phosphonate complex for all-pH hydrogen evolution reaction and overall water splitting in alkaline solution, Small, 2020, 16, 1900550 CrossRef CAS.
- Y. Y. Zhang, X. Zhang and Z. Y. Wu,
et al., Fe/P dual doping boosts the activity and durability of CoS2 polycrystalline nanowires for hydrogen evolution, J. Mater. Chem. A, 2019, 7, 5195–5200 RSC.
- L. Ji, J. Wang and X. Teng,
et al., CoP nanoframes as bifunctional electrocatalysts for efficient overall water splitting, ACS Catal., 2019, 10, 412–419 CrossRef.
- X. Wu, S. Han and D. He,
et al., Metal organic framework derived Fe-doped CoSe2 incorporated in nitrogen-doped carbon hybrid for efficient hydrogen evolution, ACS Sustain. Chem. Eng., 2018, 6, 8672–8678 CrossRef CAS.
- J. G. Li, K. Xie and H. Sun,
et al., Template-directed bifunctional dodecahedral CoP/CN@MoS2 electrocatalyst for high efficient water splitting, ACS Appl. Mater. Interfaces, 2019, 11, 36649–36657 CrossRef CAS PubMed.
- F. Liu, W. He and Y. Li,
et al., Activating sulfur sites of CoS2 electrocatalysts through tin doping for hydrogen evolution reaction, Appl. Surf. Sci., 2021, 546, 149101 CrossRef CAS.
- T. Feng, F. Wang and J. Lei,
et al., A universal CoO/CoSe2 heterostructure electrocatalyst towards hydrogen evolution reaction via in situ partial surface-oxidation-selenization method, Mater. Chem. Phys., 2021, 267, 124644 CrossRef CAS.
- Z. Pu, I. S. Amiinu and C. Zhang,
et al., Phytic acid-derivative transition metal phosphides encapsulated in N, P-codoped carbon: an efficient and durable hydrogen evolution electrocatalyst in a wide pH range, Nanoscale, 2017, 9, 3555–3560 RSC.
- Y. Yu, Z. Peng and M. Asif,
et al., FeP Nanocrystals Embedded in N-Doped Carbon Nanosheets for Efficient Electrocatalytic Hydrogen Generation over a Broad pH Range, ACS Sustain. Chem. Eng., 2018, 6, 11587–11594 CrossRef CAS.
- A. Wu, C. Tian and H. Yan,
et al., Hierarchical MoS2@MoP core–shell heterojunction electrocatalysts for efficient hydrogen evolution reaction over a broad pH range, Nanoscale, 2016, 8, 11052–11059 RSC.
- J. Yu, G. Li and H. Liu,
et al., Electrochemical flocculation integrated hydrogen evolution reaction of Fe@N-doped carbon nanotubes on iron foam for ultralow voltage electrolysis in neutral media, Adv. Sci., 2019, 6(18), 1901458 CrossRef CAS.
- Y. Çimen, A. W. Peters and J. R. Avila,
et al., Atomic layer deposition of ultrathin nickel sulfide films and preliminary assessment of their performance as hydrogen evolution catalysts, Langmuir, 2016, 32, 12005–12012 CrossRef.
- M. Patel, K. K. Joshi and K. H. Modi,
et al., CuS nanoparticles: an efficient electrocatalyst for hydrogen evolution reaction in a wide pH range, Electrochim. Acta, 2023, 441, 141740 CrossRef CAS.
|
This journal is © The Royal Society of Chemistry 2025 |
Click here to see how this site uses Cookies. View our privacy policy here.