DOI:
10.1039/D4TB01995D
(Review Article)
J. Mater. Chem. B, 2025,
13, 117-136
Biomaterials enhancing localized cancer therapy activated anti-tumor immunity: a review
Received
5th September 2024
, Accepted 1st November 2024
First published on 6th November 2024
Abstract
Localized cancer therapies such as radiotherapy, phototherapy, and chemotherapy are precise cancer treatment strategies aimed at minimizing systemic side effects. However, cancer metastasis remains the primary cause of mortality among cancer patients in clinical settings, and localized cancer treatments have limited efficacy against metastatic cancer. Therefore, researchers are exploring strategies that combine localized therapy with immunotherapy to activate robust anti-tumor immune responses, thereby eradicating metastatic cancer. Biomaterials, as novel materials, exhibit great potential in biomedical applications and have achieved great progress in clinic translation. This review introduces biomaterials and their applications in research focused on enhancing localized cancer treatment activated anti-tumor immunity. Additionally, the current challenges and future directions of biomaterials are also discussed, providing insights and references for related research.
1. Introduction
Cancer is a complex-to-treat and high-risk disease with high morbidity and mortality rates. Countless patients suffer and die from cancer every year, which has reinforced the development of cancer diagnostic techniques and treatments.1 Cancer immunotherapy recognizes and kills cancer cells by boosting the immune system.2 After years of research, cancer immunotherapy has made significant breakthroughs as a cutting-edge cancer treatment, bringing better treatment to patients. Major immunotherapies include immune checkpoint inhibitors, tumor vaccines (e.g., Provenge, Cimavax), and cellular immunomodulators.3–8 These therapies offer new treatment options for cancer patients, but still face challenges in terms of safety and efficacy. Immunotherapy is often used in conjunction with traditional therapies to increase the effectiveness of treatment and reduce damage to the patient, and although combination therapies may improve treatment efficacy, they still have many limitations.9,10 Therefore, there is an urgent need to develop more stable and efficient tools to enhance anti-tumor immune responses while reducing patient injury and expanding the recipients of cancer immunotherapy.
Localized tumor therapy is a therapeutic strategy for primary tumors, offering both advantages and limitations.11 Local tumor therapy can directly act on the primary tumor, effectively control or eliminate the tumor, reduce the damage of surrounding normal tissues, and achieve precise treatment.12 For unresectable tumors or adjuvant treatments before and after surgery, local treatments can help reduce the tumor size, decrease the difficulty of surgery, and improve the success rate and therapeutic efficacy of surgery.13 In addition, local tumor treatment can be combined with radiotherapy, chemotherapy, etc. to synergize the effect of treatment to improve the therapeutic effect.14–16 However, local tumor therapy is only effective for primary tumors and cannot treat metastatic tumors or has poor therapeutic effect; after local tumor therapy, tumor recurrence or drug resistance may occur in some patients, which requires further treatment or adjustments to the treatment regimen; some of the techniques require high level of technical expertise and specialized equipment support, which limits their widespread application in clinics.17–21
In recent years, various biomaterials (e.g., nanoparticles, organic materials) have been extensively investigated for the enhancement of anti-tumor immune responses.22,23 Biomaterial-assisted localized therapies (e.g., radiotherapy, phototherapy) are used to enhance the effectiveness of cancer immunotherapy.24–26 The destruction of cancer cells induced by these therapies releases cancer antigens, which synergize with immunostimulants to form a topical immunization vaccine, thereby enhancing the overall anti-tumor immune activity.27 Developing biomaterials into site-directed medication transport systems allows for targeted drug delivery to cancerous areas, minimizing damage to healthy tissues and improving therapeutic efficacy.11,28 By regulating their structure and properties, these systems achieve controlled release of drugs, maintain the effective dosage level locally within the neoplasm, and enhance the controllability and safety of the treatment.29,30 A wide variety of biomaterials can be used to deliver chemotherapeutic and immunotherapeutic drugs, supporting the combined application of multiple treatment modalities to improve efficacy. Designs can be customized according to patient characteristics and tumor type, enabling personalized treatment plans.31,32 However, biomaterials also face the problems of immune escape and drug resistance, and drug release control needs to precisely regulate the release rate and extent to ensure both efficacy and safety.33 In addition, biomaterial treatments may lead to local adverse effects, for example inflammation and infection, which need to be closely monitored and managed. In the body, biological materials can act as foreign substances, leading to infections and worsening conditions. The structural changes of biological materials may result in functional impairment, damage to cell membranes, and DNA damage, thereby posing health risks.34 Non-biodegradable materials left in the body may accumulate over time, affecting the immune system and increasing the risk of toxicity.35 Therefore, selecting or developing materials with high biocompatibility is essential. Natural materials such as collagen, hyaluronic acid, and chitosan often have good biocompatibility due to their similarity to the body's internal environment.36 Surface modification techniques, such as modifying the surface of the material with bioinert molecules (such as polyethylene glycol, PEG) can reduce unnecessary interactions with proteins and cells, thereby reducing inflammatory responses and rejection reactions.37,38
This article provides a review of recent advances in biomaterial-assisted other topical treatments to enhance anti-tumor immunity, in addition to the delivery of anti-tumor drugs by biomaterials for cancer immunotherapy (Scheme 1). We hope that this review article will assist scientists have a broad understanding of recent perspectives of localized anti-tumor immunotherapy combined with biomaterials technology, which may stimulate new brainstorming about anti-cancer immunotherapy.
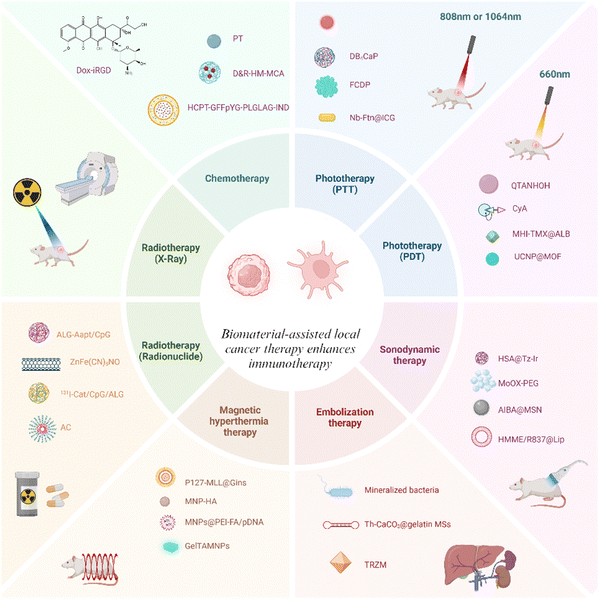 |
| Scheme 1 Schematic illustration of biomaterials enhancing localized cancer therapy activated anti-tumor immunity. | |
2. The application of biomaterials to enhance immune responses in localized tumor therapy
The immune system, as a strong line of defence in the human body, cannot be ignored for tumor defence. However, tumor cells evade the host immune system by means of altering their biological properties.39 As a result, tumor immunology has increasingly focused on the role of immune factors in tumors.40,41 Unlike traditional drug treatments for cancer, immunotherapy harnesses the patient's immune system to combat cancer.42 Although advances have been made in immunotherapy, traditional cancer immunotherapy still has many drawbacks, such as the need for repeated high-dose injections of drugs and side effects.43 A wealth of recent research indicates that the combination of immunotherapy and biomaterials can be freed from a number of limitations.44
Intravenous delivery of targeted drugs is difficult to localize within the tumor due to the lack of desirable molecular features and biological barriers that impede the therapeutic effect.45,46 Thus, localized drug delivery to tumours has emerged as a new possibility.47 Local drug delivery to tumors based on biomaterials is a new approach, and these materials include natural and synthetic, e.g. inorganic nanoparticles, hydrogels, and microspheres.48–51 We briefly discuss advances in biomaterials for localized tumor therapy (Table 1). These materials are biocompatible and biodegradable and can be used as carriers or modulators to deliver immunomodulators to the local tumor for tumor-targeted immunotherapy.52–54 Biomaterials have the advantage of design flexibility, e.g. flexible control of loading and release of immunological drugs.55,56 The goal of local drug delivery is to enhance the safety and effectiveness of therapy, and targeted drug delivery to the cancer site via carriers and affixes is consistent with this goal.57 Over the years, researchers have used biomaterials to enhance the immune system's ability to treat tumors.58,59 Cancer immunotherapy has significantly transformed traditional treatment methods. Biomaterials can modify the tumor microenvironment, improving the immune system's capacity to identify and eliminate tumors. This provides new avenues for cancer treatment.
Table 1 Summary of utilizing biomaterials to enhance topical treatments
Local treatment modalities |
Formulations |
Tumor models |
Ref. |
Chemotherapy |
Dox-iRGD |
4T1 |
60
|
T-iLNTB |
B16F10, E0771 |
61
|
E@Fe-DOX |
4T1 |
62
|
NCTD-Pt |
4T1 |
63
|
HCPT-GFFpYG-PLGLAGIND |
B16-F10, CT26 |
64
|
MTO/IND liposome |
EMT6, CT26 |
65
|
CpG-EXO/TGM |
GL261 |
66
|
5IOX1@DOX liposomal |
4T1, CT26, HCT116, MCF-7 |
67
|
|
Radiotherapy |
125I-VNP/131I-VNP |
CT26 |
68
|
AlgBi2S3@BSA |
4T1 |
69
|
IBM |
TRAMPC1, Panc-02 |
70
|
Metal-DNA MXF |
CT26 |
71
|
Pd NSs |
MCF-7 |
72
|
L-NILMDP |
MOC1 |
73
|
Ch/γ-PGA NPs |
4T1 |
74
|
GTe-RGD |
A375, HeLa, 4T1 |
75
|
|
Photothermal therapy |
IMQ@IONs/ICG |
Panc02-H7 |
76
|
Ti3C2-PEGOVA-Mn2+ |
LM8 OS |
77
|
MIL-100@MTO@HA |
CT26 |
78
|
CpG@PDA-FA |
CT26 |
79
|
NYF@BP |
4T1 |
80
|
APTEDB-cyNP@CpG |
CT26 |
81
|
R837-OVA-PEG-MnFe2O4 |
4T1 |
82
|
|
Photodynamic therapy |
RHPPE |
4T1 |
83
|
PLM-R837-ALG hydrogel |
4T1 |
84
|
FM@VP |
MDA-MB-231 |
85
|
CPIM |
B16F10 |
86
|
@E7-ICG-BSA nanovaccines |
TC-1 |
87
|
CP+-CpG NPs |
HeLa |
88
|
HPR@CCP |
B16F10 |
89
|
MON |
B16-OVA, B16F10 |
90
|
|
Sonodynamic therapy |
PEG-CDM-aPD-L1/Ce6 |
B16F10 |
91
|
FA-MnPs |
4T1 |
92
|
PIMS NPs |
4T1 |
93
|
EIPS |
4T1 |
94
|
PMPS NDs |
Panc02 |
95
|
Zr-TCPP(TPP)/R837@M |
4T1 |
96
|
Zn-TCPP/CpG nanosheets |
CT26 |
97
|
|
Embolotherapy |
LM MSs |
VX2, H22 |
98
|
TRZM |
HuH-7, VX2 |
99
|
Th-CaCO3@gelatin MSs |
N1S1 |
100
|
M@FS |
CT26, B16F10, KPC, VX2 |
101
|
|
Hyperthermia therapy |
Zn–CoFe2O4@Zn–MnFe2O4 |
HepG2 |
102
|
FSH |
4T1 |
103
|
Ir@MnFe2O4 NPs |
HeLa |
104
|
CoFe2O4@MnFe2O4 |
4T1, U87 |
105
|
RBCM-PFH-IONP-R837 |
4T1 |
106
|
SPIONs |
CT26, 4T1 |
107
|
MSCH |
H22 |
108
|
The ideal biomaterials for cancer treatment should possess good stability and biocompatibility, be friendly to organ tissues, and exhibit stable drug release kinetics. Currently, common cancer treatment methods include chemotherapy, radiation therapy (RT), etc. However, single treatment modalities can cause toxic side effects in healthy tissues. Therefore, combining biomaterials with enhanced immunotherapy can achieve better therapeutic outcomes. This approach enables more comprehensive and personalized treatment strategies by inducing immune responses, enhancing immune system activity, and identifying and eliminating tumor cells. Such combined therapies provide multiple effective avenues for tumor treatment, offering better outcomes for cancer patients.
In general, moderate-sized (10–100 nm) nanoparticles passively target drugs to tumors to achieve maximum efficacy.109 The shape of the nanoparticles can affect the accumulation of drugs at the tumor site, and the electrostatic interaction affects the recognition of immune cells, which in turn affects the recognition and phagocytosis of immune cells.110 Collagen can improve mechanical properties by lowering temperature and increasing fiber density.111 It is understood that most anticancer drugs are hydrophobic, and their solubility can be increased with the help of biological materials such as water solvents and micelles.112 The porous scaffolds of drugs designed with biomaterials such as alginate and hyaluronic acid can recruit immune cells.113 Some environmentally sensitive biomaterials, such as elastin and hydrogels, are liquid at low temperatures and convert to a gel state at body temperature to control drug release.114 Biomaterials with low permeability, such as hyaluronic acid and polycaprolactone, increase their transdermal ability by using pressure osmosis.115 Controlled drug delivery using cell adhesion properties of biomaterials, such as hyaluronic acid and stromal gel, helps control immune cells to treat tumors.116 Biodegradable embolized microspheres made of biological materials (such as sulfonate-modified polyvinyl alcohol (PVA) and polyethylene glycol acrylate (PEGDA), etc.) can deliver chemotherapy drugs and radiotherapy drugs directly into the blood vessels of liver tumors and use the size characteristics of the microspheres to block the tumor branch blood vessels in the liver, thus cutting off the tumor support.117
2.1. Biomaterial-assisted local chemotherapy enhances immunotherapy
Chemotherapeutic drugs are a class of medications used to treat cancer. These drugs affect cancer cells by preventing DNA replication, inhibiting protein synthesis, and disrupting cellular metabolic processes.118 Chemotherapy can be used alone or with other therapeutic approaches, such as surgery.60,119 Chemotherapeutic drugs can effectively kill cancer cells, but they also impact normal cells, causing side effects such as nausea and vomiting, and resistance is the key factor that limits the effectiveness of chemotherapy.120,121 It is reported that cancer patient mortality is related to drug resistance, which may be associated with genetic factors and growth factors. This resistance reduces the effectiveness of administered drugs, making tumor treatment more challenging.122–124 To address drug resistance, chemical enhancers are introduced by introducing biological materials that enhance drug sensitivity and help overcome resistance.125 Delivering chemical enhancers to tumors while avoiding normal tissues, biomaterials in combination with chemotherapy have been shown to improve the reversal of drug resistance through a variety of mechanisms, including targeting drugs, enhancing cellular uptake, improving bioavailability of drugs with poor physicochemical properties, and reediting immunosuppressive microenvironments.126
To reduce immunotherapy resistance, David J. Mooney's research group proposed a method combining immunogenic chemotherapy with pore alginate gel.60 Local drug release through the intratumoral injection of pore-forming alginate gels loaded with a granulocyte-macrophage colony-stimulating factor (GM-CSF) and doxorubicin- iRGD (Dox-iRGD) induces the immunogenic death of breast cancer cells. Furthermore, the utilization of the TLR9 agonist CpG enhances the antitumor immune response (Fig. 1a). The experimental results showed that the drug effectively reduced the rate of release compared to the drug without the use of pore alginate gel, achieving a longer drug release and potentially aiding tumor penetration. This biomaterial activates and enhances the response of dendritic cells to tumor antigens, can focus DCs on the tumor site, sample and process TAAs from dying tumor cells, significantly slow the growth of 4T1 tumors and reduce metastasis, achieving excellent results in multiple aspects while having minimal systemic toxicity.
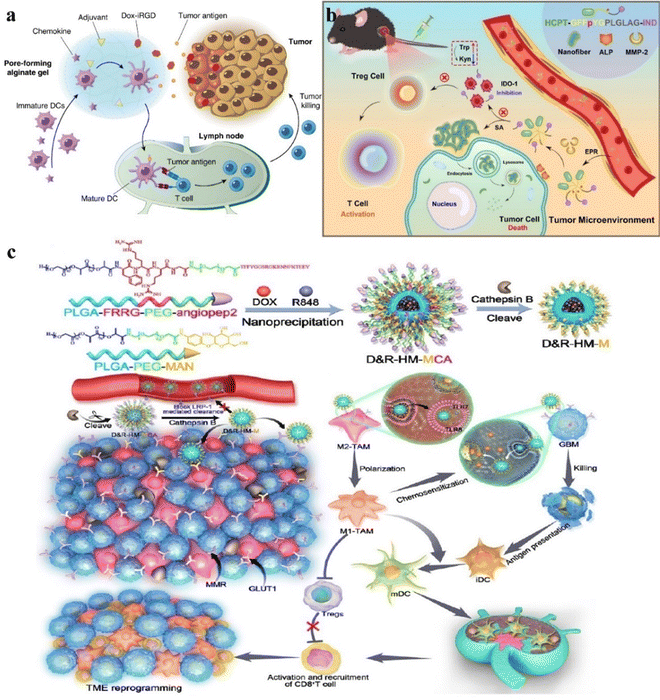 |
| Fig. 1 (a) Schematic of cancer vaccines using biomaterials to deliver immunological and chemotherapy drugs for localized tumor treatment. (b) Schematic of peptide preparations with dual enzyme reactivity. (c) Schematic of GBM treatment of D&R-HM-MCA. | |
For some highly malignant cancers, there are currently no symptomatic therapies available. Traditional chemotherapeutic drugs often lack specificity and can cause damage to normal tissues. Therefore, to improve the effectiveness of traditional medicines, Yang's research group propounded a new approach by using dual-enzyme reactions to build chemical immunotherapy drugs.64 Extracellular ALP and matrix metalloproteinase 2(MMP-2) effectively regulate the in situ self-assembly of Comp. 1 into nano-fibers that enter tumor cells and set free the immune molecule (IND). This stimulates the immune cells to clear the cancer cells (Fig. 1b). Comp. 1 leaves the lysosome and enters the cytoplasm, inducing apoptosis in cancer cells. These results showed that the peptide preparation exhibited the ability of long-term slow release, reduced the drug loss during systemic circulation and non-specific binding significantly, and the lysosomal degradation could neutralize the toxic side effects of free HCPT, thus improving the inhibitory effect of solid tumors. This combined chemotherapy and immunotherapy approach has demonstrated excellent therapeutic efficacy in cancer treatment.
Glioblastoma (GBM) is a highly aggressive tumor that causes severe cranial brain damage, primarily treated with surgery, radiotherapy, and chemotherapy.127 Targeting tumour-associated macrophages (TAMs) in combination with traditional chemotherapy has become a novel approach for treating GBM.128 To overcome the blood–brain barrier, biomaterials are used to increase drug penetration. Ruan and colleagues proposed a CTSB sensitive system directed at the brain system (D&R-HM-MCA) designed for precision release to target both TAM and GBM simultaneously.129 By cleaving and releasing CTSB-responsive peptides to bypass LRP1-mediated efflux, this system achieves chemotherapy-induced cytotoxicity against GBM and induces TAM phenotypic differentiation, enhancing the anti-GBM immune response and offering a novel approach for GBM therapy (Fig. 1c). The experimental results showed that CTSB reduced drug effection, enhanced drug targeting and enrichment ability, induced the apoptosis of GL261 cells, activated the systemic anti-tumor immune response, and enhanced the anti-GBM immune response and long-term immune monitoring compared to drugs without biomaterials.
Due to continuous angiogenesis in tumor tissues, vascular structures are relatively incomplete compared to normal tissues, which can lead to tumor necrosis by destroying tumor blood vessels. The toxicity of chemotherapy drugs is too high, in order to improve the treatment efficiency of drugs under limited dose conditions. The Tang and Yu groups co-encapsulated PSM and TPZHex with mPEG-b-PDLLA to form nanoparticles (PT-NPs).130 In the tumor microenvironment, overproduction of esterases excites PT-NPs to release plitidepsin to disrupt vasculature and induce hypoxia, activating TPZHex to kill tumor cells (Fig. 2). The experimental results showed that compared with drugs without nanoparticles, the treatment of 4T1 tumors showed that the biomaterials increased the drug tolerance dose and the drug release ability and enhanced drug utilization. It accelerates the release capacity under esterase and shows minimal side effects, leading to insufficient oxygen supply within the tumor and aggravating intratumor hypoxia. The tumor suppression rate of 92.9% and the cure rate of 20% were achieved, and tumor metastasis was significantly inhibited.
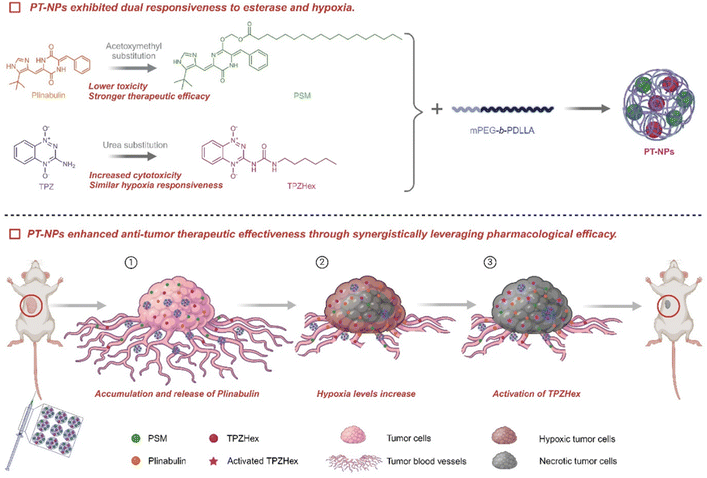 |
| Fig. 2 Schematic of the synergistic antitumor mechanisms of PT-NPs. Esterase and anoxy-responsive nanomedical drugs (PT-NPs), combined with chemotherapy, release plitidepsin at the tumor site, damaging blood vessels, causing nutrient and oxygen deficiencies to improve synergistic efficacy and reduce toxicity. | |
2.2. Biomaterial-assisted local radiotherapy enhances immunotherapy
External beam ionizing radiation and therapeutic radioactive isotopes for tumor destruction have been widely used in clinical practice.131–133 Radiation-induced DNA damage leads to cell death and sends out endogenous danger signals, a process known as immunogenic cell death (ICD).134 During ICD, dying tumor cells express calreticulin (CRT), release adenosine triphosphate (ATP), and high mobility group box-1 protein (HMGB1), which stimulate dendritic cells (DCs) to engulf them.135–138 Activation of the immune system allows RT to sometimes exhibit distant effects.139 Biomaterials can achieve accurate radiation therapy, activate the immune system, reduce damage to non-tumor tissues, achieve more accurate fixation and retention, and further improve the therapeutic effect.
To address the problem of inadequate anti-tumor immune response, Liu's research group constructed an ATP triggered CpG-releasing hydrogel using sodium alginate and an ATP-specific aptamer.140 Following local injection into tumors, this hydrogel releases CpG, leading to the escape of ATP from dying tumor cells and increasing the immunogenicity of tumor-associated antigens (Fig. 3a). The experimental results show that this smart hydrogel is preserved in the tumor and released stably, which can cooperate with radiotherapy to make the violent release of ATP in the tumor, which can not only effectively suppress established tumors and distant metastases but also inhibit tumor recurrence through the generation of long-lasting immune memory. It induces a 100% tumor rejection upon re-inoculation, extending the survival cycle of animals, reducing side effects, and avoiding the need for repeated radiotherapy.
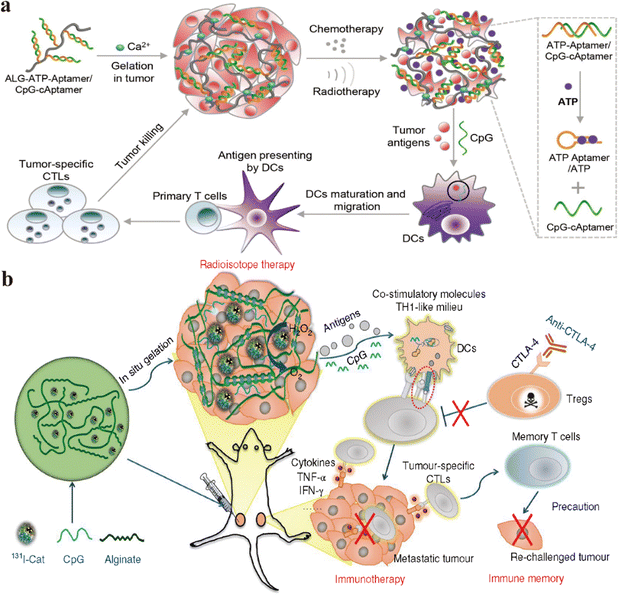 |
| Fig. 3 (a) Schematic diagram illustrating hydrogels enhancing immune response during RT for tumor treatment. (b) Schematic of the mechanism of anti-tumor response through combined radioimmunotherapy (RIT) and immune reaction based on 131I-Cat/CpG/ALG. | |
To improve traditional radiotherapy (RT), Liu's research group designed a hydrogel-based therapeutic system, incorporating 131I-labeled catalase (131I-Cat) mixed with alginate (ALG) for tumor injection (Fig. 3b).131 The experimental results show that calcium ions trigger ALG to form a gel, ensuring uniform distribution of 131I-Cat within tumors and preventing leakage into normal tissues, while injection of 131I-cat alone migrated to other organs after local injection, with almost no tumor remaining at 48 hours. This strategy combines local radiotherapy with systemic immune checkpoint blockade (ICB), effectively suppressing tumor metastasis and recurrence, and 100% local tumor elimination was achieved across multiple animal models. The introduction of the immunoadjuvant CpG further enhances systemic antitumor immune responses. This local treatment approach holds potential clinical significance for advanced cancer patients.
Wang et al. reported an enhanced cancer immunotherapy method using antigen-capturing nanoparticles (AC-NPs).141 Research has shown that AC-NPs can deliver tumor antigens to antigen-presenting cells, and αPD-1 therapy shows significant effectiveness in the B16F10 melanoma model, with a cure rate as high as 20% (Fig. 4a). In-depth studies reveal that AC-NPs enhance the proliferation of cytotoxic CD8+ T cells to activate a more robust immune response. Tumor metastasis leads to aggravation and even death in cancer patients, hence treating solid tumors and inhibiting metastasis are crucial. To improve the efficacy of RT and immunotherapy, Liu and Wang's research groups proposed a two-dimensional nanoplatform by preparing ZnFe(CN)5NO monolayered nanosheets by mixing zinc ions with nitroprusside and labeling them with 32P (Fig. 4b).142 These results showed that in the CT26 model, ZnFe(CN)5NO labeled 32P exhibited long-lasting tumor accumulation, avoiding potential side effects caused by leakage. 32P-induced Cherenkov luminescence stimulates a sustained release of NO on the nanosheets, and high concentrations of NO induce a higher immune response, resulting in distal inhibition by treating proximal tumors in a mouse bilateral CT26 model. These 32P-labeled nanosheets showed strong anti-tumor effects when used in combination with ICB therapy.
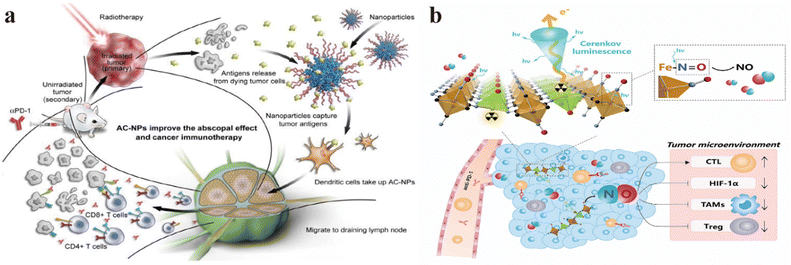 |
| Fig. 4 (a) Schematic diagram illustrating the use of AC-NPs in combination with RT to enhance cancer immunotherapy. (b) Schematic of the application of monolayered two-dimensional ZnFe(CN)5NO nanosheets to better RT and immunotherapy for cancer treatment. | |
2.3. Biomaterial-assisted local phototherapy enhances immunotherapy
Phototherapy is a rising cancer treatment modality that includes photothermal therapy (PTT) and photodynamic therapy (PDT).143 PTT utilizes photosensitizers (PSs) to generate heat energy under light irradiation, effectively “burning” cancer cells. PDT, on the other hand, relies on PSs to convert oxygen molecules into cytotoxic singlet oxygen species under specific light exposure, thereby killing cancer cells.144,145 These two methods can directly destroy tumor cells while also stimulating antitumor immune responses.146,147 Studies have shown that tumor cells dying after phototherapy release tumor danger signals, which attract immune cells for clearance, thereby triggering a strong immune response.148–150 In addition, phototherapy also promotes the generation of various immunogenic factors, such as the expression of chimeric antigen receptors (CARs), cytokine production, and the release of apoptotic cancer cell components.151 At the same time, phototherapy also has obvious disadvantages, such as limited depth of light and damage to healthy tissues. The combination of high-quality biological materials can enhance the absorption of light (such as gold nanoparticles) and stimulate the production of long-term immune memory, and can also be designed into temperature or pH responsive photosensitizer release systems, thereby improving the effectiveness and safety of phototherapy.152
2.3.1. Biomaterial-assisted local photothermal therapy enhances immunotherapy.
The development of nanobiotechnology combines photothermal therapy with immunotherapy, but low levels of antigen presentation and a suppressive tumor immune microenvironment impede this action.153,154 To overcome these obstacles, Zhu and Zhao et al. developed multifunctional carrierless nanoparticles (FCDP-NPs) containing multiple immune factors.155 After intratumoral injection, FCDP-NPs capture tumor-associated antigens under laser irradiation to form nanovaccines (FCD-NPs@TAAs) and further activate immune responses to enhance tumor clearance (Fig. 5a). The nanovaccine significantly improved survival and prevented tumor metastasis, providing a platform for synergy between photothermal therapy and immunotherapy. To enhance the response rate and tolerance of immunotherapy, Liu et al. developed a multi-valent immune checkpoint therapy platform by site-specifically conjugating anti-PD-L1 nanobodies onto ferritin nanocages (NB-FTN).156 NB-FTN intercepts the PD-1/PD-L1 reaction via endocytosis, downregulating PD-L1 levels, and encapsulates the FDA-approved dye indocyanine green (ICG) for PTT (Fig. 5b). This platform induces ICD of tumor cells, enhances immune responses, eliminates primary tumors, suppresses metastasis and ectopic tumors, and prolongs survival rates.
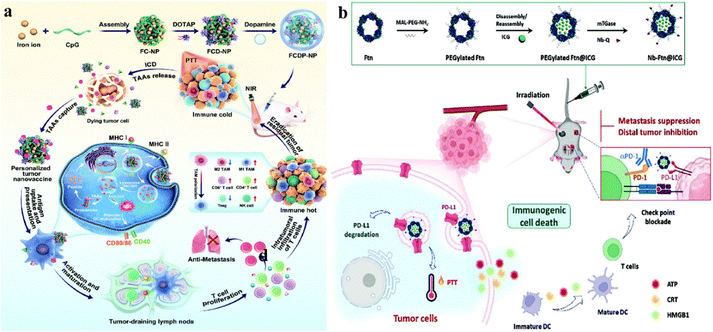 |
| Fig. 5 (a) Schematic diagram illustrating the in vivo assembly of specific nano-vaccines by FCDP-NPs for synergistic photothermal-immunotherapy. (b) Schematic for the preparation of Nb-FTN@ICG and its synergistic PTT role in immunotherapy. | |
Feng and Liu et al. developed a multifunctional photosensitizer (DB4CaP nanoparticles) with photothermal conversion capability, near-infrared-II (NIR-II) fluorescence, and cGAS-STING activation ability.143 After intravenous administration, DB4CaP nanoparticles accumulate in the tumor area and effectively eradicate primary tumors in mice under 915 nm laser irradiation, achieving a complete remission rate of 83.3% (Fig. 6a). These nanoparticles synergize with anti-PD-1 immunotherapy by activating antitumor immune responses to suppress the expansion of far-off non-irradiated and rechallenged tumors. Huang and Shang groups developed a biodegradable NIR-II photothermal-responsive polymer nanoparticle (PTEQ) system, which inherently possesses immunostimulatory properties and delivers siPD-L1 siRNA to enhance immune responses to treat cancer.157 In the CT26 tumor-bearing mouse model, PTEQ significantly promoted the penetration of immune cells (e.g., CD4+, CD8+ T cells) into the tumor microenvironment. Through NIR-II photothermal therapy, PTEQ activated FT cells and dendritic cells within the tumor. Complete tumor eradication was achieved in 7/10 cases, resulting in a 100% survival rate (Fig. 6b). In vivo vaccination experiments showed that the PTEQ/siPD-L1 + laser group achieved a 100% clearance rate of unilateral tumors. In a bilateral tumor model, PTEQ facilitated both primary tumor ablation and suppression of secondary tumors, promoting the induction of systemic immune responses.
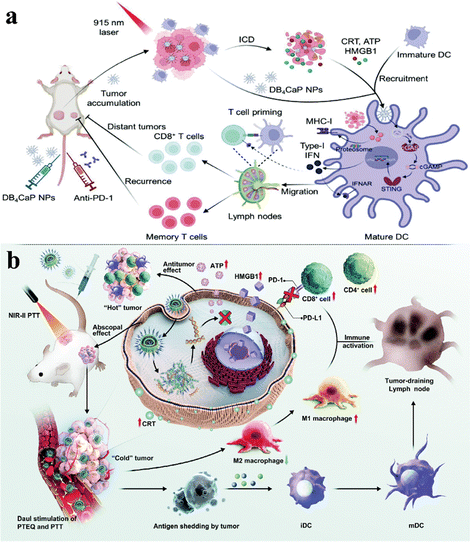 |
| Fig. 6 (a) DB4CaP NPs target tumors under the action of a 915 nm local laser, elicit an immune response, and collaborate with PD-1 inhibition to combat primary tumors and metastases. (b) Schematic diagram illustrating PTEQ-loaded siPD-L1 combined with PTT to activate immune-mediated tumor therapy. | |
2.3.2. Biomaterial-assisted local photodynamic therapy enhances immunotherapy.
Stand-alone immunotherapy is difficult to exclude distantly metastatic tumors. Photodynamic immunotherapy (PDI) involves the use of PDT and PSs to induce ICD to treat cancer.158 PDT effectively stimulates and enhances anti-tumor immunity by inducing ICD through cancer cell death, and combining PDT with immunotherapy is a promising approach for cancer treatment. Chen et al. developed a core–shell heterostructure by combining upconversion nanoparticles (UCNPs) with copper porphyrin metal–organic frameworks (Cu-porphyrin MOFs) for type I and type II PDT effects.159 UPIS exhibited a significant PDT effect under NIR light irradiation and a synergistic immunotherapeutic effect when the MOF pore was loaded with IDO/SNAP. The nanoplatform shows promising potential for the effective treatment of principal tumors and metastatic tumors.
The PS QTANHOH designed by Shi and Liu et al. exhibited excellent type I/II PDT performance, and cancer cells showed significant phototoxicity under blue laser irradiation.160 The half inhibitory concentration (IC50) is less than 10 nM under normoxic and hypoxic conditions (Fig. 7a). In addition, the active compounds inhibited HDACs, activated the immune microenvironment, and improved the efficiency of PDT in breast cancer in immunoreactive BALB/c mice, leading to the elimination of solid tumors and inhibition of metastasis. An aminopeptidase N (APN)-activated Type I phototherapy probe (CyA) was reported by Miao and Li et al. for anti-hypoxic PDT and immunotherapy to effectively treat cancer.161 CyA generates active photothermal molecules upon APN-induced substrate cleavage, specifically activates near-infrared fluorescence, photoacoustic signaling, and phototoxicity, and exhibits specific imaging and phototoxicity effects on tumor cells overexpressing APN under normoxic and hypoxic conditions (Fig. 7b). Thus, locally PDT combination with systemic immunotherapy improved the efficiency of tumor suppression in mice.
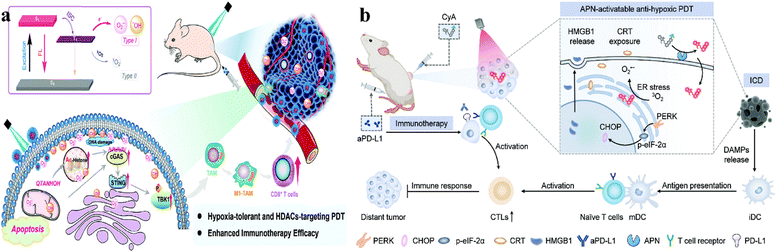 |
| Fig. 7 (a) QTANHOH NPs targeting tumors, combined with PDT immunotherapy for tumors and the principle of reactive oxygen generation. (b) Aminopeptidase N (APN) activates the Type I phototherapy drug probe (CyA), an anti-hypoxic PDT immunotherapy for cancer treatment. | |
2.3.3. Biomaterial-assisted local photothermal/photodynamic therapy enhances immunotherapy.
The therapeutic efficacy of PTT may be compromised by light attenuation and uneven distribution of PSs, leading to uneven heat distribution within tumor tissues.162 Additionally, high temperatures may cause damage to normal tissues. PDT utilizes PSs under laser irradiation of specific wavelengths to react with molecular oxygen, generating 1O2, which damages tumor cells through various mechanisms.163 However, the effectiveness of PDT is limited by the severely hypoxic environment of tumors. Therefore, standalone PTT or PDT therapies have inherent limitations.164 Synergistic PTT/PDT therapy can enhance the effectiveness of PDT by increasing tumor blood flow and improving oxygen supply.165,166
Shen and Qi groups proposed a novel nano-platform integrating aggregation-induced emission agents and Prussian blue nano-catalysts for tumor immunotherapy.167 This platform incorporates AIEgens into porous PBNPs and encapsulates them within M1 macrophage membranes, enhancing NIR-II fluorescence brightness and PDT properties (Fig. 8a). Additionally, PBNPs stimulate tumors to produce hydrogen peroxide, which decomposes to release oxygen, thereby increasing PDT efficiency. The NIR absorption of PB also enhances photoacoustic imaging and photothermal effects. This nanomaterial not only significantly suppresses the growth of mouse breast cancer but is also used as a systemic cancer cell treatment option to prevent tumor recurrence in mice.
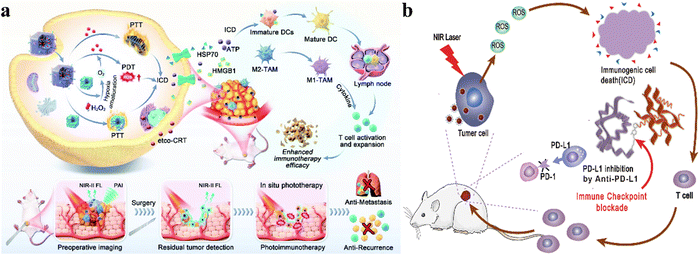 |
| Fig. 8 (a) Synthesis of near-infrared AIEgen and mesoporous PB nano-catalysts to boost the therapeutic performance of vigorous cancer immunotherapy guided by NIR-II and PA imaging. (b) Schematic of PTT/PDT combined ICG@SANPs-cRGD for tumor immunotherapy. | |
Tang et al. successfully prepared ICG@SANPs-cRGD multifunctional nanoparticles, which enhanced the efficacy of PTT/PDT, achieving the combination of tumor phototherapy and immunotherapy.168 ICG@SANPs-cRGD enhances PTT and PDT under near-infrared radiation, inhibiting proliferation and promoting the apoptosis of 4T1 tumor cells (Fig. 8b). Simultaneously, it effectively targets tumor tissues in vivo. The nanoparticles induce a tumor immunogenic environment that sensitizes tumors to PD-L1 therapy, achieving combined immunotherapy for breast cancer. This approach effectively suppresses distant metastatic tumors.
Biomaterial-based phototherapy induces necrosis and apoptosis of tumor cells, leading to cell membrane damage, triggering robust inflammatory responses and releasing antigens. Tumor tissues attract diverse immune cells, promoting the presentation of tumor-associated antigens to T cells, resulting in potent immune responses. When combined with ICB, it enhances systemic immune responses while reducing adverse reactions.
2.4. Biomaterial-assisted local sonodynamic therapy facilitates immunotherapy
The principle of ultrasound (US) involves converting electrical energy into high-frequency pulses. Its application mechanisms primarily include the generation of reactive oxygen species (ROS), mechanical damage, and thermal effects.169,170 Traditional low-frequency ultrasound is mainly used for diagnostic imaging, whereas high-frequency ultrasound can focus energy on a specific point, minimizing damage to surrounding tissues. In cancer treatment, high-frequency ultrasound can precisely focus sound waves to destroy cancer cells without harming healthy tissues. It can be used independently for treatment, can enhance the efficacy of existing drugs and also facilitate drug delivery or ablation by adjusting intensity.171 The sonodynamic therapy has some limitations such as insufficient acoustic efficiency and poor therapeutic performance, and biomaterials can be used as acoustic sensitizers to enhance the sonodynamic effect.
In recent years, researchers have targeted cancer ultrasound therapies that combine biomaterials with ultrasound to stimulate the immune system for cancer treatment. This includes placing non-toxic sensitizers at tumor sites and activating them with ultrasound waves to generate significant amounts of ROS. This process induces cytotoxic effects on tumors, effectively killing cancer cells.172
Chen and Zhang et al. reported nanosonosenitizers (HMME/R837@Lip) that encapsulate sonosensitizers and immune adjuvants.173 Enhanced sonodynamic therapy (SDT) combined with anti-PD-L1 immunotherapy achieved efficient tumor treatment. After systemic administration, HMME/R837@Lip nanosonosensitizers, under repeated non-invasive ultrasound irradiation, caused tumor cell death and significantly inhibited both primary tumors and distant metastases (Fig. 9a). This combination therapy not only enhanced immune responses but also conferred long-term immune memory, preventing tumor recurrence. The components used are FDA-approved drugs, highlighting their potential for clinical application.
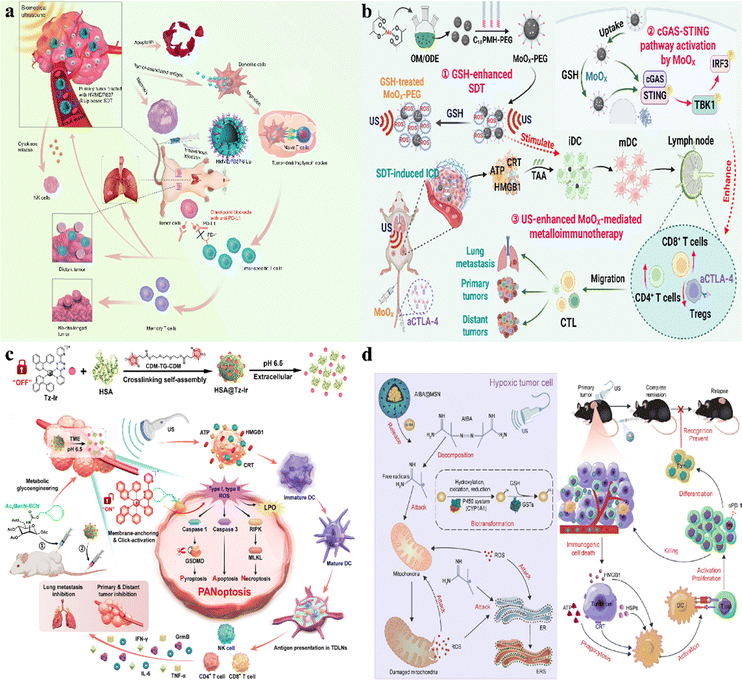 |
| Fig. 9 (a) Schematic diagram of SDT combined with an immunoadjuvant-containing nanosonic sensitizer for tumor immunotherapy. (b) Schematic diagram of MoOX-PEG combined with SDT to enhance the immune response against tumors. (c) Schematic diagram of HSA@Tz-Ir to enhance tumor-specific immunogenic therapy induced by SDT. (d) Schematic diagram of AIBA@MSN-based combination with SDT for immunotherapy of cervical cancer. | |
Cheng et al. utilized MoOX nanomaterials as ultrasound catalysts to amplify oxidative stress and enhance metal-based immunotherapy.174 They successfully synthesized and modified MoOX-PEG NPs with efficient ROS generation capability under ultrasound irradiation. MoOX-PEG NPs, under ultrasound (US) irradiation, enhance intratumoral oxidative stress, induce ICD, and weaken tumor antioxidant capacity by depleting glutathione (GSH) (Fig. 9b). Additionally, they stimulate dendritic cell (DC) maturation, activating cellular immune responses. MoOX-triggered SDT along with anti-CTLA-4 therapy enhances antitumor efficacy, inhibits tumor metastasis, and activates systemic immune responses, demonstrating no significant side effects. This integration of GSH-enhanced SDT and metal-based immunotherapy holds promising prospects for cancer treatment.
Cai and colleagues developed a membrane-anchored clickable nanosonosensitizer (HSA@Tz-Ir) to enhance tumor sonodynamic immunotherapy by inducing tumor-specific pan-apoptosis.175 The nanosonosensitizer HSA@Tz-Ir releases the inactive Tz-Ir in the acidic tumor microenvironment. Tz-Ir achieves click activation and membrane localization by cross-linking with intratumoral BCN receptors. Under ultrasound irradiation, it generates ROS, severely damaging the tumor cell membrane and achieving efficient SDT (Fig. 9c). This membrane-targeted SDT inhibits primary and metastatic tumors and also induces immunogenic pan-apoptosis, eliciting a robust antitumor immune response.
To overcome the issues of low immunogenicity and solid tumor hypoxia, Hua and Qiu et al. designed a nanosensitizer AIBA@MSN to address the challenges of low immunogenicity and hypoxia in solid tumors.176 Upon ultrasound stimulation, AIBA@MSN spontaneously decomposes into highly oxidized azo radicals, independent of oxygen. The strong oxidative stress generated by these radicals disrupts the activity of electron respiratory chain (ETC) complexes, leading to mitochondrial membrane potential collapse and subsequent tumor mitochondrial destruction (Fig. 9d). This process induces ER stress and DAMPs release via SDT, promoting tumor ICD and dendritic cell (DC) maturation, which activates cytotoxic T lymphocyte (CTL) responses to mount an immune defense against the tumor. Combined with PD-1 blockers, SDT enhances the low response rates and provides relapse protection in tumor treatment, laying a theoretical foundation for oxygen-independent SDT-triggered immunotherapy.
2.5. Biomaterial-assisted embolization therapy promotes immunotherapy
Transarterial embolization (TAE) is a method that selectively blocks the blood vessels supplying the tumor using a microcatheter system administered through the arteries.177 So, the liver is the first choice for this type of treatment because the liver is mainly supplied with blood by the portal vein and the hepatic artery.178 The portal vein works for a healthy liver, while the hepatic artery delivers blood to the tumor. However, traditional embolization materials often have unsatisfactory effects, and biological materials such as collagen, hyaluronic acid, gelatin and other biological materials with better biocompatibility and efficacy are selected to improve treatment safety and effectiveness.
Some patients with hepatocellular carcinoma (HCC) are unable to undergo surgical resection of the cancerous tissue. However, transarterial embolization (TAE) can offer the advantage of minimally invasive treatment. Liu and Feng groups utilized a biphasic microfluidic approach to directly incorporate thrombin into CaCO3-encapsulated gelatin microspheres, creating procoagulant embolic microspheres (Th-CaCO3@gelatin MSs).100 It released thrombin and Ca2+ in the acidic tumor environment, promoting intravascular thrombus formation, achieving complete occlusion of tumor blood vessels, and effectively suppressing liver cancer in rats without any side effects (Fig. 10a). This method also activates anti-tumor immune responses, increases infiltration of CD8+ T cells into tumors, and modulates the tumor immune-suppressive microenvironment, demonstrating the potential of embolization therapy.
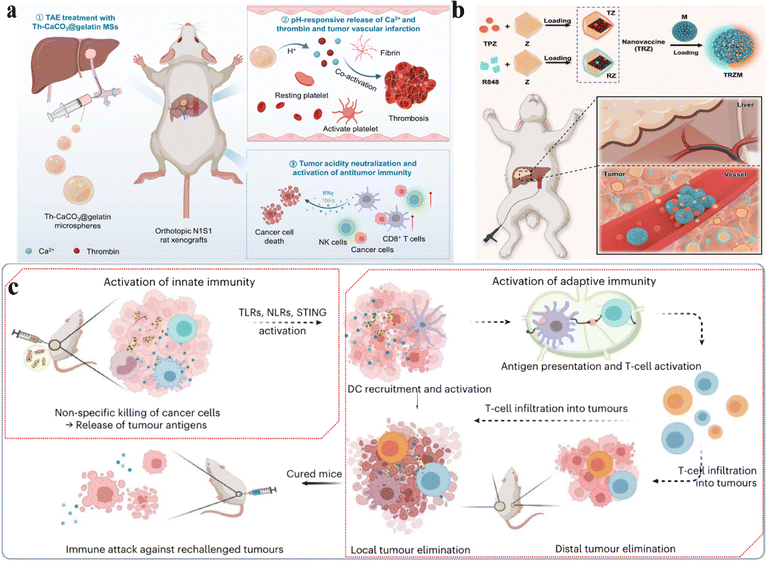 |
| Fig. 10 (a) Schematic of the enhanced anti-tumor immune response of Th-CaCO3@gelatin MSs. (b) Schematic of the application of embolic agent TRZM in hepatic tumor treatment. (c) Schematic diagram illustrating the mechanism of mineralizing bacteria in tumor immune therapy. | |
Embolization materials aid in depriving cancer cells of nutrients and oxygen, while immune checkpoint inhibitors (ICIs) exhibit high immunotoxicity. To tackle the non-responsiveness to immune checkpoint inhibitors (ICIs) and the organ toxicity in patients with hepatocellular carcinoma (HCC), Liu and Yan's research team proposed a nano-drug approach involving the incorporation of tirapazamine (TPZ) and the immune adjuvant R848 to formulate tumor nano-vaccines (TRZ).99 TPZ activates the generation of highly reactive free radicals in hypoxic environments, causing DNA strand breaks and toxicity to topoisomerase II, simultaneously reducing the expression of hypoxia-inducible factor-1α (HIF-1α) and vascular endothelial growth factor. The nano-vaccine can be encapsulated within porous gelatin microcarriers, forming functional embolic agents (TRZM). Administered via arterial routes into tumor-feeding arteries, this approach achieves high drug concentrations and potent anti-tumor activity while reducing systemic toxicity (Fig. 10b). In a rabbit VX2 orthotopic liver tumor model, this combined treatment strategy significantly enhanced the therapeutic efficacy against liver cancer, promising potential application in clinical transarterial chemoembolization (TACE).
Oncolytic bacteria can elicit innate immune activity. However, the antitumor effects of inactivated bacteria are limited, and attenuated live bacteria pose substantial safety risks. Here, Liu et al. developed a method for lysing and mineralizing bacteria by growing nanostructured manganese dioxide on the surface of inactivated bacteria, resulting in the formation of mineralized bacteria (e.g., mineralized FS).101 When injected into tumors, these mineralized bacteria effectively activated both innate and adaptive immunity, demonstrating significant anti-tumor effects in various animal tumor models (Fig. 10c). Compared to BCG bacteriotherapy and high-dose Oncorine lysovirus, the mineralized bacteria exhibited greater anti-tumor efficacy. Even at lower doses, they provoked systemic immune responses and built long-term immune memory, assisting in the prevention of tumor recurrence.
Overall, biomaterial-based local delivery systems offer a highly effective method for local immunotherapy, facilitating the triggered release of immunotherapeutic agents. Furthermore, this approach can circumvent the adverse effects linked to the systemic administration of immunomodulatory therapies. Further research may prioritize refining these biocompatible delivery systems to achieve systemic antitumor responses via localized administration of immunomodulatory therapies.
2.6. Biomaterial-assisted local magnetic hyperthermia therapy facilitates immunotherapy
Magnetic hyperthermia therapy (MHT) utilizes alternating magnetic fields to activate magnetic nanoparticles, inducing a heat effect that damages tumor cells through internal heating while sparing healthy tissues.179–181 Nanoparticles are precisely delivered to tumor sites using targeted delivery techniques. Under the action of a magnetic field, they convert into heat energy, thereby inducing apoptosis in tumor cells.98,182,183 Combining MHT with biomaterials can synergistically enhance immunotherapy, leading to cooperative tumor suppression.105,184 MHT releases tumor antigens, activates the immune system, increases vascular permeability locally, facilitates biomaterial delivery, and accelerates immune responses.185 Combining MHT with biomaterials enhances targeted therapy, strengthens systemic anti-tumor immune responses, minimizes the risk of recurrence and metastasis, and allows for personalized customization, thereby improving treatment efficacy.
Hu and Shi groups proposed a mild magnetic hyperthermia modulation method using a magnetic-responsive nanoplatform, MNPs@PEI-FA/pDNA (MPFD) to facilitate the growth and activation of tumor-infiltrating natural killer cells (NK cells) for in situ hepatocellular carcinoma immunotherapy.186 MPFD initiates mild remote magnetic thermal modulation (∼40 °C) in situ within hepatic tumors, triggering HSP70 promoter activation and inducing interleukin-2 (IL-2) cytokine gene transcription, thereby promoting the proliferation and activation of NK cells (Fig. 11a). In addition to in vitro experiments, in vivo experimental results demonstrate significant tumor suppression, highlighting the potential and mechanism of the magnetic-responsive nanoplatform in liver cancer therapy. While surgery is a common cancer treatment method, it can still lead to postoperative recurrence and metastasis. Postoperative immunotherapy for tumors has been proven to improve surgical outcomes. Therefore, Li and Adam groups developed a gelatin-tannic acid gel crosslinked bimetallic magnetically responsive hydrogel (GelTAMNPs) for mediating thermo-permeation-relay-immunotherapy, effectively inhibiting post-operative tumor recurrence and migration.187 GelTAMNPs produce hyperthermia under varying magnetic fields and liberate ions, reinforcing pyroptosis and enhancing the systemic immune response (Fig. 11b). When partnered with programmed death receptor 1 immune checkpoint blockade, this treatment significantly suppresses tumor reoccurrence and metastasis, as manifested in preclinical studies on B16F10 tumor-bearing mice.
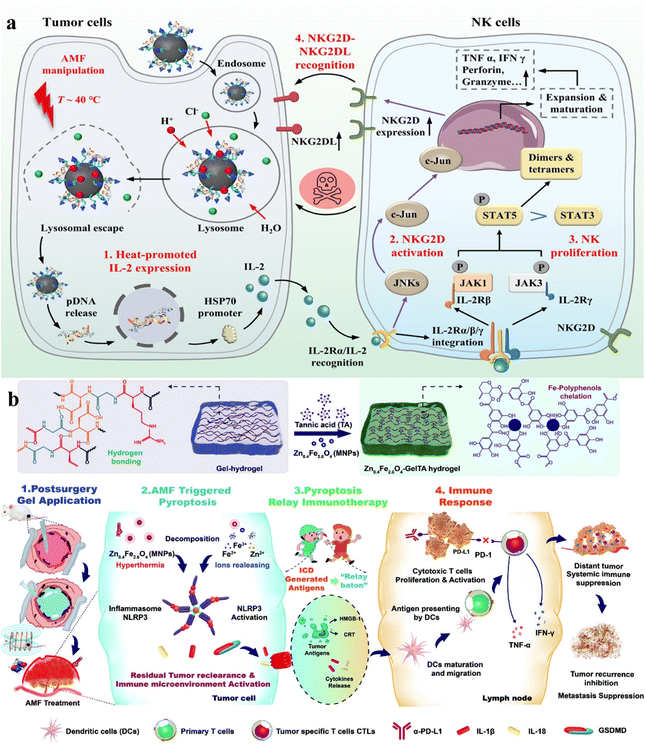 |
| Fig. 11 (a) Schematic representation of MPFD enhancing immune therapy for in situ liver cancer during magnetic hyperthermia treatment. (b) Schematic depiction of the GelTAMNPs nanomaterial activating immune response to suppress tumors during magnetic hyperthermia treatment. | |
Surgical treatment followed by chemotherapy is the primary management approach for bladder cancer, but the high surgical risk, chemotherapy toxicity, and high postoperative recurrence rate hinder its treatment. Shi and Yao groups first proposed a novel approach to treat bladder cancer using mild MHT via intravesical administration of magnetic nanoparticles.188 Core–shell Zn–CoFe2O4@Zn–MnFe2O4 (MNP) nanoparticles functionalized with hyaluronic acid were utilized for controlled mild magnetic hyperthermia (MNPHA) achieving temperatures of 43–44 °C, effectively suppressing bladder tumor cell proliferation and tumor growth (Fig. 12a). This method also stimulated immune responses in macrophages, dendritic cells, and T cells, reversing the immunosuppressive milieu of bladder tumors and significantly decreasing tumor recurrence, highlighting its clinical potential in bladder cancer treatment.
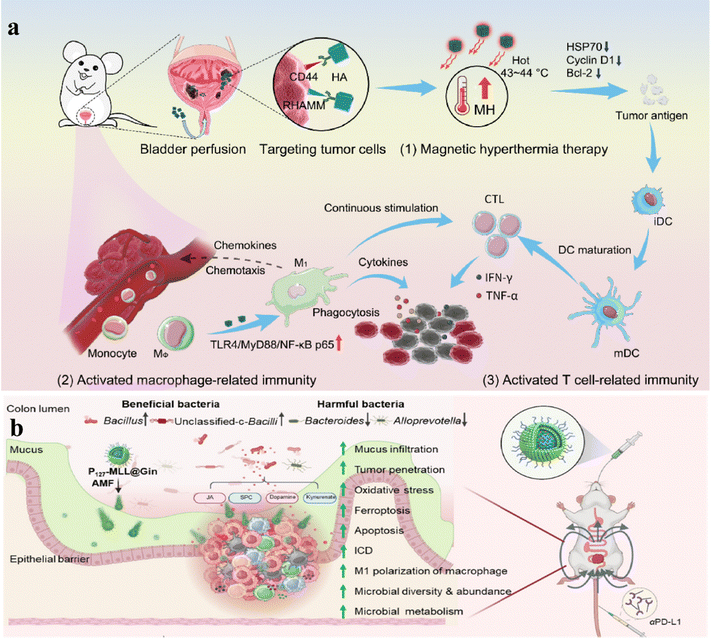 |
| Fig. 12 (a) Mechanism of MNP-HA enhancing immune therapy for primary bladder cancer during MHT. (b) Schematic illustration of MNP-HA targeting bladder cancer and immunotherapy in the mild magnetothermal therapy (MHT) state. | |
To address the therapeutic restrictions of oral nanoparticle-driven therapy for colorectal cancer (CRC), Xiao and Shi's team developed magnetically loaded mesoporous silica nanoparticles (MSNs) carrying 6-gingerol (Gin), functionalized with mulberry leaf extract lipids and Pluronic F127.189 Experimental research has demonstrated that P127 functionalization and alternating magnetic fields (AMFs) enhance the uptake of nanoparticles by colorectal cancer cells, triggering apoptosis/ferroptosis, stimulating anti-tumor immunity, and inhibiting tumor progression. (Fig. 12b). This therapeutic approach also improves gut microbiota balance and, when combined with checkpoint inhibitors, significantly enhances the efficacy of rectal cancer treatment and prolongs mouse survival.
3. Summary and future perspectives
This review concludes with utilizing biomaterials to enhance cancer immunotherapy through adjunct local chemotherapy, radiotherapy, phototherapy, sonodynamic therapy, and local application of immunomodulatory agents. Traditional local treatments primarily aim to directly kill cancer cells, but by leveraging immunomodulatory properties, they can provoke robust immune responses. Induction of ICD after local treatment can activate DCs by promoting cancer cells to release danger signals (e.g., antigens, calreticulin), thereby delivering antigenic material, which in turn causes immune cells (e.g., CD8+ T cells) to kill tumor cells. However, the presence of immunosuppressive factors suppresses the immune response, and local treatments alone may not sufficiently induce effective antitumor immune responses. Therefore, combining biomaterials with exogenous immune adjuvants or antagonists targeting various immune checkpoint receptors (such as ICB antibodies) in local delivery systems can achieve more potent antitumor immune responses. Numerous preclinical studies have demonstrated that such biomaterial-assisted local therapies can trigger systemic antitumor responses, attacking the remaining cancer cells and reducing the probability of tumour metastasis and recurrence. In addition, biomaterials are extensively explored for their investigational use in local immunomodulatory therapies. Local administration of biomaterials enables sustained and efficient release of immunotherapy, precisely targeting the location of the lesion, thus reducing various side effects associated with systemic treatments.
However, the clinical translation of biomaterial-based anti-tumor immunotherapies still faces some challenges. In animals, the permeability and retention (EPR) of biomaterial-based drugs targeting tumors via intravenous administration remain uncertain, and EPR effects in human tumors are controversial. Therefore, modifying targeted drugs on biomaterials may be a good strategy to solve the problem of tumor targeting. However, as biomaterials become more complex, uncontrollable factors also increase. Although intratumoral administration can solve the targeting problem, it is limited to local treatment, and the retention is still doubtful. At the same time, biomaterials that can trigger a systemic anti-tumor immune response can inhibit the development of distal tumors. At present, there are still many pathways based on tumor immune regulation that have not been developed. Therefore, research on biological material delivery mechanisms and tumor immunotherapy should be strengthened to build a mature system, so as to provide a diversified tumor treatment plan and thus provide a platform and opportunity for breakthroughs in tumor immunotherapy.
Data availability
No primary research results, software or code have been included. No new data were generated in this review.
Conflicts of interest
There are no conflicts to declare.
References
- R. L. Siegel, K. D. Miller, H. E. Fuchs and A. Jemal, Ca-Cancer J. Clin., 2022, 72, 7–33 CrossRef PubMed.
- S. Farkona, E. P. Diamandis and I. M. Blasutig, BMC Med., 2016, 14, 73 CrossRef PubMed.
- R. W. Jenkins, D. A. Barbie and K. T. Flaherty, Br. J. Cancer, 2018, 118, 9–16 CrossRef CAS.
- J. B. Haanen and C. Robert, Prog. Exp. Tumor Res., 2015, 42, 55–66 Search PubMed.
- A. W. Xiong, J. M. Fang, S. X. Ren, W. Li, J. Wang, Y. Zhao, G. Y. Chen, Q. Xu and C. C. Zhou, Front. Oncol., 2021, 11, 745699 CrossRef CAS PubMed.
- J. Kaur, J. Elms, A. L. Munn, D. Good and M. Q. Wei, Crit. Rev. Oncol. Hemat., 2021, 164, 103417 CrossRef PubMed.
- G. Z. Xie, H. Dong, Y. Liang, J. D. Ham, R. Rizwan and J. Z. Chen, EBioMedicine, 2020, 59, 102975 CrossRef CAS PubMed.
- S. L. Topalian, C. G. Drake and D. M. Pardoll, Cancer Cell, 2015, 27, 450–461 CrossRef CAS.
- M. Chehelgerdi, M. Chehelgerdi, O. Q. B. Allela, R. D. C. Pecho, N. Jayasankar, D. P. Rao, T. Thamaraikani, M. Vasanthan, P. Viktor, N. Lakshmaiya, M. J. Saadh, A. Amajd, M. A. Abo-Zaid, R. Y. Castillo-Acobo, A. H. Ismail, A. H. Amin and R. Akhavan-Sigari, Mol. Cancer, 2023, 22, 169 CrossRef PubMed.
- F. Marofi, R. Motavalli, V. A. Safonov, L. Thangavelu, A. V. Yumashev, M. Alexander, N. Shomali, M. S. Chartrand, Y. Pathak, M. Jarahian, S. Izadi, A. Hassanzadeh, N. Shirafkan, S. Tahmasebi and F. M. Khiavi, Stem Cell Res. Ther., 2021, 12, 81 CrossRef CAS PubMed.
- S. Z. Tana, D. P. Lib and X. Zhua, Biomed. Pharmacother., 2020, 124, 109821 CrossRef.
- J. Massague and A. C. Obenauf, Nature, 2016, 529, 298–306 CrossRef CAS PubMed.
- M. Pascual-Garcia, E. Bonfill-Teixidor, E. Planas-Rigol, C. Rubio-Perez, R. Iurlaro, A. Arias, I. Cuartas, A. Sala-Hojman, L. Escudero, F. Martinez-Ricarte, I. Huber-Ruano, P. Nuciforo, L. Pedrosa, C. Marques, I. Brana, E. Garralda, M. Vieito, M. Squatrito, E. Pineda, F. Graus, C. Espejo, J. Sahuquillo, J. Tabernero and J. Seoane, Nat. Commun., 2019, 10, 2416 CrossRef PubMed.
- J. L. Liang, G. F. Luo, W. H. Chen and X. Z. Zhang, Adv. Mater., 2021, 33, 2007630 CrossRef CAS PubMed.
- S. B. Son, J. H. Kim, X. W. Wang, C. L. Zhang, S. A. Yoon, J. Shin, A. Sharma, M. H. Lee, L. Cheng, J. S. Wu and J. S. Kim, Chem. Soc. Rev., 2020, 49, 3244–3261 RSC.
- M. W. Joo, S. Jin, G. J. Lee, Y. S. Lee and Y. G. Chung, Clin. Orthop. Surg., 2024, 16, 157–167 CrossRef PubMed.
- W. A. Lim and C. H. June, Cell, 2017, 168, 724–740 CrossRef CAS PubMed.
- N. G. Minutolo, E. E. Hollander and D. J. Powell, Jr., Front. Oncol., 2019, 9, 176 CrossRef.
- K. Newick, S. O'Brien, E. Moon and S. M. Albelda, Annu. Rev. Med., 2017, 68, 139–152 CrossRef CAS PubMed.
- S. Park, E. Shevlin, Y. Vedvyas, M. Zaman, S. Park, Y. S. Hsu, I. M. Min and M. M. Jin, Sci. Rep., 2017, 7, 14366 CrossRef PubMed.
- D. Meyran, J. J. Zhu, J. Butler, D. Tantalo, S. MacDonald, T. N. Nguyen, M. Wang, N. Thio, C. D’Souza, V. M. Qin, C. Slaney, A. Harrison, K. Sek, P. Petrone, K. Thia, L. Giuffrida, A. M. Scott, R. L. Terry, B. Tran, J. Desai, H. M. Prince, S. J. Harrison, P. A. Beavis, M. H. Kershaw, B. Solomon, P. G. Ekert, J. A. Trapani, P. K. Darcy and P. J. Neeson, Sci. Transl. Med., 2023, 15, eabk1900 CrossRef CAS PubMed.
- Y. Chao and Z. Liu, Nat. Rev. Bioeng., 2023, 1, 125–138 CrossRef.
- C. Fischbach, R. Chen, T. Matsumoto, T. Schmelzle, J. S. Brugge, P. J. Polverini and D. J. Mooney, Nat. Methods, 2007, 4, 855–860 CrossRef CAS PubMed.
- Y. C. Xu, H. Q. Lai, S. Y. Pan, L. L. Pan, T. Liu, Z. Y. Yang, T. F. Chen and X. Q. Zhu, Biomaterials, 2024, 305, 122452 CrossRef CAS PubMed.
- L. Jeanbart and M. A. Swartz, Proc. Natl. Acad. Sci. U. S. A., 2015, 112, 14467–14472 CrossRef CAS PubMed.
- H. P. Kok, E. N. K. Cressman, W. Ceelen, C. L. Brace, R. Ivkov, H. Grull, G. Ter Haar, P. Wust and J. Crezee, Int. J. Hyperthermia, 2020, 37, 711–741 CrossRef CAS PubMed.
- R. Kuai, L. J. Ochyl, K. S. Bahjat, A. Schwendeman and J. J. Moon, Nat. Mater., 2017, 16, 489–496 CrossRef CAS PubMed.
- D. G. Leach, S. Young and J. D. Hartgerink, Acta Biomater., 2019, 88, 15–31 CrossRef CAS PubMed.
- H. J. Zhao, Q. L. Song, C. X. Zheng, B. B. Zhao, L. X. Wu, Q. H. Feng, Z. Z. Zhang and L. Wang, Adv. Funct. Mater., 2020, 30, 2005747 CrossRef CAS.
- Q. Chen, C. Wang, X. D. Zhang, G. J. Chen, Q. Y. Hu, H. J. Li, J. Q. Wang, D. Wen, Y. Q. Zhang, Y. F. Lu, G. Yang, C. Jiang, J. Wang, G. Dotti and Z. Gu, Nat. Nanotechnol., 2019, 14, 89–97 Search PubMed.
- J. Wang, Y. Dong, Y. W. Li, W. Li, K. Cheng, Y. Qian, G. Q. Xu, X. S. Zhang, L. Hu, P. Chen, W. Du, X. J. Feng, Y. D. Zhao, Z. H. Zhang and B. F. Liu, Adv. Funct. Mater., 2018, 28, 1707360 CrossRef.
- L. Qin, J. Cao, K. Shao, F. Tong, Z. H. Yang, T. Lei, Y. Z. Wang, C. Hu, C. S. Umeshappa, H. L. Gao and N. A. Peppas, Sci. Adv., 2020, 6, eabb3116 CrossRef CAS PubMed.
- N. Eckman, A. Nejatfard, R. Cavet, A. K. Grosskopf and E. A. Appel, Nat. Rev. Bioeng., 2024, 2, 408–424 Search PubMed.
- H. Xu, D. Kim, Y. Y. Zhao, C. Kim, G. Song, Q. Hu, H. Kang and J. Yoon, Adv. Mater., 2024, 36, 2402806 Search PubMed.
- J. Lv, R. Yue, H. Liu, H. Du, C. Lu, C. Zhang, G. Guan, S. Min, S. Huan, H. Kang and G. Song, Coord. Chem. Rev., 2024, 510, 215842 Search PubMed.
- M. Sylvestre, C. A. Crane and S. H. Pun, Adv. Mater., 2020, 32, 1902007 CrossRef CAS.
- H. Shen, S. Liao, Z. Li, Y. Wang, S. Huan, X. B. Zhang and G. Song, Chemistry, 2023, 29, 202301209 CrossRef.
- C. Y. Ang, S. Y. Tan and Y. Zhao, Org. Biomol. Chem., 2014, 12, 4776–4806 RSC.
- D. S. Chen and I. Mellman, Nature, 2017, 541, 321–330 CrossRef CAS PubMed.
- I. Mellman, G. Coukos and G. Dranoff, Nature, 2011, 480, 480–489 CrossRef CAS PubMed.
- K. J. Curran, H. J. Pegram and R. J. Brentjens, J. Gene Med., 2012, 14, 405–415 CrossRef CAS.
- R. S. Riley, C. H. June, R. Langer and M. J. Mitchell, Nat. Rev. Drug Discovery, 2019, 18, 175–196 CrossRef CAS PubMed.
- P. S. Hegde and D. S. Chen, Immunity, 2020, 52, 17–35 CrossRef CAS.
- R. Nagareddy, R. G. Thomas and Y. Y. Jeong, Int. J. Mol. Sci., 2021, 22, 12510 CrossRef CAS.
- W. W. Mu, Q. H. Chu, Y. J. Liu and N. Zhang, Nano-Micro Lett., 2020, 12, 142 CrossRef CAS PubMed.
- D. Y. Lee, K. Huntoon, Y. F. Wang, W. Jiang and B. Y. S. Kim, Adv. Mater., 2021, 33, 2007576 CrossRef CAS PubMed.
- H. Zhou, C. Y. Liang, Z. Wei, Y. J. Bai, S. B. Bhaduri, T. J. Webster, L. M. Bian and L. Yang, Mater. Today, 2019, 28, 81–97 CrossRef CAS.
- Y. Q. Li, X. L. Liu, L. Yu, X. Huang, X. Wang, D. Han, Y. Yang and Z. Liu, J. Am. Chem. Soc., 2023, 145, 24506–24521 CAS.
- A. Perez-Lopez, C. Martin-Sabroso, L. Gomez-Lazaro, A. I. Torres-Suarez and J. Aparicio-Blanco, Acta Biomater., 2022, 149, 1–15 CrossRef CAS PubMed.
- W. Chen, F. H. Meng, R. Cheng and Z. Y. Zhong, J. Controlled Release, 2010, 142, 40–46 CrossRef CAS PubMed.
- W. Park, H. Shin, B. Choi, W.-K. Rhim, K. Na and D. Keun Han, Prog. Mater. Sci., 2020, 114, 100686 CrossRef CAS.
- Y. Bo and H. Wang, Adv. Mater., 2023, 2210452 Search PubMed.
- S. X. Lv, Z. H. Tang, M. Q. Li, J. Lin, W. T. Song, H. Y. Liu, Y. B. Huang, Y. Y. Zhang and X. S. Chen, Biomaterials, 2014, 35, 6118–6129 CrossRef CAS PubMed.
- H. Dong, Q. Li, Y. Zhang, M. Ding, Z. G. Teng and Y. B. Mou, Adv. Sci., 2023, 10, 2301339 CrossRef CAS PubMed.
- H. Y. Tian, Z. H. Tang, X. L. Zhuang, X. S. Chen and X. B. Jing, Prog. Polym. Sci., 2012, 37, 237–280 CrossRef CAS.
- G. F. Luo, W. H. Chen, X. Zeng and X. Z. Zhang, Chem. Soc. Rev., 2021, 50, 945–985 RSC.
- P. Tarantino, R. Carmagnani Pestana, C. Corti, S. Modi, A. Bardia, S. M. Tolaney, J. Cortes, J. C. Soria and G. Curigliano, Ca-Cancer J. Clin., 2022, 72, 165–182 CrossRef.
- R. X. He, P. D. Yang, A. X. Liu, Y. L. Zhang, Y. Q. Chen, C. Chang and B. Lu, J. Mater. Chem. B, 2023, 11, 9798–9839 RSC.
- L. X. Zhang, C. J. Zhu, J. Zhao, L. C. Scimeca, M. D. Dong, R. T. Liu, Y. B. Jia and Z. P. Xu, Adv. Funct. Mater., 2024, 34, 2311914 CrossRef CAS.
- H. Wang, A. J. Najibi, M. C. Sobral, B. R. Seo, J. Y. Lee, D. Wu, A. W. Li, C. S. Verbeke and D. J. Mooney, Nat. Commun., 2020, 11, 5696 CrossRef CAS.
- S. B. Yong, S. Ramishetti, M. Goldsmith, Y. Diesendruck, I. Hazan-Halevy, S. Chatterjee, G. Somu Naidu, A. Ezra and D. Peer, Adv. Mater., 2022, 34, 2106350 CrossRef CAS PubMed.
- Z. Y. Han, C. Zhang, J. X. An, Y. Z. Wang, J. Y. Qiao, X. Zeng and X. Z. Zhang, Adv. Funct. Mater., 2023, 33, 2302728 CrossRef CAS.
- J. J. Xiang, K. X. Liu, H. X. Xu, Z. H. Zhao, Y. Piao, S. Q. Shao, J. B. Tang, Y. Q. Shen and Z. X. Zhou, Adv. Sci., 2023, 10, 2301216 CrossRef CAS PubMed.
- Y. H. Wang, L. M. Xie, X. X. Li, L. Wang and Z. M. Yang, Bioact. Mater., 2024, 31, 549–562 CAS.
- K. C. Mei, Y. P. Liao, J. Jiang, M. Chiang, M. Khazaieli, X. Liu, X. Wang, Q. Liu, C. H. Chang, X. Zhang, J. Li, Y. Ji, B. Melano, D. Telesca, T. Xia, H. Meng and A. E. Nel, ACS Nano, 2020, 14, 13343–13366 CrossRef CAS PubMed.
- J. W. Cui, X. Wang, J. G. Li, A. R. Zhu, Y. J. Du, W. Zeng, Y. M. Guo, L. Q. Di and R. N. Wang, ACS Nano, 2023, 17, 1464–1484 CrossRef CAS PubMed.
- J. Liu, Z. H. Zhao, N. S. Qiu, Q. Zhou, G. W. Wang, H. P. Jiang, Y. Piao, Z. X. Zhou, J. B. Tang and Y. Q. Shen, Nat. Commun., 2021, 12, 2425 CrossRef CAS.
- P. Pei, Y. Zhang, Y. C. Jiang, W. H. Shen, H. Chen, S. Yang, Y. X. Zhang, X. Yi and K. Yang, ACS Nano, 2022, 16, 11325–11337 CrossRef CAS PubMed.
- B. Colak and Y. N. Ertas, ACS Appl. Mater. Interfaces, 2024, 16, 15718–15729 CrossRef CAS.
- S. Yasmin-Karim, B. Ziberi, J. Wirtz, N. Bih, M. Moreau, R. Guthier, V. Ainsworth, J. Hesser, G. M. Makrigiorgos, M. D. Chuong, X. Wei, P. L. Nguyen and W. Ngwa, Int. J. Radiat. Oncol., Biol., Phys., 2022, 112, 475–486 CrossRef PubMed.
- Y. Yang, B. Liu, Y. Liu, J. Q. Chen, Y. J. Sun, X. S. Pan, J. Xu, S. J. Xu, Z. Liu and W. H. Tan, Nano Lett., 2022, 22, 2826–2834 CrossRef CAS PubMed.
- Y. W. Jiang, G. Gao, H. R. Jia, X. Zhang, X. Cheng, H. Y. Wang, P. Liu and F. G. Wu, Langmuir, 2020, 36, 11637–11644 CrossRef CAS PubMed.
- D. G. Leach, N. Dharmaraj, T. L. Lopez-Silva, J. R. Venzor, B. H. Pogostin, A. G. Sikora, J. D. Hartgerink and S. Young, ACS Biomater. Sci. Eng., 2021, 7, 415–421 CrossRef CAS PubMed.
- F. Castro, M. L. Pinto, C. L. Pereira, K. Serre, M. A. Barbosa, K. Vermaelen, F. Gartner, R. M. Goncalves, O. De Wever and M. J. Oliveira, Biomaterials, 2020, 257, 120218 CrossRef CAS.
- W. Huang, L. Z. He, J. Ouyang, Q. Chen, C. Liu, W. Tao and T. F. Chen, Matter, 2020, 3, 1725–1753 CrossRef.
- M. Wang, Y. Li, M. Wang, K. L. Liu, A. R. Hoover, M. Li, R. A. Towner, P. Mukherjee, F. F. Zhou, J. L. Qu and W. R. Chen, Acta Biomater., 2022, 138, 453–462 CrossRef CAS.
- K. Y. Liu, Y. X. Liao, Z. F. Zhou, L. Zhang, Y. Y. Jiang, H. L. Lu, T. Y. Xu, D. Yang, Q. M. Gao, Z. H. Li, S. Tan, W. T. Cao, F. Chen and G. D. Li, Biomaterials, 2022, 282, 121383 CrossRef CAS PubMed.
- W. D. Ni, J. Y. Wu, H. P. Fang, Y. J. Feng, Y. Y. Hu, L. Lin, J. Chen, F. F. Chen and H. Y. Tian, Nano Lett., 2021, 21, 7796–7805 CrossRef CAS PubMed.
- Y. Duan, H. Yang, J. X. Gao, D. H. Wei, Y. Zhang, J. Wang, X. H. Zhang, J. C. Zhang, K. Ge, X. L. Wu and J. Chang, ACS Appl. Bio Mater., 2021, 4, 1524–1535 CrossRef CAS.
- D. Y. Xu, J. Liu, Y. X. Wang, Y. Y. Jian, W. W. Wu and R. C. Lv, ACS Biomater. Sci. Eng., 2020, 6, 4940–4948 CrossRef CAS PubMed.
- I. Noh, Y. J. Son, W. Jung, D. Kim, H. Shin, Y.-C. Kim and S. Jon, Biomaterials, 2021, 275, 120926 CrossRef CAS PubMed.
- B. Q. Zhou, Q. Wu, M. Wang, A. Hoover, X. Wang, F. F. Zhou, R. A. Towner, N. Smith, D. Saunders, J. Song, J. L. Qu and W. R. Chen, Chem. Eng. J., 2020, 396, 125239 CrossRef CAS.
- X. Sun, Z. Y. Cao, K. R. Mao, C. X. Wu, H. M. Chen, J. L. Wang, X. Wang, X. X. Cong, Y. Li, X. Y. Meng, X. Z. Yang, Y. G. Yang and T. M. Sun, Biomaterials, 2020, 240, 119845 CrossRef CAS PubMed.
- G. Shu, W. Zhu, Y. Z. Jiang, X. W. Li, J. B. Pan, X. N. Zhang, X. J. Zhang and S. K. Sun, Adv. Funct. Mater., 2021, 31, 2104472 CrossRef CAS.
- C. H. Chung, K. Y. Lu, W. C. Lee, W. J. Hsu, W. F. Lee, J. Z. Dai, P. W. Shueng, C. W. Lin and F. L. Mi, Biomaterials, 2020, 257, 120227 CrossRef CAS PubMed.
- Y. Zhang, X. Y. Du, S. G. Liu, H. X. Yan, J. B. Ji, Y. W. Xi, X. Y. Yang and G. G. Zhai, Biomaterials, 2021, 278, 121135 CrossRef CAS PubMed.
- L. M. Zhang, K. Wang, Y. H. Huang, H. Zhang, L. Zhou, A. Li and Y. Y. Sun, Biomaterials, 2022, 282, 121411 CrossRef CAS PubMed.
- H. Y. Huang, W. S. Xie, D. N. Hu, X. Z. He, R. X. Li, X. Y. Zhang and Y. Wei, Chem. Eng. J., 2023, 451, 138617 CrossRef CAS.
- C. L. Yang, Y. Y. Fu, C. Huang, D. R. Hu, K. Zhou, Y. Hao, B. Y. Chu, Y. Yang and Z. Y. Qian, Biomaterials, 2020, 255, 120194 CrossRef CAS PubMed.
- H. J. Wang, K. Wang, L. H. He, Y. Liu, H. Q. Dong and Y. Y. Li, Biomaterials, 2020, 244, 119964 CrossRef CAS PubMed.
- J. S. Huang, Z. C. Xiao, Y. C. An, S. S. Han, W. Wud, Y. Wang, Y. Guo and X. T. Shuai, Biomaterials, 2021, 269, 120636 CrossRef CAS PubMed.
- H. Q. Chen, L. L. Liu, A. Q. Ma, T. Yin, Z. Chen, R. J. Liang, Y. Z. Qiu, M. B. Zheng and L. T. Cai, Biomaterials, 2021, 269, 120639 CrossRef CAS PubMed.
- H. Tian, G. H. Wang, W. Sang, L. S. Xie, Z. Zhang, W. X. Li, J. Yan, Y. Tian, J. Li, B. Li and Y. L. Dai, Nano Today, 2022, 43, 101405 CrossRef CAS.
- J. Y. Wu, J. S. Huang, J. Yu, M. K. Xu, J. Liu and K. Y. Pu, Adv. Mater., 2024, 2400762 CrossRef CAS.
- J. F. Chen, L. T. Feng, P. L. Jin, J. X. Shen, J. Y. Lu, Y. Song, G. W. Wang, Q. Chen, D. Y. Huang, Y. Zhang, C. Zhang, Y. F. Xu and P. T. Huang, J. Nanobiotechnol., 2022, 20, 283 CrossRef CAS PubMed.
- J. L. Luo, X. Wang, Z. Shi, Y. Q. Zeng, L. C. He, J. Cao, Y. Sun, T. Zhang and P. T. Huang, J. Nanobiotechnol., 2022, 20, 228 CrossRef CAS.
- W. J. Zhu, Q. Chen, Q. T. Jin, Y. Chao, L. L. Sun, X. Han, J. Xu, L. L. Tian, J. L. Zhang, T. Liu and Z. Liu, Nano Res., 2020, 14, 212–221 CrossRef.
- N. L. Yang, X. W. Sun, Y. K. Zhou, X. Y. Yang, J. X. You, Z. P. Yu, J. Ge, F. Gong, Z. S. Xiao, Y. Jin, Z. Liu and L. Cheng, Sci. Bull., 2023, 68, 1772–1783 CrossRef CAS.
- Q. Shi, W. Zhang, Y. J. Zhou, S. J. Huang, J. Yu, M. J. Yang, Z. H. Zhang, J. Q. Ma, J. J. Luo, S. X. Rao, D. R. Lu, S. J. Peng, Y. B. Cao, L. X. Liu and Z. P. Yan, Biomaterials, 2024, 306, 122480 CrossRef CAS.
- Z. L. Dong, L. Zhang, D. X. Zhao, C. J. Wang, Y. Hao, Q. G. Li, Y. Y. Zhang, Z. J. Yang, C. F. Ni, Z. Liu and L. Z. Feng, Nano Today, 2024, 55, 102216 CrossRef CAS.
- C. Y. Wang, L. P. Zhong, R. Peng, J. C. Xu, X. J. Chen, H. Q. Tao, C. Hu, J. Y. Li, Q. Zhao, X. J. Sun, Z. Liu, Y. Huo, Q. Zhuang, F. Q. Huang, F. Gong, N. L. Yang, Q. Chen and Y. X. Zhao, Nat. Biomed. Eng., 2024, 8, 561–578 CrossRef CAS PubMed.
- J. O. Pan, Y. Y. Xu, Q. S. Wu, P. Hu and J. L. Shi, J. Am. Chem. Soc., 2021, 143, 8116–8128 CrossRef CAS PubMed.
- K.-Y. Qian, Y. H. Song, X. Yan, L. Dong, J. Z. Xue, Y. J. Xu, B. Wang, B. Q. Cao, Q. B. Hou, W. Peng, J. L. Hu, K. Jiang, S. Chen, H. Q. Wang and Y. Lu, Biomaterials, 2020, 259, 120299 CrossRef CAS PubMed.
- I. Shena, T. W. Reesa, Z. G. Zhoub, S. P. Yangb, L. N. Jia and H. Chaoa, Biomaterials, 2020, 251, 120079 CrossRef.
- J. Pan, P. Hu, Y. D. Guo, J. N. Hao, D. L. Ni, Y. Y. Xu, Q. Q. Bao, H. L. Yao, C. Y. Wei, Q. S. Wu and J. L. Shi, ACS Nano, 2020, 14, 1033–1044 CrossRef CAS PubMed.
- S. Y. Wang, Y. S. Jiang, Z. P. Qian, L. L. Ren, J. H. Wang, Y. W. Liu, Y. C. Li, J. Li, K. M. Qu, F. Wang, H. A. Wu, F. Yang, Y. Zhang, Y. Gao and L. H. Wang, Chem. Eng. J., 2024, 488, 151072 CrossRef CAS.
- A. M. M. Dias, A. Courteau, P. S. Bellaye, E. Kohli, A. Oudot, P. E. Doulain, C. Petitot, P. M. Walker, R. Decreau and B. Collin, Pharmaceutics, 2022, 14, 2388 CrossRef CAS PubMed.
- L. Z. Chen, W. W. Ren, Q. Q. Bao, P. Hu, W. M. Fang, T. Wu and J. L. Shi, Nano Today, 2024, 56, 102284 CrossRef CAS.
- G. Guan, H. Liu, J. Xu, Q. Zhang, Z. Dong, L. Lei, C. Zhang, R. Yue, H. Gao, G. Song and X. Shen, J. Nanobiotechnol., 2023, 21, 434 CrossRef CAS.
- C. Lu, Z. Li, N. Wu, D. Lu, X.-B. Zhang and G. Song, Chem, 2023, 9, 3185–3211 CAS.
- N. Momin, N. Mehta, N. Bennett, L. Ma, J. Palmeri, M. Chinn, E. Lutz, B. Kang, D. Irvine and S. Spranger, Sci. Transl. Med., 2019, 498, 483–484 Search PubMed.
- A. K. Jangid, S. Kim and K. Kim, Biomater. Res., 2023, 27, 59 CrossRef CAS.
- K. Adu-Berchie and D. J. Mooney, Acc. Chem. Res., 2020, 53, 1749–1760 CrossRef CAS.
- J. M. Karp and R. Langer, Curr. Opin. Biotechnol, 2007, 18, 454–459 CrossRef CAS PubMed.
- B. He, X. Sui, B. Yu, S. Wang, Y. Shen and H. Cong, Drug Delivery, 2020, 27, 1474–1490 CrossRef CAS.
- A. Bigham, V. Rahimkhoei, P. Abasian, M. Delfi, J. Naderi, M. Ghomi, F. Dabbagh Moghaddam, T. Waqar, Y. Nuri Ertas, S. Sharifi, N. Rabiee, S. Ersoy, A. Maleki, E. Nazarzadeh Zare, E. Sharifi, E. Jabbari, P. Makvandi and A. Akbari, Chem. Eng. J., 2022, 432, 134146 CrossRef CAS.
- P. F. Chiang, C. L. Peng, Y. H. Shih, Y. H. Cho, C. S. Yu, Y. M. Kuo, M. J. Shieh and T. Y. Luo, ACS Biomater. Sci. Eng., 2018, 4, 3425–3433 CrossRef CAS.
- A. Athenodorou, H. Panagopoulos and A. Tsapalis, Phys. Lett. B, 2008, 659, 252–259 CrossRef CAS.
- H. W. Liao, S. F. Yang, Z. Y. Liang, L. Xiao, S. Z. Xie, P. H. Lin, F. Xia, C. Y. Fang, Q. Chen, D. S. Ling and F. Y. Li, ACS Nano, 2023, 17, 18548–18561 CrossRef CAS PubMed.
- W. M. C. van den Boogaard, D. S. J. Komninos and W. P. Vermeij, Cancers, 2022, 14, 627 CrossRef CAS PubMed.
- Brianna and S. H. Lee, Med. Oncol., 2023, 40, 88 CrossRef CAS.
- G. Q. Wei, Y. Wang, G. Yang, Y. Wang and R. Ju, Theranostics, 2021, 11, 6370–6392 CrossRef CAS PubMed.
- L. L. Xue, A. S. Thatte, D. Mai, R. M. Haley, N. Q. Gong, X. X. Han, K. Wang, N. C. Sheppard, C. H. June and M. J. Mitchell, Nat. Rev. Mater., 2023, 9, 100–118 CrossRef.
- N. N. Shah and T. J. Fry, Nat. Rev. Clin. Oncol., 2019, 16, 372–385 CAS.
- L. Lei, F. Yang, X. Meng, L. Xu, P. Liang, Y. Ma, Z. Dong, Y. Wang, X. B. Zhang and G. Song, J. Am. Chem. Soc., 2023, 145, 24386–24400 CrossRef CAS PubMed.
- Y. Wang, L. Shi, Z. Ye, K. Guan, L. Teng, J. Wu, X. Yin, G. Song and X.-B. Zhang, Nano Lett., 2019, 20, 176–183 CrossRef PubMed.
- B. J. Umlauf, G. Frampton, A. Cooper and H. F. Greene, J. Controlled Release, 2023, 364, 195–205 CrossRef CAS PubMed.
- A. M. Aklilu and A. C. Shirali, Kidney360, 2023, 4, 409–422 CrossRef PubMed.
- S. P. Jiang, W. P. Li, J. Yang, T. Zhang, Y. Q. Zhang, L. Xu, B. Hu, Z. Li, H. L. Gao, Y. Y. Huang and S. B. Ruan, ACS Nano, 2024, 18, 6445–6462 CrossRef CAS PubMed.
- J. L. Lv, Y. J. Xu, Y. Liu, K. Z. Sakurai, H. Y. Yu and Z. H. Tang, Biomaterials, 2024, 309, 122586 CrossRef CAS PubMed.
- Y. Chao, L. G. Xu, C. Liang, L. Z. Feng, J. Xu, Z. L. Dong, L. L. Tian, X. Yi, K. Yang and Z. Liu, Nat. Biomed. Eng., 2018, 2, 611–621 CrossRef CAS PubMed.
- N. H. Liu, J. J. Zhu, W. J. Zhu, L. F. Chen, M. Y. Li, J. J. Shen, M. C. Chen, Y. M. Wu, F. Pan, Z. Deng, Y. Liu, G. B. Yang, Z. Liu, Q. Chen and Y. Yang, Adv. Mater., 2023, 35, 2302220 CrossRef CAS PubMed.
- S. Y. Pan, Z. W. Sun, B. Zhao, L. Q. Miao, Q. F. Zhou, T. F. Chen and X. Q. Zhu, Biomaterials, 2023, 302, 122321 CrossRef CAS PubMed.
- G. Petroni, L. C. Cantley, L. Santambrogio, S. C. Formenti and L. Galluzzi, Nat. Rev. Clin. Oncol., 2022, 19, 114–131 CrossRef CAS PubMed.
- L. Hovhannisyan, C. Riether, D. M. Aebersold, M. Medova and Y. Zimmer, Mol. Cancer, 2023, 22, 82 CrossRef PubMed.
- C. Galassi, V. Klapp, T. Yamazaki and L. Galluzzi, Immunol. Rev., 2024, 321, 20–32 CrossRef CAS PubMed.
- T. Liu, P. Pei, W. H. Shen, L. Hu and K. Yang, Small Methods, 2023, 7, 2201401 CrossRef CAS PubMed.
- C. He, H. Y. Ding, L. B. Li, J. Chen, X. F. Mo, Y. N. Ding, W. J. Chen, Q. S. Tang and Y. T. Wang, Int. J. Nanomed., 2023, 18, 5701–5712 CrossRef CAS PubMed.
- H. J. Luo, H. M. Cao, H. X. Jia, Y. N. Shang, J. J. Liu, H. Gui, C. H. Yang, C. H. Ren, Z. Y. Wang and J. F. Liu, Adv. Healthcare Mater., 2023, 12, 2301083 CrossRef CAS PubMed.
- L. L. Sun, F. Y. Shen, L. L. Tian, H. Q. Tao, Z. J. Xiong, J. Xu and Z. Liu, Adv. Mater., 2021, 33, 2007910 CrossRef CAS PubMed.
- Y. Z. Min, K. C. Roche, S. M. Tian, M. J. Eblan, K. P. McKinnon, J. M. Caster, S. J. Chai, L. E. Herring, L. Z. Zhang, T. Zhang, J. M. DeSimone, J. E. Tepper, B. G. Vincent, J. S. Serody and A. Z. Wang, Nat. Nanotechnol., 2017, 12, 877–882 CrossRef CAS PubMed.
- L. L. Tian, Y. X. Wang, L. L. Sun, J. Xu, Y. Chao, K. Yang, S. A. Wang and Z. Liu, Matter, 2019, 1, 1061–1076 CrossRef.
- J. X. Gao, Q. H. Wu, Y. F. Yan, M. M. Chen, Q. G. Li, Y. C. Xu, C. J. Wang, E. H. Hao, Z. Liu and L. Z. Feng, Adv. Funct. Mater., 2024, 2401830 CrossRef CAS.
- H. R. Wang, Y. Xie, Y. L. Chen, H. Zhao, X. J. Lv, Z. M. Zhang, G. Li, J. Pan, J. Wang and Z. Liu, Adv. Healthcare Mater., 2023, 12, 2300848 CrossRef CAS PubMed.
- M. Guo, H. J. Mao, Y. L. Li, A. J. Zhu, H. He, H. Yang, Y. Y. Wang, X. Tian, C. C. Ge, Q. L. Peng, X. Y. Wang, X. L. Yang, X. Y. Chen, G. Liu and H. B. Chen, Biomaterials, 2014, 35, 4656–4666 CrossRef CAS PubMed.
- B. Nasseri, E. Alizadeh, F. Bani, S. Davaran, A. Akbarzadeh, N. Rabiee, A. Bahadori, M. Ziaei, M. Bagherzadeh, M. R. Saeb, M. Mozafari and M. R. Hamblin, Appl. Phys. Rev., 2022, 9, 011317 CAS.
- S. S. Sun, J. Q. Chen, K. Jiang, Z. D. Tang, Y. H. Wang, Z. J. Li, C. B. Liu, A. G. Wu and H. W. Lin, ACS Appl. Mater. Interfaces, 2019, 11, 5791–5803 CrossRef CAS PubMed.
- Y. J. Wang, N. Q. Gong, Y. J. Li, Q. C. Lu, X. Wang and J. H. Li, J. Am. Chem. Soc., 2019, 142, 1735–1739 CrossRef PubMed.
- M. Overchuk, R. A. Weersink, B. C. Wilson and G. Zheng, ACS Nano, 2023, 17, 7979–8003 CrossRef CAS PubMed.
- Y. Wang, L. L. Chang, H. Y. Gao, C. H. Yu, Y. J. Gao and Q. Peng, Eur. J. Med. Chem., 2024, 272, 116508 CrossRef CAS PubMed.
- Y. J. Hou, X. X. Yang, R. Q. Liu, D. Zhao, C. X. Guo, A. C. Zhu, M. N. Wen, Z. Liu, G. F. Qu and H. X. Meng, Int. J. Nanomed., 2020, 15, 6827–6838 CrossRef CAS PubMed.
- S. Lee, J. Yoo, G. Bae, R. Thangam, J. Heo, J. Y. Park, H. Choi, C. Kim, J. An, J. Kim, K. R. Mun, S. Shin, K. Zhang, P. Zhao, Y. Kim, N. Kang, S.-B. Han, D. Kim, J. Yoon, M. Kang, J. Kim, L. Yang, S. Karamikamkar, J. Kim, Y. Zhu, A. H. Najafabadi, G. Song, D.-H. Kim, K.-B. Lee, S. J. Oh, H.-D. Jung, H.-C. Song, W. Y. Jang, L. Bian, Z. Chu, J. Yoon, J. S. Kim, Y. S. Zhang, Y. Kim, H. S. Jang, S. Kim and H. Kang, Bioact. Mater., 2024, 34, 164–180 CAS.
- G. Gao, X. B. Sun and G. L. Liang, Adv. Funct. Mater., 2021, 31, 2100738 CrossRef CAS.
- S. P. Feng, J. Y. Lu, K. L. Wang, D. H. Di, Z. N. Shi, Q. F. Zhao and S. L. Wang, Chem. Eng. J., 2022, 435, 134886 CrossRef CAS.
- C. L. Huang, H. Y. Wang, X. Y. Yang, Q. Y. Yu, H. Wang, L. H. Zhang, Y. L. Zhao and D. W. Zhu, Adv. Funct. Mater., 2024, 2401489 CrossRef CAS.
- M. M. Liu, D. Jin, W. X. Yu, J. J. Yu, K. M. Cao, J. J. Cheng, X. H. Zheng, A. Wang and Y. Z. Liu, Adv. Sci., 2024, 11, 2308248 CrossRef CAS PubMed.
- J. R. Wang, W. L. Zhang, Z. Y. Xie, X. Y. Wang, J. S. Sun, F. Ran, W. X. Jiang, Y. Liu, Z. G. Wang, H. T. Ran and D. J. Guo, Biomaterials, 2024, 308, 122570 CrossRef CAS PubMed.
- J. Choi, M. K. Shim, S. Yang, H. S. Hwang, H. Cho, J. Kim, W. S. Yun, Y. Moon, J. Kim, H. Y. Yoon and K. Kim, ACS Nano, 2021, 15, 12086–12098 CrossRef CAS PubMed.
- J. R. Du, T. Jia, F. Li, Y. Li, Q. H. Wang, L. C. He, H. Agren and G. Y. Chen, Adv. Funct. Mater., 2024, 2401272 CrossRef CAS.
- L. F. Fan, C. J. Jian, L. Li, L. Wang, X. J. Song, M. J. Wang, C. T. Liu, X. X. Liu, S. D. Xu, X. H. Gao, X. J. Shi, M. M. Yuan, B. Liu and L. L. Shi, Adv. Funct. Mater., 2023, 34, 2313755 CrossRef.
- M. Zhao, Y. Y. Zhang, J. Miao, H. Zhou, Y. Jiang, Y. Zhang, M. Q. Miao, W. Chen, W. Xing, Q. Li and Q. Q. Miao, Adv. Mater., 2024, 36, 2305243 CrossRef CAS PubMed.
- X. S. Li, J. F. Lovell, J. Y. Yoon and X. Y. Chen, Nat. Rev. Clin. Oncol., 2020, 17, 657–674 CrossRef PubMed.
- X. Y. Deng, Z. W. Shao and Y. L. Zhao, Adv. Sci., 2021, 8, 2002504 CrossRef CAS PubMed.
- R. Chen, X. Wang, X. K. Yao, X. C. Zheng, J. Wang and X. Q. Jiang, Biomaterials, 2013, 34, 8314–8322 CrossRef CAS PubMed.
- S. S. Ren, X. Cheng, M. K. Chen, C. Liu, P. C. Zhao, W. Huang, J. He, Z. Y. Zhou and L. Y. Miao, ACS Appl. Mater. Interfaces, 2017, 9, 31509–31518 CrossRef CAS PubMed.
- Y. P. Chen, R. Y. Lyu, J. Wang, Q. C. Cheng, Y. F. Yu, S. X. Yang, C. B. Mao and M. Y. Yang, Adv. Sci., 2023, 10, 2302700 CrossRef CAS PubMed.
- Y. Li, Z. K. Du, Y. Zhang, X. Y. Kang, J. W. Song, X. D. Chen, Y. B. Hu, Z. M. Yang, J. Qi and X. Shen, Adv. Funct. Mater., 2024, 34, 2315127 CrossRef CAS.
- C. Q. Kong, B. H. Xu, G. H. Qiu, M. Wei, M. Q. Zhang, S. X. Bao, J. L. Tang, L. Q. Li and J. J. Liu, Int. J. Nanomed., 2022, 17, 5391–5411 CrossRef CAS PubMed.
- V. S. Bachu, J. Kedda, I. Suk, J. J. Green and B. Tyler, Ann. Biomed. Eng., 2021, 49, 1975–1991 CrossRef PubMed.
- P. Yan, L. H. Liu and P. Wang, ACS Appl. Bio Mater., 2020, 3, 3456–3475 CrossRef CAS PubMed.
- N. Huebsch, C. J. Kearney, X. H. Zhao, J. Kim, C. A. Cezar, Z. Suo, Z. G. Suo and D. J. Mooney, Proc. Natl. Acad. Sci. U. S. A., 2014, 111, 9762–9767 CrossRef CAS PubMed.
- M. Kuroki, K. Hachimine, H. Abe, H. Shibaguchi, M. Kuroki, S.-I. Maekawa, J. Yanagisawa, T. Kinugasa, T. Tanaka and Y. Yamashita, Anticancer Res., 2007, 27, 3673–3677 CAS.
- W. W. Yue, L. Chen, L. D. Yu, B. G. Zhou, H. H. Yin, W. W. Ren, C. Liu, L. H. Guo, Y. F. Zhang, L. P. Sun, K. Zhang, H. X. Xu and Y. Chen, Nat. Commun., 2019, 10, 2025 CrossRef PubMed.
- Y. J. Wang, F. Gong, Z. H. Han, H. L. Lei, Y. K. Zhou, S. N. Cheng, X. Y. Yang, T. Y. Wang, L. Wang, N. L. Yang, Z. Liu and L. Cheng, Angew. Chem., Int. Ed., 2023, 62, 202215467 CrossRef PubMed.
- X. Y. Xu, S. Shabiti, X. Zhang, J. L. Zheng, N. Liang, Z. X. Wang, S. W. Yu, Y. J. Wang, S. Jiang, Z. Y. Pan, W. J. Li and L. T. Cai, Nano Today, 2024, 56, 102270 CrossRef CAS.
- Y. M. Wang, B. Lv, H. Wang, T. T. Ren, Q. Jiang, X. Y. Qu, D. L. Ni, J. J. Qiu and K. Q. Hua, ACS Nano, 2024, 18, 11042–11057 CrossRef CAS PubMed.
- A. Perez-Lopez, C. Martin-Sabroso, A. I. Torres-Suarez and J. Aparicio-Blanco, Pharmaceutics, 2020, 12, 1028 CrossRef CAS PubMed.
- K. Hatamaru, S. Azuma, T. Akamatsu, T. Seta, S. Urai, Y. Uenoyama, Y. Yamashita and K. Ono, World J. Gastroenterol., 2015, 21, 1344–1348 CrossRef PubMed.
- E. Ximendes, R. Marin, Y. L. Shen, D. Ruiz, D. Gómez-Cerezo, P. Rodríguez-Sevilla, J. Lifante, P. X. Viveros-Méndez, F. Gámez, D. García-Soriano, G. Salas, C. Zalbidea, A. Espinosa, A. Benayas, N. García-Carrillo, L. Cussó, M. Desco, F. J. Teran, B. H. Juárez and D. Jaque, Adv. Mater., 2021, 33, 2100077 CrossRef CAS PubMed.
- J. C. Peeken, P. Vaupel and S. E. Combs, Front. Oncol., 2017, 7, 132 CrossRef PubMed.
- K. F. Chu and D. E. Dupuy, Nat. Rev. Cancer, 2014, 14, 199–208 CrossRef CAS PubMed.
- S. H. Noh, S. H. Moon, T. H. Shin, Y. Lim and J. Cheon, Nano Today, 2017, 13, 61–76 CrossRef CAS.
- S. Dutz and R. Hergt, Nanotechnology, 2014, 25, 452001 CrossRef PubMed.
- J. Ge, N. L. Yang, Y. Q. Yang, H. Yu, X. Y. Yang, Y. J. Wang, T. Y. Wang, S. N. Cheng, Y. J. Wang, Z. H. Han, Y. Teng, J. Zou, H. L. Yang and L. Cheng, Bioact. Mater., 2023, 25, 73–85 CAS.
- A. Grillone and G. Ciofani, Chem. – Eur. J., 2017, 23, 16109–16114 CrossRef CAS PubMed.
- H. Jiang, H. Fu, T. Min, P. Hu and J. L. Shi, J. Am. Chem. Soc., 2023, 145, 13147–13160 CrossRef CAS PubMed.
- S. Y. Wang, H. Q. Jing, R. Yang, Z. Heger, S. Krizkova, Y. Zhou, X. Y. Liang, V. Adam and N. Li, Adv. Funct. Mater., 2024, 34, 2314194 CrossRef CAS.
- F. G. Qi, Q. Q. Bao, P. Hu, Y. D. Guo, Y. Yan, X. D. Yao and J. L. Shi, Biomaterials, 2024, 307, 122514 CrossRef CAS PubMed.
- B. Y. Li, M. H. Zu, A. D. Jiang, Y. G. Cao, J. X. Wua, M.-A. Shahbazi, X. X. Shi, R. L. Reis, S. C. Kundu and B. Xiao, Biomaterials, 2024, 307, 122530 CrossRef CAS PubMed.
Footnote |
† These authors contributed equally to this work. |
|
This journal is © The Royal Society of Chemistry 2025 |
Click here to see how this site uses Cookies. View our privacy policy here.