Highly sensitive OFET based room temperature operated gas sensors using a thieno[3,2-b]thiophene extended phthalocyanine semiconductor†
Received
26th July 2024
, Accepted 28th October 2024
First published on 29th October 2024
Abstract
Over the past decades, organic field-effect transistor (OFET) gas sensors have maintained a rapid development. However, the majority of OFET gas sensors show insufficient detection capability towards oxidizing and hazardous gases such as nitrogen dioxide (NO2) and sulfide dioxide (SO2). In this report, a sustainable approach toward the fabrication of OFET gas sensors, consisting of a thieno[3,2-b]thiophene (TT) and phthalocyanine (Pc) based electron rich structure (TT-Pc) for the detection of both nitrogen dioxide (NO2) and sulfide dioxide (SO2) is disclosed for the first time. Khaya gum (KG), a natural, biodegradable biopolymer is used as the gate dielectric in these OFET-based sensors. Thin film properties and surface morphology of TT-Pc were investigated by UV-Vis, SEM, AFM and contact angle measurements, which indicated a uniform and smooth film formation. The UV-Vis properties were supported by computational chemistry, performed using density functional theory (DFT) for optimizing geometry and absorption of TT-Pc models. Sensitive and selective responses of 90% and 60% were obtained from TT-Pc OFET-based sensors upon exposure to 20 ppm of NO2 and SO2, respectively, under ambient conditions. One of the lowest limits of detection of ∼165 ppb was achieved for both NO2 and SO2 using a solution-processed TT-Pc sensor with a natural, biodegradable dielectric biopolymer. The sensors showed excellent long-term environmental and operational stability with only a 7% reduction of the sensor's initial response (%) upon exposure to NO2 and SO2 over nine months of operation in air.
Introduction
Gas sensors are important for air quality monitoring and food safety detection of toxic and pollutant gases such as nitrogen dioxide (NO2), sulphur dioxide (SO2), ammonia (NH3), hydrogen sulfide (H2S) and volatile organic compounds (VOC).1–3 Conventional electronic noses (E-noses), which are generally made of a resistor-type multi-sensor array based on metal oxides, require a high operation temperature and power consumption and, hence, are not suitable for flexible and portable sensors.4 In contrast, organic semiconductor FET-based gas sensors benefit from gate-modulated carrier concentration in the channel and, thus, exhibit superior merits in room-temperature operation and sensing response signal amplification.5 OFET-based gas sensors detect gas species under certain environmental conditions by recording a change in device parameters, such as a change in OFET IDS (at a constant VGS and VDS), mobility, threshold voltage, etc.6 The employment of OFET platforms as gas sensors is an efficient method for selective gas identification and provides a fingerprint for gas recognition.7 As a general rule, the sensing mechanism of OFET sensors is described by the charge distribution at the semiconductor/dielectric interface upon exposure to gas. Depending on the properties and morphology of the active layer, the gaseous analyte may induce charge trapping/detrapping and, hence, increase/decrease of the potential barrier between continuous grains, leading to a change in the source-drain current, threshold voltage and mobility.8 Unfortunately, OFETs generally use synthetic polymer dielectrics processed from expensive and toxic organic solvents. Therefore, naturally occurring biopolymer dielectric materials deposited from low-cost, environmentally friendly solvents, such as water, are gaining attention in the fabrication of electronic devices and circuits in an environmentally friendly and sustainable way. It has been previously reported that natural gums such as gum arabic, almond gum and Khaya gum are promising dielectric materials for sustainable fabrication of low voltage OFETs.9,10 In particular, Khaya gum, a natural substance of Khaya senegalensis trees, with excellent dielectric (k value of 7), antimicrobial, non-toxicity, biodegradability, and water solubility properties offers a great opportunity for sustainable fabrication of low power OFET-based gas sensors.
Phthalocyanines (Pcs), a member of the tetraisoindole macrocyclic molecular material series, have been attracting great attention since the first day they were synthesized.11 This interest covers various fields ranging from photodynamic therapy agents in cancer treatment, nonlinear optic materials,12 photo-initiators for polymerization,13,14 chemical sensors,15–19 organic semiconductors,20 memory devices,21 and, recently, to energy-based materials. This is due to their interesting spectroscopic, photochemical, and photophysical properties as well as high thermal and optical stabilities.22 Moreover, Pcs have a conjugated 18 π-electron system, affording strong absorption and emission capabilities in the near infrared region (NIR). Additionally, their easy modification, and introduction of various central metals and axial ligands attached to the central metal help to adjust the photochemical properties of phthalocyanine molecules.23 Metal phthalocyanines (MPcs) are identified as promising organic semiconductors as gas sensors due to their outstanding thermal and chemical stability, and chemical sensitivity toward different reactive gases, especially oxidizing gases, via manipulation of the metal core.24–27 In particular, zinc phthalocyanine (ZnPc) has shown excellent electrical performance, stability, and sensing capability to detect various gaseous analytes, which make it a suitable material for applications in organic integrated circuits, sensors, and photovoltaics.2,28 Another group of well-known organic semiconductors are thienothiophenes (TTs) for energy-based materials. TTs are the simplest fused thiophenes, composed of two annulated thiophene rings, and have four isomers.29–33 The most widely used TT isomer is thieno[3,2-b]thiophene, which provides continuous conjugation through two fused thiophenes when integrated into small molecules and polymers.34–39 TTs have an extended π-conjugation, planar structure, intermolecular S–S interaction, and electron rich, flat, rigid, and good electron delocalized skeleton.40–44 These properties make them highly suitable and desirable main building blocks for organic electronics such as OLED, OFET, organic solar cells (OSC), capacitors and sensors.45–52
In this work, the design and synthesis of a first example of a TT (thieno[3,2-b]thiophene) and Pc-based semiconductor, TT-Pc, composed of nonyl-substituted TT and ZnPc, are reported. The novel TT-Pc was clarified by spectroscopic and microscopic methods including UV-Vis, scanning electron microscopy (SEM), and atomic force microscopy (AFM). TT-Pc was incorporated into a field-effect transistor device with a Khaya gum dielectric layer to detect hazardous NO2 and SO2 gas analytes, operating at low voltage with high responsivity and sensitivity at room temperature. This novel OFET-based gas sensor provides new opportunities for the scalable production of high-performance flexible sensing electronics.
Results and discussion
Design and synthesis
The monoketone (2) and TT (3) were synthesized following our previous reports.53–56 The reaction of 3-bromothiophene (1) with n-butyllithium at −78 °C was followed by the addition of elemental sulfur and then α-haloketone to produce 2 in 88% yield. Its ring closure reaction was conducted in the presence of polyphosphoric acid (PPA) in refluxing chlorobenzene to give 3 in 83% yield. The TT (3) was monostannylated using n-butyllithium at −78 °C, which was followed by the addition of tributyltin chloride to give 4. Its Stille coupling reaction with 4-iodophthalonitrile was performed using Pd(0) as a catalyst in dry DMF to construct 5 in a yield of 61% to be used as a main ligand. The cyclotetramerization reaction of 5 was conducted in the presence of zinc acetate (Zn(OAc)2) and 1,8-diazabicyclo[5.4.0]undec-7-ene (DBU) to give TT-Pc in 52% yield (Scheme 1).
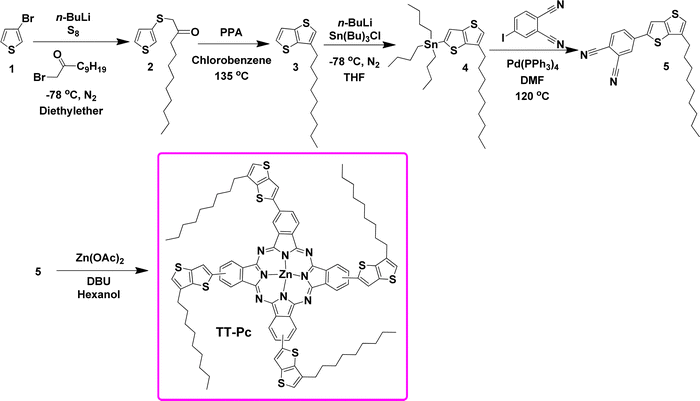 |
| Scheme 1 Synthetic routes of the TT-Pc. | |
Photophysical properties
Optical properties of TT-Pc were investigated by UV-Vis spectroscopy, which showed typical non-aggregated phthalocyanine B (Soret) and Q bands at 345 and 695 nm, respectively, in THF (Fig. 1). The optical band gap (Eoptic) was calculated to be 1.69 eV from the onset threshold wavelength of the absorption spectra at 732 nm. In addition to solution state properties, its film was deposited on the surface of ITO glass by spin-coating from a chloroform solution (2 mg mL−1) under ambient conditions. The solid-state UV-Vis maximums were determined to be 343 and 698 nm. A difference of ∼3 nm between the maximum absorptions of its solution and film spectra suggested a low interaction in solution due to the length of the four alkyl chains (C9H19) on TT cores. Moreover, the shoulder intensity around 630 nm in solution increased in the film form, indicating a typical H-aggregate behavior of zinc-phthalocyanine on TT-Pc film.57,58
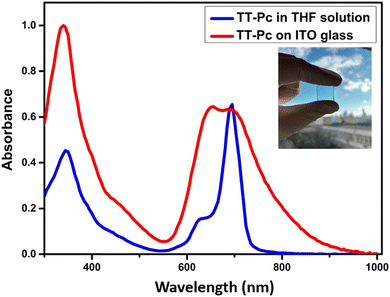 |
| Fig. 1 UV-Vis spectroscopy of TT-Pc in THF solution and on ITO glass (inset: thin film of the TT-Pc). | |
Computational study
Depending on the positions of the alkylated TT units around the Pc core, three possible conformations of TT-Pc models (TT-Pc-1, TT-Pc-2, and TT-Pc-3) were examined by computational chemistry. Different backbone geometries and their optimized structures were investigated at the level of B3LYP/6-31G(d,p) in the ground state.59 Long alkyl chains on the TT moiety were replaced with methyl groups to simplify the calculation. Fig. 2 illustrates optimized top and side views of TT-Pc-1, TT-Pc-2, and TT-Pc-3. The molecular orbitals derived from the HOMO and LUMO of Pc derivatives and their energy levels measured in eV are shown in Fig. 3. All structures displayed a similar band gap (Eg) around 2.00 eV with similar HOMO and LUMO energies around −4.90 and −2.87 eV, respectively.13,24,25 While HOMO is highly localized on the phthalocyanine core and partially on the TT unit, the phthalocyanine units contributed mainly to the LUMO. TD-DFT calculations were conducted to examine the electronic transition wavelengths and corresponding theoretical UV-Vis spectra. Fig. S1 (ESI†) presents the absorption spectra of TT-Pc structures in THF simulated at the CAM-B3LYP/6-31G(d,p) level. All the structures showed the strongest absorption at 663 nm, which is attributed to the Q band (Pc band), while the peaks observed in the region of the B band were in between the 376–383 nm range. The theoretically predicted optical maximum values were found to be consistent with the corresponding experimental data.
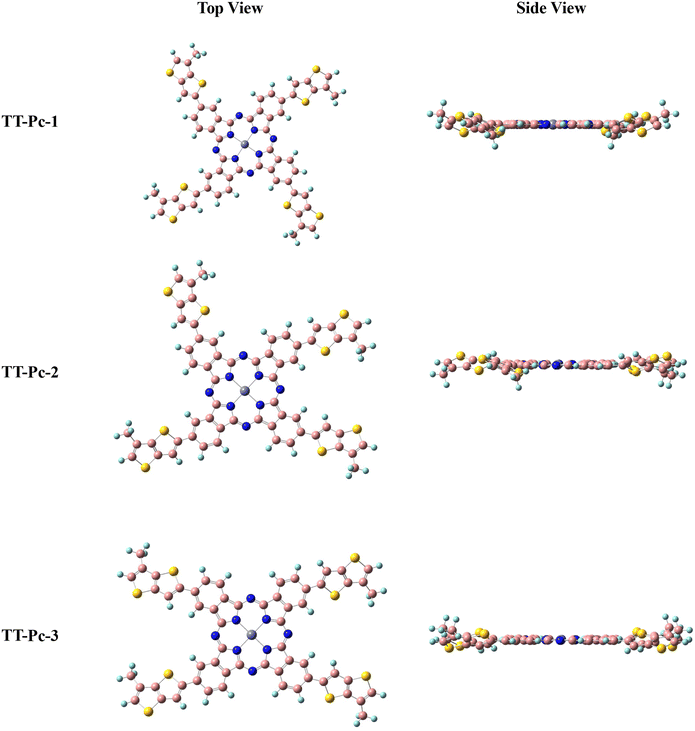 |
| Fig. 2 Top and side view of TT-Pc-1, TT-Pc-2, and TT-Pc-3 optimized at the B3LYP/6-31G(d,p) level of theory. | |
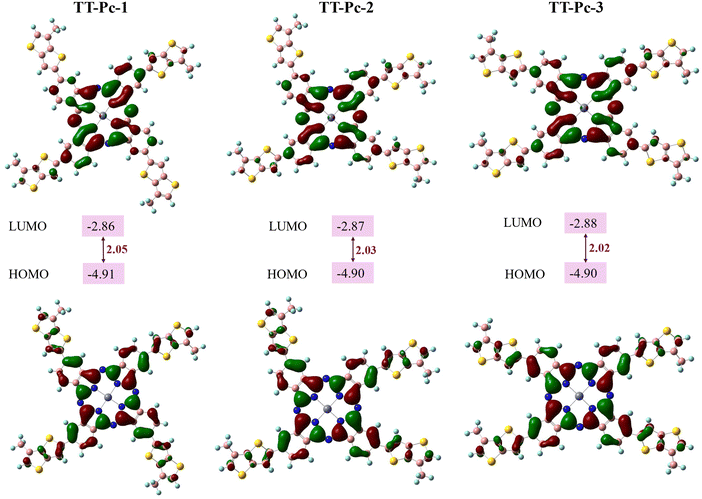 |
| Fig. 3 HOMO and LUMO energy levels of optimized TT-Pc structures at the B3LYP/6-31G(d,p) level of theory, and their energy gap values. | |
Surface topography and morphology
The surface morphology of the TT-Pc film was investigated by scanning electron microscopy (SEM) and atomic force microscopy (AFM). SEM images indicated a quite smooth and clear surface of the TT-Pc film with different scale bars of 2 and 200 μm (Fig. 4a and b). The RMS roughness, obtained from the cross section of the AFM images, was determined to be 42 nm, demonstrating a relatively uniform and smooth surface morphology (Fig. 4c and d). It was illuminated with topographic visuals that TT-Pc is a very suitable material for film formation. Moreover, further surface property was investigated by contact angle (CA) measurement (Fig. 4e). Deposition of dH2O sessile drop on the TT-Pc film showed a 122.3° CA, indicating a very high hydrophobic surface, which is higher than many Pc-based materials reported in the literature.60,61 This is possibly due to the presence of long alkyl chains on already hydrophobic TT moieties.
 |
| Fig. 4 (a) SEM image of the TT-Pc film with scale bars of 2 μm and (b) 200 μm, (c) AFM images of the TT-Pc film based on a thickness of 42 nm. (d) 3D AFM surface topographic image and cross section analyses of the TT-Pc film. (e) Contact angle image of the TT-Pc film using a water droplet. | |
OFET application and gas sensing performance
The performance of TT-Pc as an active material in low voltage OFETs and sensing layer in room temperature gas sensors was evaluated. Bottom-gate, top-contact OFET devices (Fig. 5a) using the Khaya gum dielectric layer were used as sensing platforms to detect different concentrations of nitrogen dioxide (NO2) and sulphur dioxide (SO2) gases. Naturally occurring and biodegradable Khaya gum (KG) was used as a high-k (k ≈ 7)62 gate dielectric layer to realize sustainable manufacturing of low-power, low-waste ‘green’ sensors.63 The KG used in this work was collected from exudates of Khaya senegalensis trees growing in Senegal (Fig. 5b). It is a natural polysaccharide known for its biodegradability, non-toxicity, water solubility, great dielectric properties64 and anti-inflammatory features.65 The purification process of KG powder (Fig. 5c) is described elsewhere.62 A 2.5 wt% solution of KG in deionized water was spun at 3500 rpm for 2 min onto pattered aluminum gate electrodes. The KG layer was annealed at 100 °C for 30 minutes under ambient conditions, giving a thickness of ∼200 nm. The TT-Pc was, then, spin-coated as the semiconductor channel from a 1 wt% 1,2-dichlorobenzene solution and annealed at 90 °C for 2 hours. Finally, source and drain gold electrodes were evaporated throwing shadow masks, creating OFETs with a channel length (L) and width (W) of 30 μm and 1000 μm, respectively.
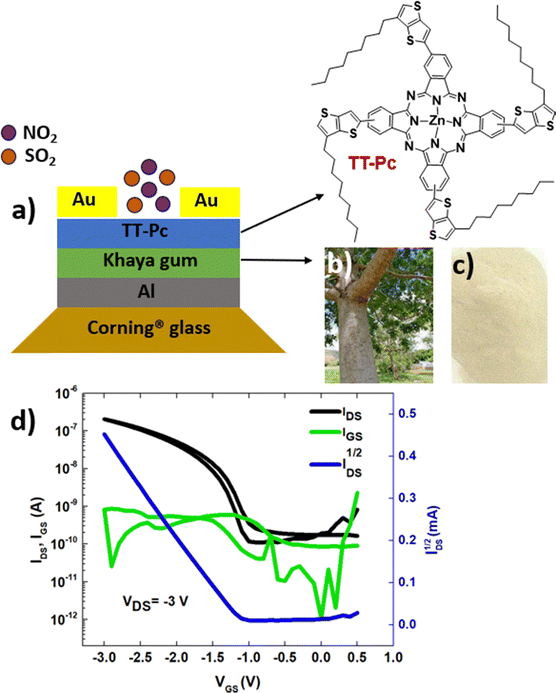 |
| Fig. 5 (a) Configuration of the fabricated bottom-gate, top-contact OFET platform as a gas sensor, (b) Khaya senegalensis tree, (c) Khaya gum powder post-purification and (d) transfer characteristics of TT-Pc OFETs. | |
Each substrate consisted of 20 OFETs with identical channel dimensions. Each transistor was characterized under ambient conditions (air with 45% relative humidity) using an Agilent E5270B measurement mainframe with Karl Suss PH100 micromanipulator probes. All the OFET parameters were calculated in the saturation regime using the standard metal-oxide semiconductor field-effect transistor (MOSFET) equation:66
where
IDS,sat is the drain current in the saturation regime,
W is the channel width,
L is the channel length,
μ represents the field-effect carrier mobility,
Ci is the areal capacitance of the dielectric layer and
VGS and
Vth are the gate voltage and threshold voltage, respectively. As previously reported, the
Ci value for Khaya gum is calculated to be ∼130 nF cm
−2 at 1 kHz.
62
The transfer and leakage current characteristics of the OFETs using TT-Pc as the active material are presented in Fig. 5d and Fig. S2 (ESI†), respectively. The transistors were operated in the accumulation mode and distinctively displayed p-type field-effect behavior under a gate voltage (VGS) of −3 V. As observed from the transfer curve, devices exhibit a good pinch-off with clear distinctive linear and saturation regimes and negligible hysteresis between the forward and reverse sweeps. The leakage current through the Khaya gum dielectric layer is more than two orders of magnitude lower than the “on” current (IDS) at −3 V. The figures-of-merit of 20 characterized OFETs are collated in Table 1. The average hole mobility (∼0.025 cm2 V−1 s−1) of the OFETs in this work are comparable to and in some cases higher than those in the literature using solution processed ZnPc. The mobility values of MPcs depend on the central metal atom, gate dielectric, and the deposition techniques.67 In addition, these low voltages operating OFETs showed a significantly smaller subthreshold swing (at least one order of magnitude) than similar devices using MPcs.68–70 Moreover, compared to the previously reported solution-processed OFETs using Khaya gum as the dielectric layer,62 these TT-Pc transistors showed a notably smaller (two folds) subthreshold swing. Nevertheless, compared to their counterparts, the herein fabricated OFETs, applying solution-processed TT-Pc, yielded superior field-effect performance, particularly at very low voltages, using a naturally occurring dielectric layer.
Table 1 Figures-of-merit of TT-Pc OFETs
Average hole mobility (μsat), cm2 V−1 s−1 |
Threshold voltage Vth, V |
I
on/Ioff |
Subthreshold swing (SS), mV dec−1 |
2.5 × 10−2 ± 0.5 |
−1.1 ± 0.1 |
>103 |
230 ± 20 |
The characterized TT-Pc OFETs were subsequently evaluated as gas sensing platforms upon exposure to different concentrations of two redox gases, i.e. nitrogen dioxide (NO2) and sulphur dioxide (SO2), under ambient conditions (23 °C and 45% relative humidity). In this context, we report highly sensitive room temperature operated NO2 and SO2 gas sensors based on low-voltage, and TT-Pc-based OFETs, using naturally occurring Khaya gum as the dielectric material. Different concentrations of NO2 and SO2 were obtained by feeding a mixture of dry air and the selected gaseous analytes in appropriate volumes through a mass flow controller. Prior to introducing the adjusted concentrations to the sensors, a baseline was recorded for exposure of the devices to dry air only. The OFET-based sensors were then exposed to sequential concentrations, from 20 ppm down to 100 ppb, of each gas separately under ambient conditions.
The transfer curves of OFETs in dry air and after exposure to each specific concentration of NO2 and SO2 are plotted in Fig. 6. Both sets of IDSvs. VGS curves manifested a directly proportional increase in IDS as the concentration of the target gases increased. NO2 is an oxidizing gas that is believed to increase the conductivity of p-type OFETs during exposure.28 NO2 diffuses into the TT-Pc active layer and displaces absorbed species such as oxygen, creating charge carriers (holes) in the semiconducting layer, thereby, increasing the IDS of OFET at a constant bias and inducing positive shifts in Vth (Fig. 6a). On the other hand, where the target gas is the nonoxidizing SO2, the binding of the gas molecule to both the electron donating thienothiophene and the zinc center of ZnPc of the active layer may alter conduction through the entire semiconductor layer. Similarly, an increase in IDS and positive shift in Vth of OFETs is recorded upon exposure to different concentrations of SO2 (Fig. 6b). In agreement with the literature,71 the thiophene monomer donates to or accepts electrons from specific analytes, for instance, SO2 and NH3, respectively, which results in a positive IDS change for SO2. Nonetheless, it is highly speculated that both target gas molecules can bind to the zinc metal at the center of the phthalocyanine in TT-Pc and cause a change in the overall conduction of the active channel.28
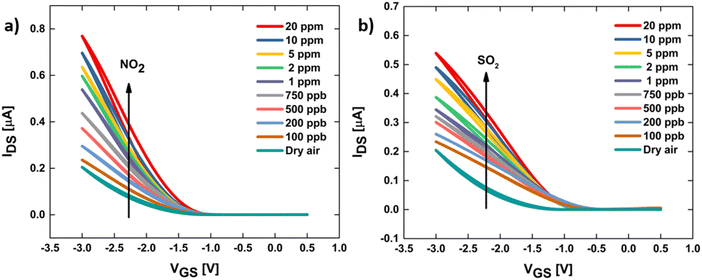 |
| Fig. 6 Transfer characteristics of TT-Pc OFET platforms in response to dry air and various concentrations of (a) NO2 and (b) SO2 at room temperature. | |
The room temperature transient response of sensors upon exposure to sequential concentrations of NO2 and SO2 from 20 ppm down to 100 ppb under ambient conditions was investigated (Fig. 7). The exposure and recovery times were found to be 2 and 5 minutes, respectively.
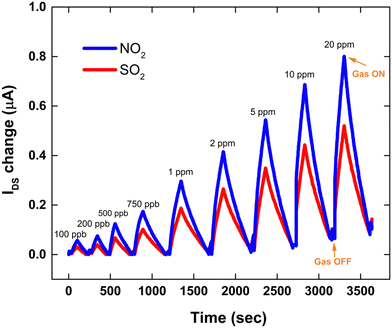 |
| Fig. 7 Room temperature transient sensor response upon exposure to sequential concentrations of NO2 and SO2 from 20 ppm down to 100 ppb. | |
In agreement with the transfer curves plotted in Fig. 8, a progressively significant increase in the transient responses of the TT-Pc OFET-based sensors was recorded upon exposure to sequential concentrations of NO2 and SO2 from 20 ppm down to 100 ppb. The sensor showed good response and recovery to different concentrations of both gases. However, a higher IDS change (about 25%) was observed for NO2, compared to SO2. This could be attributed to the stronger interaction of oxidizing NO2 and higher sensitivity of the TT-Pc active layer to NO2. At 20 ppm, a substantial 0.8 μA and 0.5 μA increase in IDS was recorded for NO2 and SO2, respectively. It is worth mentioning that, as the concentration of the gaseous analytes increased, a moderate drift from the original baseline was observed for both gases only at higher concentrations. Although MPcs demonstrate a very high sensitivity towards oxidizing gases like NO2, they suffer from a prolonged recovery. This slow recovery is due to strong chemisorption of the gas molecules onto the sensor surface.72 However, in this work, the sensors consistently recovered to the baseline within 5 minutes assigned time, showing reversibility with negligible drift for lower gas concentrations (<2 ppm). This was ascribed to the adopted top-contact configuration of the OFET platform, which only exposes the channel area (3 × 10−4 cm2) to the analyte and the remaining semiconductor layer under the top contacts is kept intact. The thinner the semiconductor layer and the smaller the active area of the OFET sensor exposed to the gas are, the faster the response and the recovery time will become.
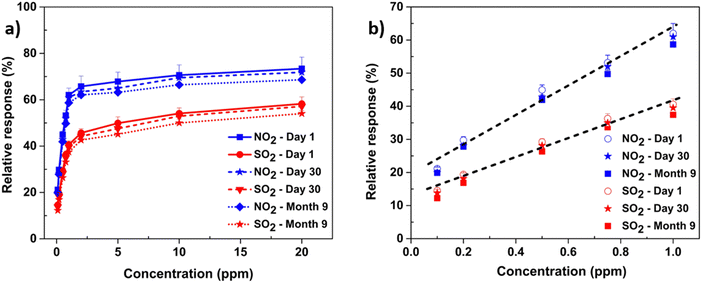 |
| Fig. 8 (a) The NO2 and SO2 sensors’ calibration curves and (b) the linear fit to the response at a lower concentration regime for both NO2 and SO2. | |
As a key merit of gas sensors, the response of the OFET platforms upon exposure to the target gases was calculated using the equation:
where
IGas is the
IDS value after exposure to the selected gas and
I0 is the baseline value, immediately before exposure. Moreover, an evaluation of responses from the baseline (dry air) is required. The calibration curve obtained by plotting the response (
R) for each gaseous analyte
vs. its concentrations is shown in
Fig. 8a.
Significant and never previously reported responses of 90% and 60% were obtained for the TT-Pc OFETs upon exposure to 20 ppm of NO2 and SO2 (using air as carrier gas), respectively, under ambient conditions. Moreover, a distinguishable differentiation between the sensor responses to NO2 and SO2 is observed, which makes these low-voltage TT-Pc OFETs a promising gas sensor for room temperature operation with high sensitivity.
Long-term stability of the sensor's performance was evaluated over one month and nine months of operation in air. The experimental results in Fig. 8a showed only a 5–7% reduction of the sensor's initial response (%) upon exposure to NO2 and SO2 of the same concentration range. The linearity of the calibration curve for the sensor's responses to both gases obtained on day 1, day 30 and month 9 (Fig. 8b), was determined by adequate regression analysis of the data of the lower concentrations. Based on the linear fit of the calibration curve, the theoretical lower limit of detection (LOD) is extrapolated. The LOD is defined as the lowest concentration of a gas corresponding to the smallest signal that can be detected with reasonable certainty, but not necessarily quantified as an exact value.8 It is often denoted as (3 × N/S), where N is the noise of the baseline (the sensors response in the absence of gas) and S is the sensitivity of the sensor. From the linear fit in Fig. 8b, the LOD for both NO2 and SO2 was estimated to be ∼165 ppb, which is one of the lowest limits of detection reported for solution-processed, low-voltage OFET-based sensors using natural, biodegradable dielectric biopolymers.72,73 As collated in Table 2, although a lower value of LOD for NO2 was reported using ZnO nanowire-FET, the sensor was fabricated at a very high temperature, operated at high voltage and detected only one type of gas. Similarly, despite a lower limit of detection, graphene-based sensors were not only fabricated using high-temperature and timely techniques, but also operated at high voltage with a single-element sensing capability. On the other hand, OFET sensors using DPP-based polymers that showed a much lower limit of detection for SO2, were operating at high voltage and did not show any response against other gases, for example NO2. Moreover, current examples of nanosheet-based materials have also been used as sensors for NO2, but these materials require high operation temperature and power consumption.74–82
Table 2 Summary and comparison of the morphology and charge transport characteristics of various OFET-based gas sensors in the literature and the present work
Sensing material |
Deposition |
Dielectric |
Operating voltage (V) |
Detected gas |
LOD (ppb) |
Ref. |
SWCNT |
Hot filament chemical vapor deposition (HF-CVD) |
SiO2 |
−4 |
NO2 |
560 |
74
|
SWCNT |
Aerosol jet printed |
AlOx |
−1.5 |
NO2 |
69 |
75
|
ZnO-NW |
Printing and calcination at 200–700° |
SiO2 |
80 |
NO2 |
0.05 |
76
|
Mg-porphyrin modified graphene |
CVD |
SiO2 |
40 |
NO2 |
52 |
77
|
Pentacene |
Thermal evaporation |
Polystyrene on SiO2 |
−60 |
NO2 |
100 |
78
|
P3HT |
Spin-cast |
PMMA/poly-TPD blend (solution-cast) |
−40 |
NO2 |
242.6 |
79
|
DPP-based polymers |
Spin-cast |
SiO2 |
−80 |
SO2 |
0.24 |
80
|
MoS2 nanosheets |
Thermal evaporation |
— |
6 |
NO2 |
62.5 |
81
|
ZnIn2S4 nanosheets |
Thermal evaporation |
— |
−0.6 |
NO2 |
130 |
82
|
TT-Pc
|
Spin-cast |
Natural biopolymer (Khaya gum) processed from water |
<−3 |
NO2 and SO2 |
165 |
This work |
Herein, the reported TT-Pc sensors show excellent sensitivity, low LOD, good response time and reversibility, low drift, long-term environmental and operational stability, and good sensing response to oxidizing NO2 and non-oxidizing SO2 gases. The sensors were only tested under ambient conditions, without varying the moisture content of the gas mixture to avoid probable degradation of the sensing material upon repetitive exposure. This approach allowed evaluation of the sensor's long-term operational stability in the detection of NO2 and SO2. Using natural biopolymer Khaya gum enables sustainable fabrication of low-cost, low-power gas sensors for cost-effective, fast, and reliable domestic, industrial and ecosystem air quality monitoring.
Conclusion
In this work, a novel, sustainable strategy for low power, low-cost OFET-based NO2 and SO2 sensors with high responsivity and sensitivity has been realized using TT-Pc as an active layer. Naturally occurring Khaya gum processed from water is used as the dielectric layer to promote sustainable and environmentally friendly fabrication of the reported gas sensors. TT-Pc, composed of nonyl substituted thienothiophene (TT) and Zn phthalocyanine (ZnPc), was clarified by SEM, AFM and contact angle measurements, indicating excellent film formation and a superior hydrophobic surface with 122.3° water droplet. TT-Pc was successfully fabricated for a low voltage OFET device using biodegradable and water soluble Khaya gum (KG) dielectric at room temperature. Remarkable responses of 90% and 60% were achieved for the TT-Pc OFETs upon exposure to 20 ppm of NO2 and SO2, respectively, under ambient conditions. The obtained LOD of ∼165 ppb for both NO2 and SO2 is one of the lowest recorded for solution-processed polymer sensors using biodegradable dielectric materials. Moreover, these low-voltage OFET-based sensors yielded great long-term stability with less than 7% reduction of the sensor's initial response (%) upon exposure to NO2 and SO2 over nine months of operation in air. Briefly, the present study introduced a sustainable approach towards the fabrication of a novel OFET sensor for the detection of toxic and pollutant NO2 and SO2 gases based on a TT-Pc structure that can satisfy the rigorous requirements for highly sensitive and stable gas sensors for practical applications with the possibility of reducing their adverse environmental impacts.
Experimental details
Materials
All the reagents were purchased from Aldrich and Acros and used without further purification. All the solvents, used in the syntheses, were of technical grade and freshly distilled prior to use. The solvents, used in spectroscopic measurements, were of spectroscopic grade. Flash chromatography was performed with ≤0.063 μm silica gel. 1H and 13C NMR spectra were recorded on a Varian 500 and 126 MHz, respectively, spectrometer. Proton and carbon chemical shifts are reported in parts per million downfield from tetramethylsilane, TMS.
Synthesis
Synthesis of 1-(thiophen-3-ylthio)undecan-2-one (2).
To a solution of 3-bromothiophene 1 (2.0 g, 12.25 mmol) in dry diethyl ether (40 mL), a solution of n-butyllithium in hexane (5.4 mL, 13.50 mmol, 2.5 M) was added dropwise at −78 °C, under a nitrogen atmosphere. After the reaction was stirred for 1 h, elemental sulfur (S8) (0.41 g, 12.85 mmol) was added, and the mixture was further stirred for 45 min. Then, 1-bromoundecan-2-one (3.66 g, 14.70 mmol) was introduced portion wise. Stirring was continued overnight, and the reaction was quenched with water. The solution was extracted with dichloromethane, and the organic layer was washed with Na2CO3 solution (10%) and water. The organic layer was dried over Na2SO4, filtered and the solvent was evaporated under reduced pressure. The crude product was purified by flash column chromatography eluting with a mixture of n-hexane
:
CH2Cl2 (5
:
1) to give the title compound 2 as a yellowish powder (3.07 g, 88%). 1H NMR (500 MHz, CDCl3) δ 7.33 (dd, J = 5.0, 3.0 Hz, 1H), 7.22 (dd, J = 3.0, 1.3 Hz, 1H), 7.03 (dd, J = 5.0, 1.3 Hz, 1H), 3.58 (s, 2H), 2.57 (t, J = 7.4 Hz, 2H), 1.62–1.58 (m, 2H), 1.33–1.22 (m, 12H), 0.89 (t, J = 7.0 Hz, 3H). 13C NMR (126 MHz, CDCl3) δ 205.77, 130.04, 129.59, 126.53, 124.98, 45.00, 40.68, 31.85, 30.92, 29.39, 29.34, 29.24, 29.11, 23.82, 22.65, 14.10.
Synthesis of 3-nonylthieno[3,2-b]thiophene (3).
To a solution of polyphosphoric acid (PPA) (3.58 g, 35.10 mmol) in chlorobenzene (10 mL) at 135 °C was added 2 (1.0 g, 3.51 mmol), dissolved in chlorobenzene (5 mL), dropwise. The reaction was stirred at this temperature for 8 h, after which the mixture was extracted with CH2Cl2, NaHCO3 solution (10%) and water. The organic layer was dried over Na2SO4, filtered and the solvent was evaporated under reduced pressure. The residue was purified by column chromatography eluting with a mixture of n-hexane to obtain the title compound 3 as a yellowish oil (0.78 g, 83%). 1H NMR (500 MHz, CDCl3) δ 7.38 (d, J = 6.3 Hz, 1H), 7.27 (d, J = 5.2 Hz, 1H), 7.02 (s, 1H), 2.76 (t, J = 7.7 Hz, 2H), 1.82–1.75 (m, 2H), 1.42–1.29 (m, 12H), 0.92 (t, J = 6.2 Hz, 3H). 13C NMR (126 MHz, CDCl3) δ 139.96, 138.70, 134.89, 126.54, 121.70, 119.90, 31.91, 29.98, 29.55, 29.42, 29.39, 29.33, 28.63, 22.70, 14.13.
Synthesis of tributyl(6-nonylthieno[3,2-b]thiophen-2-yl)stannane (4).
To a solution of 3 (600 mg, 2.25 mmol) in 40 mL THF, a solution of n-butyllithium in hexane (1.80 mL, 2.82 mmol, 1.6 M) was added dropwise at −78 °C under a nitrogen atmosphere. The solution was stirred at −78 °C for 1 h and 1.0 M solution of tributyltin chloride (4.23 mL, 4.23 mmol) in THF was added in one portion. The solution was warmed to room temperature and stirred overnight. The reaction was quenched with water. The organic layer was extracted with twice brine and water. The organic layer was dried over anhydrous Na2SO4. The organic layer was evaporated under reduced pressure and the product 4 was used for the next step without further purification.
Synthesis of 4-(6-nonylthieno[3,2-b]thiophen-2-yl)phthalonitrile (5).
To a solution of 4-iodophthalonitrile (200 mg, 0.79 mmol) and 4 (670 mg, 1.20 mmol), dissolved in DMF (20 mL) and degassed for 20 min under nitrogen, was added Pd(PPh3)4 (91 mg, 0.079 mmol) at room temperature in the dark. The reaction mixture was heated and stirred at 120 °C for 48 h. The reaction flask was then cooled to room temperature and the mixture was poured into a concentrated KF solution. The resulting suspension was extracted several times with CH2Cl2. Afterward, the combined organic layers were washed with distilled water and dried over anhydrous sodium sulfate. The solvent was removed under reduced pressure and the crude product was purified by column chromatography eluting with n-hexane
:
CH2Cl2 (1
:
1) to obtain light yellow pure compound 5 (187 mg, 61%). 1H NMR (500 MHz, CDCl3) δ 7.94 (s, 1H), 7.85 (d, J = 8.3 Hz, 1H), 7.74 (d, J = 9.0 Hz, 1H), 7.61 (d, J = 1.1 Hz, 1H), 7.12 (d, J = 0.6 Hz, 1H), 2.73 (t, J = 7.7 Hz, 2H), 1.76 (dt, J = 14.6, 7.1 Hz, 2H), 1.39–1.27 (m, 12H), 0.89 (t, J = 6.5 Hz, 3H). 13C NMR (126 MHz, CDCl3) δ 141.39, 140.06, 139.92, 139.78, 135.22, 133.96, 129.50, 128.82, 124.69, 119.39, 116.58, 115.50, 115.24, 112.94, 31.89, 29.79, 29.53, 29.40, 29.35, 29.31, 28.57, 22.69, 14.15. MALDI-TOF-MS (m/z): [M] calcd for C23H24N2S2, 392.58; found, 391.382.
Synthesis of TT-Pc.
A mixture of (5) (190 mg, 0.48 mmol) and anhydrous zinc acetate (29.6 mg, 0.16 mmol) in dry hexanol (1.5 mL) in a Schlenk tube was sonicated for 5 min. Subsequently, the reaction mixture was stirred for 20 min under an argon atmosphere and heated to 120 °C. At this temperature DBU (50.0 μL) was added and the tube was sealed. The reaction mixture was stirred at 160 °C for 6 h. It was then cooled to room temperature and the mixture was precipitated in methanol and centrifuged. The crude product was purified by column chromatography eluting initially with CH2Cl2
:
THF (20
:
1) and then THF. The solid product was washed with hot methanol several times to obtain TT-Pc as a dark green powder (98 mg, 52%). 1H NMR (600 MHz, THF-d8) δ 11.18–10.55 (m, 10H), 9.92 (m, 8H), 9.14 (d, J = 16.8 Hz, 2H), 4.90–4.74 (m, 7H), 4.35–4.26 (m, 7H), 3.80 (8H), 3.49–3.25 (m, 45H), 2.83 (m, 9H). 13C NMR (151 MHz, THF-d8) δ 153.01, 152.89, 147.19, 147.11, 147.09, 145.37, 145.29, 142.40, 142.20, 141.14, 141.10, 140.60, 140.56, 140.52, 140.49, 139.52, 139.44, 137.55, 137.46, 137.39, 137.02, 136.45, 136.10, 126.85, 126.75, 126.48, 126.39, 125.85, 125.82, 123.74, 123.60, 123.44, 123.41, 123.01, 119.67, 119.44, 119.28, 118.23, 118.16, 118.08, 117.99, 33.20, 33.18, 32.88, 31.09, 30.96, 30.88, 30.85, 30.75, 30.67, 30.62, 30.14, 30.01, 23.87, 14.76, 14.75, 13.41, 13.35, 13.31. MALDI-TOF-MS (m/z): [M] calcd for C92H96N8S8Zn, 1635.70; found, 1635.103.
Data availability
The authors confirm that the data supporting the findings of this study are available within the article and its ESI.† Raw data that support the findings of this study are available from the corresponding author, upon reasonable request.
Conflicts of interest
There are no conflicts to declare.
Acknowledgements
The authors thank ITU (Istanbul Technical University), TDA-2022-43696, TDA-2024-45680, TGA-2023-44077 and TGA-2023-45124 numbered ITU BAP Projects, 122Z568 numbered TUBITAK 1001 Project and Unsped Global Lojistik, Turkey, for financial supports. The authors would like to thank Prof. Abdou Karim Diallo at the Université Gaston Berger for providing the Khaya gum.
References
- W. Huang, J. Sinha, M.-L. Yeh, J. F. M. Hardigree, R. LeCover, K. Besar, A. M. Rule, P. N. Breysse and H. E. Katz, Adv. Funct. Mater., 2013, 23, 4094–4104 CrossRef CAS
.
- H. Gou, G. Wang, Y. Tong, Q. Tang and Y. Liu, Org. Electron., 2016, 30, 158–164 CrossRef CAS
.
- A. Kumar, R. Meunier-Prest and M. Bouvet, Sensors, 2020, 20, 4700 CrossRef CAS
.
- Z. Song, Q. Tang, Y. Tong and Y. Liu, IEEE Electron Device Lett., 2017, 38(11), 1586–1589 CAS
.
- C. Zhang, P. Chen and W. Hu, Chem. Soc. Rev., 2015, 44, 2087–2107 RSC
.
- S. Han, Z. Yang, Z. Li, X. Zhuang, D. Akinwande and J. Yu, ACS Appl. Mater. Interfaces, 2018, 10(44), 38280–38286 CrossRef CAS
.
- L. Torsi, A. Dodabalapur, L. Sabbatini and P. G. Zambonin, Sens. Actuators, B, 2000, 67(3), 312–316 CrossRef CAS
.
- L. Torsi, M. Magliulo, K. Manoli and G. Palazzo, Chem. Soc. Rev., 2013, 42, 8612–8628 RSC
.
- M. Seck, N. Mohammadian, A. K. Diallo, S. Faraji, M. Saadi, M. Erouel, E. H. B. Ly, K. Khirouni and L. A. Majewski, Synth. Met., 2020, 267, 116447 CrossRef CAS
.
- M. Seck, N. Mohammadian, A. K. Diallo, S. Faraji, M. Saadi, M. Erouel, E. H. B. Ly, K. Khirouni and L. A. Majewski, Org. Electron., 2020, 83, 105 CrossRef
.
-
A. B. P. Lever and C. C. Leznoff, Phthalocyanines: Properties and Applications, VCH, New York, pp. 1989–1996 Search PubMed
.
- M. Louzada, J. Britton, T. Nyokong and S. Khene, J. Phys. Chem. A, 2017, 121(38), 7165–7175 CrossRef CAS PubMed
.
- L. Breloy, O. Yavuz, I. Yilmaz, Y. Yagci and D. L. Versace, Polym. Chem., 2021, 12(30), 4291–4316 RSC
.
- L. Breloy, V. Brezová, A. Blacha-Grzechnik, M. Presset, M. S. Yildirim, I. Yilmaz, Y. Yagci and D. L. Versace, Macromolecules, 2020, 53, 112–124 CrossRef CAS
.
- O. Yavuz, Y. Alcay, K. Kaya, M. Sezen, S. K. Atasen, M. S. Yildirim, Y. Ozkilic, N. S. Tuzun and I. Yilmaz, Inorg. Chem., 2019, 58(1), 909–923 CrossRef CAS PubMed
.
- O. Yavuz, M. Sezen, Y. Alcay, M. S. Yildirim, K. Kaya, Y. Ozkilic, N. Ş. Tuzun and I. Yilmaz, Sens. Actuators, B, 2021, 329, 129002 CrossRef CAS
.
- O. Yavuz, M. Sezen, Y. Alcay, M. S. Yildirim, H. Aribuga, E. Ozdemir, U. Ertugral, Y. Ozkilic, N. S. Tuzun and I. Yilmaz, Spectrochim. Acta, Part A, 2023, 284, 121484 CrossRef CAS
.
- D. Cetin, O. Yavuz, Y. Alcay, M. S. Yildirim, M. Kaplan, H. Aribuga, E. Ozdemir, U. Ertugral and I. Yilmaz, Spectrochim. Acta, Part A, 2023, 297, 122725 CrossRef CAS PubMed
.
- M. S. Yildirim, Y. Alcay, O. Yavuz, S. K. Atasen, Z. Mermer, H. Aribuga and I. Yilmaz, Anal. Chim. Acta, 2022, 1198, 339531 CrossRef CAS
.
- G. De La Torre, C. G. Claessens and T. Torres, Chem. Commun., 2007, 2000–2015 RSC
.
- B. Das, M. Samanta, P. Sarkar, U. K. Ghorai, A. Mallik and K. K. Chattopadhyay, Adv. Electron. Mater., 2021, 7(4), 2001079 CrossRef CAS
.
- G. Lu, X. Kong, P. Ma, K. Wang, Y. Y. Chen and J. Jiang, ACS Appl. Mater. Interfaces, 2016, 8(9), 6174–6182 CrossRef CAS PubMed
.
- T. Nyokong, Coord. Chem. Rev., 2007, 251(13–14), 1707–1722 CrossRef CAS
.
- M. Chaabene, B. Gassoumi, P. Mignon, R. B. Chaabane and A. R. Allouche, J. Mol. Graphics, 2019, 88, 174–182 CrossRef CAS PubMed
.
- A. G. Martynov, J. Mack, K. A. May, T. Nyokong, Y. G. Gorbunova and A. Y. Tsivadze, ACS Omega, 2019, 4, 7265–7284 CrossRef CAS
.
- F. I. Bohrer, A. Sharoni, C. Colesniuc, J. Park, I. K. Schuller, A. C. Kummel and W. C. Trogler, J. Am. Chem. Soc., 2007, 129(17), 5640–5646 CrossRef CAS
.
- S. Ji, H. Wang, T. Wang and D. A. Yan, Adv. Mater., 2013, 25, 1755–1760 CrossRef CAS
.
- F. D’Souza, E. Maligaspe, K. Ohkubo, M. E. Zandler, N. K. Subbaiyan and S. Fukuzumi, J. Am. Chem. Soc., 2009, 131(25), 8787–8797 CrossRef PubMed
.
- R. Isci, E. Tekin, K. Kaya, S. P. Mucur, S. F. Gorkem and T. Ozturk, J. Mater. Chem. C, 2020, 8, 7908–7915 RSC
.
- R. Isci, E. Tekin, S. P. Mucur and T. Ozturk, ChemistrySelect, 2020, 5, 13091–13098 CrossRef CAS
.
- M. E. Cinar and T. Ozturk, Chem. Rev., 2015, 115(9), 3036–3140 CrossRef CAS PubMed
.
- G. Turkoglu, M. E. Cinar and T. Ozturk, Eur. J. Org. Chem., 2017, 4552–4561 CrossRef CAS
.
- R. Isci, M. Unal, G. Kucukcakir, N. A. Gurbuz, S. F. Gorkem and T. Ozturk, J. Phys. Chem. B, 2021, 125, 13309–13319 CrossRef CAS PubMed
.
- R. Isci, A. R. Varzeghani, K. Kaya, B. Sütay, E. Tekin and T. Ozturk, ACS Sustainable Chem. Eng., 2022, 10(4), 1605–1615 CrossRef CAS
.
- R. Isci, E. Baysak, G. Kesan, B. Minofar, M. S. Eroglu, O. Duygulu, S. F. Gorkem and T. Ozturk, Nanoscale, 2022, 14, 16602–16610 RSC
.
- B. Amna, R. Isci, H. M. Siddiqi, L. A. Majewski, S. Faraji and T. Ozturk, J. Mater. Chem. C, 2022, 10(21), 8254–8265 RSC
.
- R. Isci, D. Gunturkun, A. S. Yalin and T. Ozturk, J. Polym. Sci., 2021, 59, 117–123 CrossRef CAS
.
- R. Isci, L. Wan, S. Topal, D. Gunturkun, A. J. Campbell and T. Ozturk, J. Mater. Chem. C, 2022, 10(29), 10719–10727 RSC
.
- R. Isci, E. Gencosman, Y. Yagci and T. Ozturk, J. Photochem. Photobiol., A, 2024, 449, 115427 CrossRef CAS
.
- H. Bronstein, Z. Chen, R. S. Ashraf, W. Zhang, J. Du, J. R. Durrant, P. S. Tuladhar, K. Song, S. E. Watkins, Y. Geerts, M. M. Wienk, R. A. J. Janssen, T. Anthopoulos, H. Sirringhaus, M. Heeney and I. McCulloch, J. Am. Chem. Soc., 2011, 133(10), 3272–3275 CrossRef CAS
.
- F. Turksoy, J. D. Wallis, U. Tunca and T. Ozturk, Tetrahedron, 2003, 59(41), 8107–8116 CrossRef CAS
.
- E. Ertas and T. Ozturk, Tetrahedron Lett., 2004, 45(17), 3405–3407 CrossRef CAS
.
- G. Yildiz, Z. Aydogmus, M. E. Cinar, F. Senkal and T. Ozturk, Talanta, 2017, 173, 1–8 CrossRef CAS
.
- G. M'Baye, A. S. Klymchenko, D. A. Yushchenko, V. V. Shvadchak, T. Ozturk, Y. Mély and G. Duportail, Photochem. Photobiol. Sci., 2007, 6(1), 71–76 CrossRef
.
- T. Ozturk, C. R. Rice and J. D. Wallis, Mater. Chem., 1995, 5, 1553–1556 RSC
.
- E. Akman, S. Akin, T. Ozturk, B. Gulveren and S. Sonmezoglu, Sol. Energy, 2020, 202, 227–237 CrossRef CAS
.
- N. Saygili, R. J. Brown, P. Day, R. Hoelzl, P. Kathirgamanathan, E. R. Mageean, T. Oztuk, M. Pilkington, M. M. B. Qayyum, S. S. Turner, L. Vorgerrw and J. D. Wallis, Tetrahedron, 2001, 57(23), 5015–5026 CrossRef CAS
.
- R. Isci, T. Balkan, S. Tafazoli, B. Sütay, M. S. Eroglu and T. Ozturk, ACS Appl. Energy Mater., 2022, 5, 13284–13292 CrossRef CAS
.
- S. E. Ozturk, R. Isci, S. Faraji, B. Sütay, L. A. Majewski and T. Ozturk, Eur. Polym. J., 2023, 191, 112028 CrossRef CAS
.
- D. Gunturkun, R. Isci, B. Sütay, L. A. Majewski, S. Faraji and T. Ozturk, Eur. Polym. J., 2022, 170, 111167 CrossRef CAS
.
- R. Isci and T. Ozturk, Turk. J. Chem., 2023, 47(5), 1239–1248 CrossRef PubMed
.
- A. Suerkan, R. Isci, T. Ozturk and Y. Yagci, Mol. Syst. Des. Eng., 2023, 8(10), 1319–1326 RSC
.
- R. Isci, M. Unal, T. Yesil, A. Ekici, B. Sütay, C. Zafer and T. Ozturk, Front. Mater., 2023, 10, 1125462 CrossRef
.
- D. Gunturkun, R. Isci, S. Faraji, B. Sütay, L. A. Majewski and T. Ozturk, J. Mater. Chem. C, 2023, 11(38), 13129–13141 RSC
.
- R. Isci and T. Ozturk, Beilstein J. Org. Chem., 2023, 19, 1849–1857 CrossRef CAS
.
- R. Isci, K. B. Donmez, Y. Karatepe and T. Ozturk, ACS Appl. Energy Mater., 2024, 7(4), 1488–1494 CrossRef CAS
.
- M. Bayda, F. Dumoulin, G. L. Hug, J. Koput, R. Gorniaka and A. Wojcik, Dalton Trans., 2017, 46, 1914–1926 RSC
.
- S. Doria, A. Lapini, M. D. Donato, R. Righini, N. Azzaroli, A. Iagatti, J. R. Caram, T. S. Sinclair, L. Cupellini, S. Jurinovich, B. Mennucci, G. Zanotti, A. M. Paoletti, G. Pennesi and P. Fogg, Phys. Chem. Chem. Phys., 2018, 20, 22331–22341 RSC
.
-
M. J. Frisch, G. W. Trucks, H. B. Schlegel, G. E. Scuseria, M. A. Robb, J. R. Cheeseman, G. Scalmani, V. Barone, G. A. Petersson, H. Nakatsuji, X. Li, M. Caricato, A. V. Marenich, J. Bloino, B. G. Janesko, R. Gomperts, B. Mennucci, H. P. Hratchian, J. V. Ortiz, A. F. Izmaylov, J. L. Sonnenberg, D. Williams-Young, F. Ding, F. Lipparini, F. Egidi, J. Goings, B. Peng, A. Petrone, T. Henderson, D. Ranasinghe, V. G. Zakrzewski, J. Gao, N. Rega, G. Zheng, W. Liang, M. Hada, M. Ehara, K. Toyota, R. Fukuda, J. Hasegawa, M. Ishida, T. Nakajima, Y. Honda, O. Kitao, H. Nakai, T. Vreven, K. Throssell, J. A. Montgomery, Jr., J. E. Peralta, F. Ogliaro, M. J. Bearpark, J. J. Heyd, E. N. Brothers, K. N. Kudin, V. N. Staroverov, T. A. Keith, R. Kobayashi, J. Normand, K. Raghavachari, A. P. Rendell, J. C. Burant, S. S. Iyengar, J. Tomasi, M. Cossi, J. M. Millam, M. Klene, C. Adamo, R. Cammi, J. W. Ochterski, R. L. Martin, K. Morokuma, O. Farkas, J. B. Foresman and D. J. Fox, Gaussian, Gaussian, Inc., Wallingford, CT, 2016.
- J. B. Brito, D. J. C. Gomes, V. D. Justina, A. M. F. Lima, C. A. Olivati, J. R. Silva and N. C. de Souza, J. Colloid Interface Sci., 2012, 367(1), 467–471 CrossRef CAS PubMed
.
- A. Dekshinamoorthy, P. P. Samal, S. Krishnamurty, P. K. Khatri, S. L. Jain, A. Ray and S. Vijayaraghavan, Langmuir, 2023, 39(48), 17295–17307 CrossRef CAS PubMed
.
- A. Tall, S. Faraji, A. K. Diallo, N. Mohammadian, M. Erouel, M. Seck, M. Saadi, K. Khirouni and L. A. Majewski, J. Mater. Sci.: Mater. Electron., 2022, 33, 15283–15295 CrossRef CAS
.
- M. Seck, N. Mohammadian, A. K. Diallo, S. Faraji, M. Saadi, M. Erouel, E. H. B. Ly, K. Khirouni and L. A. Majewski, Org. Electron., 2020, 83, 105 CrossRef
.
- A. Tall, A. K. Diallo, M. Erouel, M. Seck, L. Chouiref, M. Saadi, M. A. Wederni, E. H. B. Ly, A. Diallo, N. Bouguila, D. Kobor and K. Khirouni, J. Sol-Gel Sci. Technol., 2022, 104, 401–411 CrossRef CAS
.
- M. M. Zhou, W. Y. Zhang, R. J. Li, C. Guo, S. S. Wei, X. M. Tian, J. Luo and L. Y. Kong, Phytomedicine, 2018, 42, 152–163 CrossRef CAS
.
- H. Liu, A. T. Neal and P. D. Ye, ACS Nano, 2012, 6(10), 8563–8569 CrossRef CAS PubMed
.
- L. Li, Q. Tang, H. Li, W. Hu, X. Yang, Z. Shuai, Y. Liu and D. Zhu, Pure Appl. Chem., 2008, 80(11), 2231–2240 CrossRef CAS
.
- A. Sharma, M. Zahedi, M. Durmuş, T. Basova, A. Ray, V. Ahsen and N. Chaure, J. Appl. Phys., 2010, 107, 114503 CrossRef
.
- G. Chaidogiannos, F. Petraki, N. Glezos, S. Kennou and S. Nešpůrek, Appl. Phys. A: Mater. Sci. Process., 2009, 96, 763–767 CrossRef CAS
.
- O. A. Melville, B. H. Lessard and T. P. Bender, ACS Appl. Mater. Interfaces, 2015, 7(24), 13105–13118 CrossRef CAS
.
- H. Sajid, T. Mahmood and K. Ayub, J. Mol. Model., 2017, 23, 295 CrossRef PubMed
.
- P. Kumar, V. N. Mishra and R. Prakash, IEEE Sens. J., 2023, 23(12), 12544–12551 CAS
.
- S. Tyagi, M. Chaudhary, A. K. Ambedkar, K. Sharma, Y. K. Gautama and B. P. Singh, Sens. Diagn., 2022, 1, 106–129 RSC
.
- S. Forel, L. Sacco, A. Castan, I. Florea and C. S. Cojocaru, Nanoscale Adv., 2021, 3, 1582–1587 RSC
.
- X. Wang, M. Wei, X. Li, S. Shao, Y. Ren, W. Xu, M. Li, W. Liu, X. Liu and J. Zhao, ACS Appl. Mater. Interfaces, 2020, 12, 51797–51807 CrossRef CAS
.
- S. Oh, J.-I. Cho, B. H. Lee, S. Seo, J.-H. Lee, H. Choo, K. Heo, S. Y. Lee and J.-H. Park, Sci. Adv., 2021, 7, eabg9450 CrossRef CAS PubMed
.
- T. Ikuta, T. Tamaki, H. Masai, R. Nakanishi, K. Endo, J. Terao and K. Maehashi, Nanoscale Adv., 2021, 3, 5793–5800 RSC
.
- M. Mirza, J. Wang, L. Wang, J. He and C. Jiang, Org. Electron., 2015, 24, 96–100 CrossRef CAS
.
- Z. Yang, X. Zhuang, S. Han and J. Yu, Mater. Lett., 2019, 236, 285–288 CrossRef CAS
.
- Y. Kang, D. H. Kwak, J. E. Kwon, B.-G. Kim and W. H. Lee, ACS Appl. Mater. Interfaces, 2021, 13, 31910–31918 CrossRef CAS PubMed
.
- K. Zhao, X. Chang, J. Zhang, F. Yuan and X. Liu, ACS Sens., 2024, 9, 388–397 CrossRef CAS PubMed
.
- C. Wang, X. Chang, X. Liu and J. Zhang, J. Phys. Chem. Lett., 2024, 15, 5875–5882 CrossRef CAS
.
|
This journal is © The Royal Society of Chemistry 2025 |
Click here to see how this site uses Cookies. View our privacy policy here.