DOI:
10.1039/D4VA00136B
(Critical Review)
Environ. Sci.: Adv., 2025,
4, 189-222
Innovative approaches to sustainable wastewater treatment: a comprehensive exploration of conventional and emerging technologies
Received
14th May 2024
, Accepted 21st November 2024
First published on 22nd November 2024
Abstract
Access to clean water is under threat due to population growth, climate change, and pollution, emphasizing the need for effective wastewater treatment. Wastewater pollutants pose risks to public health and ecosystems, necessitating proper treatment methods. This paper outlines both conventional and emerging technologies for wastewater treatment. Established techniques, such as activated sludge processing, chlorination, and constructed wetlands, are discussed alongside newer methods, such as advanced oxidation, ultraviolet disinfection, membrane bioreactors, reverse osmosis, artificial intelligence optimization, and nanofiltration, which enhance contaminant removal but may incur high costs and energy demands. Integration of renewable energy sources, such as solar, wind, and biomass, into treatment facilities improves efficiency and reduces emissions. The process efficiency can be possibly enhanced through real-time monitoring and automation, while a sustainable and resource-efficient method involves integrating bio-electrochemical systems with constructed wetlands. There are still challenges in sludge handling, land requirements, and long-term system maintenance. Balancing technological solutions, environmental protection, and economic feasibility is essential for sustainable wastewater management, which can ensure continuous access to clean water in the face of increasing demand for this vital resource.
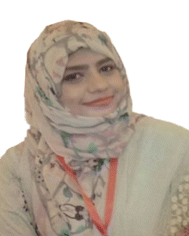 Jaweria Shamshad | Dr Jaweria Shamshad holds a PhD in Environmental Sciences from the University of the Punjab, Lahore. She completed the IPFP fellowship under the Higher Education Commission of Pakistan and conducts research on critical environmental issues. Her work primarily focuses on assessing the impacts of climate change on Pakistan's water resources, wastewater treatment, nanotechnology, and environmental chemistry. During her PhD, Dr Shamshad was awarded the IRSIP fellowship and the Indigenous scholarship. With over seven years of teaching and research experience, she possesses expertise in GIS, hydrological modeling, and related fields. Passionate about education and research, she actively shares her knowledge through her online platform, Global Geosoft. |
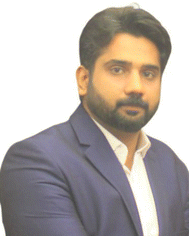 Rashid Ur Rehman | Dr Rashid Ur Rehman is a Chemical Engineer with extensive international research experience spanning Pakistan, China, South Korea, and Singapore. He is currently a Research Scientist at the Singapore Membrane Technology Centre, Nanyang Technological University, specializing in advanced membrane technologies for gas separation, water treatment, and environmental sustainability. Dr Rehman completed his PhD in Chemical Engineering at Nanjing Tech University in June 2019, where he conducted modern research on SAPO-34 zeolite membranes for wet gas separation. His expertise in developing innovative inorganic, polymeric and composite membranes as well as thin-film fabrication for CO2/N2 separation and ion-exchange processes is widely recognized through high-impact publications. |
Environmental significance
This work titled “Innovative Approaches to Sustainable Wastewater Treatment: A Comprehensive Exploration of the Conventional and Emerging Technologies” addresses pressing environmental concerns about wastewater treatment and its implications on sustainability. In the face of escalating threats to clean water access due to population growth, climate change, and pollution, effective wastewater treatment has become imperative to safeguard public health and preserve ecosystems. This comprehensive review provides a critical examination of a wide range of wastewater treatment technologies, spanning from established conventional methods to cutting-edge emerging approaches. By exploring techniques, such as activated sludge processing, chlorination, constructed wetlands, advanced oxidation, ultraviolet disinfection, membrane bioreactors, and reverse osmosis, this study illustrates the diverse landscape of options available for contaminant removal. In particular, this study highlights the integration of renewable energy sources, including solar, wind, and biomass, into treatment facilities as a means to enhance the efficiency and reduce environmental impacts. Furthermore, the discussion on real-time monitoring, automation, and controls underscores the role of digital integration in promoting sustainability, water quality, and cost-effectiveness in wastewater treatment processes. Despite the progress made in technological innovation, this study acknowledges persistent challenges in sludge handling, land requirements, and long-term system maintenance. These challenges underscore the need for a balanced approach to wastewater treatment management, which prioritizes environmental preservation, technological advancement, and economic feasibility. Overall, this study provides valuable insights into the discourse on sustainable wastewater management. By synthesizing technological advancements with environmental preservation strategies and economically viable solutions, it offers a holistic understanding of the challenges and opportunities in wastewater treatment. The findings presented in this study hold significance for environmental scientists, policymakers, and practitioners alike, as we collectively strive towards a more sustainable future.
|
1. Introduction
Water, crucial for life on Earth, is gaining global recognition as a valuable economic and social resource.1 Although water constitutes 71% of the Earth's surface, only 2.5% is pure, with merely 1% being easily accessible.2 Freshwater resources that are vital for sustaining life are at risk of being depleted due to population growth, industrialization, climate change, and its role in energy production.3 This scarcity poses a pressing environmental challenge, emphasizing the need for sustainable water management practices.4 Water quality is one of the 17 goals of the Sustainable Development Agenda 2030, highlighting the necessity of addressing this issue.5
Wastewater originating from domestic, commercial, agricultural, and run-off sources due to human activities primarily consists of 99.9% water content and some solids.6 The composition of wastewater, influenced by chemical components and flow conditions, is crucial in designing wastewater treatment plants, with seasonal variations impacting the flow conditions.7,8 The evaluation of wastewater quality involves the analysis of various chemical and material components, such as organic and inorganic substances.9 Key parameters assessed include BOD (biological oxygen demand), TSSs (total suspended solids), COD (chemical oxygen demand), TSs (total solids), VSs (volatile solids), TN (total nitrogen), TP (total phosphorus), pH, and alkalinity.10
Although access to sufficient quantities of high-quality water is fundamental to human survival, the escalating global population is putting immense strain on the planet's already scarce freshwater resources.1 It is anticipated that by 2050, there will be nine billion people on the planet, leading to elevated pressures on water and food resources.11 However, current urbanization and population increase faster than the measures taken to enhance the drinking water quality and wastewater treatment.12 The growing economy and population have increased water use and wastewater discharge, which has significantly increased pollution.13
Water pollution has far-reaching impacts on freshwater availability, ecology, and human health, necessitating comprehensive solutions.14 Human activities, even in modest doses, significantly alter landscapes, vegetation, and water quality processes, affecting the entire drainage basins.15 Managing materials released into land, water, and air requires proactive techniques due to lengthy removal timelines.16
Water scarcity and pollution have become urgent global issues, demanding innovative and sustainable strategies in managing wastewater.17 While traditional wastewater treatment methods are effective at removing certain contaminants, they often face challenges related to energy consumption, sludge production, and the treatment of new pollutants.4 As a result, there is a growing motivation to explore and adopt advanced technologies that can overcome these obstacles and promote environmental sustainability.18
Wastewater treatment is pivotal in maintaining high water quality standards, safeguarding human health, and preventing waterborne illnesses.19 By removing pollutants and toxins, treatment plants contribute significantly to public sanitation and safe drinking water.20,21 Additionally, wastewater treatment is crucial for preserving the ecological balance of aquatic environments.22 The repercussions extend beyond aquatic ecosystems, potentially harming land ecosystems through the water cycle.23,24
Although wastewater treatment technologies are a widely researched topic, there are still research gaps in the utilization of emerging technologies in sustainable wastewater treatment systems. While membrane filtration,25,26 advanced oxidation processes,27,28 and biological treatment systems29 are under investigation for wastewater reclamation, there is limited systematic research on their combined effectiveness in evolving wastewater treatment scenarios, as noted by Singh et al.30 Furthermore, exploring these technologies in decentralized wastewater treatment systems to address sanitation needs in rural and remote communities requires more attention.31,32 Additionally, Wang Yi, and colleagues have highlighted the lack of comprehensive utilization of artificial intelligence algorithms in wastewater treatment, particularly in conjunction with machine learning for real-time process optimization modeling.33 Addressing these knowledge gaps is crucial for developing innovative and efficient wastewater treatment plants.
This review examines a range of traditional and emerging wastewater treatment technologies, offering a thorough evaluation of their effectiveness, efficiency, and environmental impacts. The goal is to present a comprehensive view of the latest advancements in wastewater treatment, emphasizing the potential of novel approaches in achieving sustainable water management objectives. Through a detailed analysis of the strengths, weaknesses, and opportunities of various technologies, this review aims to educate policymakers, researchers, and industry professionals on the most promising solutions for addressing the complexities of wastewater treatment and resource recovery. Ultimately, the fusion of conventional and emerging technologies, supported by robust policy frameworks and sustainable practices, is crucial to ensure the long-term availability of water resources for future generations. The exploration of integrating new fields such as nanotechnology, artificial intelligence optimization, and biotechnology with traditional methods is crucial in this context. The review further presents an updated and distinctive outlook on sustainable wastewater management, examining factors such as environmental considerations, economic implications, and the challenges posed by emerging contaminants. Furthermore, the incorporation of examples showcasing successful applications of innovative techniques enhances the uniqueness of this work.
2. Research methodology
A systematic literature review methodology is used in this research project. An exhaustive computer-based search of academic databases was conducted to pinpoint studies discussing both traditional and cutting-edge wastewater treatment systems. Studies were selected based on the technology used and inclusion and exclusion criteria and were rigorously assessed for quality. Essential information was extracted from the technology table, including technology type, performance indicators, environmental considerations, and economic viability. Further, the compiled data were analyzed to identify the trends and potential areas for improvement through a critical evaluation against other effective practices. This systematic process aimed to conduct a thorough and evidence-based examination of sustainable wastewater treatment options.
3. Traditional wastewater treatment methods
3.1. Overview of conventional treatment technologies
Conventional techniques for treating wastewater, such as preliminary, primary, secondary, and tertiary treatment, are commonly used to remove pollutants from wastewater.34 These approaches serve the common goal of mitigating diverse contaminants including heavy metals, inorganic metallic materials, organic matter, residues from disinfection, and microbiological chemicals present in wastewater.35 Some applications of treated wastewater are shown in Fig. 1.
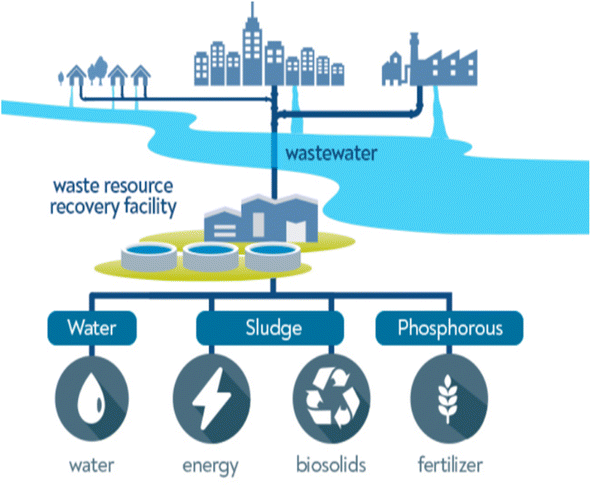 |
| Fig. 1 Stages and applications of wastewater treatment (ref. 36). | |
Following steps are taken to purify water in the conventional wastewater treatment process, as shown in Fig. 2.
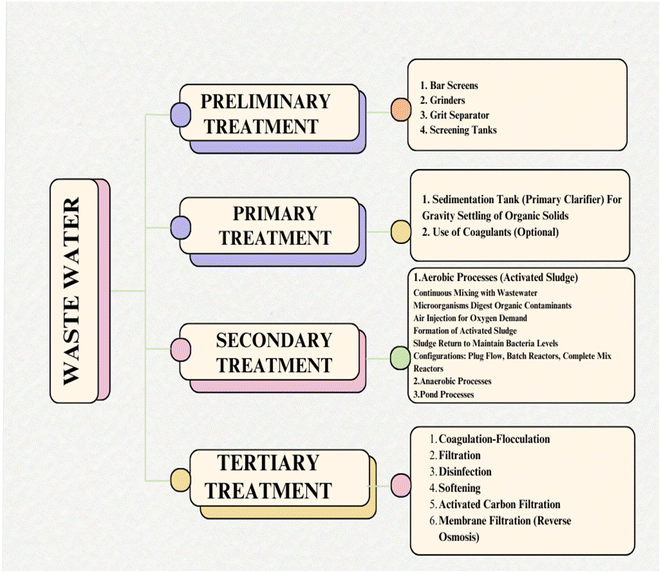 |
| Fig. 2 Overview of wastewater treatment processes. | |
(1) Preliminary treatment (screening): preliminary treatment involves the initial removal of large solids and debris from the wastewater, typically through processes such as screening and grit removal. This stage aims to protect downstream treatment processes and equipment from damage or clogging.
(2) Primary treatment: primary treatment removes suspended solids and organic matter from wastewater via processes such as sedimentation and flotation, which allow the solids to settle or float to the surface for removal. Primary treatment also helps to reduce the organic load and turbidity of the wastewater before further treatment.
(3) Secondary treatment (activated sludge process and trickling filters): secondary treatment includes biological treatment techniques such as sequencing batch reactors, trickling filters, and activated sludge processes.37 By using microbes to decompose organic materials, these methods significantly enhance the removal of pollutants.38
Tertiary treatment (chlorination and filtration): some wastewater treatment plants use tertiary treatment to further purify water quality.39 Filtration, chemical treatment, and advanced biological treatment technologies are used in this step. Using disinfection techniques including ozonation, UV irradiation, and chlorination, remaining germs and bacteria are removed.40 For hygienic, safe wastewater production, digestion, dewatering, and incineration techniques are important to reduce sludge and improve disposal or reuse.41
A cost-effective approach to wastewater treatment includes separating solid waste and sludge, purifying water through aeration and sedimentation, and employing constructed wetlands.42 These natural systems are highly effective in removing pollutants, and the treated water can be used for irrigation and agricultural purposes, especially in regions prone to drought.43 For a comparative analysis of traditional wastewater treatment methods, see Table 1.
Table 1 Comparison of conventional methods for treating wastewater
Method |
Description |
Advantages |
Disadvantages |
Energy consumption and cost |
Sludge production |
Ref. |
Primary treatment |
Screening and sedimentation to remove large solids residuals |
Simple, low cost, removes large solids |
Poor performance for dissolved pollutants, sludge generation |
Low |
High |
44
|
Secondary treatment |
Biological treatment to remove organic matter and suspended solids. Includes activated sludge, trickling filter, and lagoon systems |
High removal efficiency for organic matter, nutrient removal |
Requires skilled operation, susceptible to shock loads, sludge production |
Moderate to high |
High |
45
|
Tertiary treatment |
Advanced treatment to remove remaining nutrients, pathogens, and suspended solids. Includes filtration, disinfection, and chemical precipitation |
High effluent quality, removal of specific pollutants |
High cost, energy intensive, complex operation |
High |
Moderate |
46
|
3.2. Limitations of traditional methods
Primary methods may not achieve complete eradication of contaminants including microbial compounds, heavy metals, inorganic metallic debris, and disinfection byproducts found in wastewater.34,47 Furthermore, these conventional techniques require substantial space for the establishment of treatment plants and can incur considerable costs.48 Conventional methods have failed in the separation of emerging contaminants, persistent organic pollutants, and certain recalcitrant substances.49 Concerns arise over the impacts of conventional technologies on the environment like the energy-intensive aeration in activated sludge systems.50 The adaptability of conventional treatment methods is crucial to handle varying in-fluent compositions, especially potential strain from fluctuations in industrial discharges.51 The reliance on large-scale infrastructure and substantial land requirements limits their suitability in densely populated urban areas. Dealing with potential large volumes of residual sludge requires proper disposal or resource recovery strategies.52
3.3. Need for technology advancements
The evolving range of contaminants in wastewater emphasizes the need for continuous innovation and the integration of advanced treatment methods.53 Prioritizing research and development in the ongoing discourse on wastewater management is essential to overcome these limitations and ensure treatment systems' resilience in response to emerging environmental concerns.54 Consequently, there is a growing demand for alternative methods that offer enhanced efficiency, cost-effectiveness, and environmental sustainability.30 Stringent regulations and hefty fines for violating wastewater discharge limits drive the advancement of cutting-edge treatment methods in the industrial sector.55
4. Role of technology in wastewater treatment
Technology is crucial in treating wastewater by offering effective and sustainable approaches to eliminate impurities and contaminants from water sources.56 Numerous techniques including chemical, physical, and biological processes are utilized in wastewater treatment.57 One such technique involves the utilization of algae for phytoremediation, whereby algae are cultivated in wastewater to eradicate contaminants and generate biomass for the production of biofuel.58 Moreover, the utilization of biochar and green nanoparticles derived from agricultural waste exhibits promising potential in eliminating persistent pollutants from water and wastewater.59 Membrane technology and nanotechnology offer promising prospects in wastewater treatment including carbon nanostructures and nanofilters.60 Surface-modified carbon nanotubes improve heavy metal adsorption in wastewater treatment, where technology selection depends on parameters such as chemical oxygen demand (COD), total solids (TSs), volatile solids (VSs), and customized solutions to address specific issues.10,11 Similarly, wastewater that contains metal and cyanide compounds goes through a single oxidation phase that is carefully planned and executed using an alkaline reagent and a chlorine solution.12
Anaerobic and aerobic methods, known for their eco-friendliness and cost-effectiveness, particularly the low-energy-consuming anaerobic technologies, have gained popularity for treating organic wastewater.59 Various alternatives including membrane-based procedures, ion exchange, electrochemical treatment, adsorption, biological treatment, Fenton processes, coagulation, flocculation, and UV-based processes have been strategically employed to address these inherent limitations.61 While these modern methods present promising solutions for the removal of pollutants from wastewater, it is imperative to acknowledge and systematically address the remaining challenges and limitations associated with their applications.30
Novel approaches for wastewater treatment encompass a range of methods, including the generation of ozone through water electrolysis or corona discharge, electrocoagulation, hybrid techniques, nanotechnology, and membrane technology.62 The primary goal of these technologies is the elimination of toxic contaminants and pollutants in wastewater, such as viruses, bacteria, heavy metals, pharmaceuticals, hormones, synthetic dyes, and flame retardants.56 This involves employing physical, chemical, and biological methods such as membrane filtration, adsorption, coagulation–flocculation, solvent extraction, ion exchange, photo degradation, catalytic oxidation, electrochemical oxidation, and precipitation, as shown in Fig. 3.63 The integration of nanomaterials into these methods has efficiently eliminated various contaminants.64 However, the significant energy consumption associated with these technologies can lead to substantial costs.65 The true objective is to develop wastewater treatment plants capable of completely and effectively eliminating emerging contaminants (ECs). Ongoing research is dedicated to enhancing the efficiency, effectiveness, and sustainability of these treatment methods.25,27,28
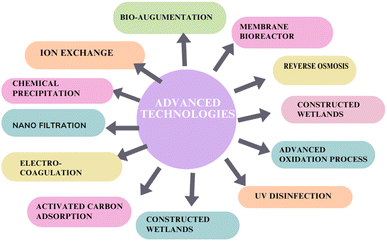 |
| Fig. 3 Advanced wastewater treatment technologies. | |
Innovative initiatives have resulted in the development of a wastewater treatment method that combines biological treatment with aerobic microorganisms and ozone sterilization seamlessly.66 Although these approaches have great potential for treating wastewater and removing pollutants, it is crucial to recognize that the unique approach has different benefits and drawbacks. In summary, technology is a critical driver in advancing sustainable and practical approaches to wastewater treatment.
4.1. Membrane technologies
A membrane is a semi-permeable barrier that controls the transport of substances between two adjacent phases.67 Due to their intrinsic properties, membranes are playing an important role in the separation of science and new engineering approaches toward industry.68 Membrane technology is an advanced separation process that utilizes semi-permeable membranes to selectively transport or reject substances between different phases such as liquids or gases.69 This technology has gained prominence across various industries including water treatment, pharmaceuticals, biotechnology, and environmental protection.70,71 Operating on the principle of filtration, membrane technology allows for the separation of particles based on size, where the membrane's pore size is smaller than the contaminants to be removed, such as microorganisms or pollutants.72 This process is often more energy-efficient than the traditional thermal separation methods such as distillation and can operate effectively at lower temperatures, making it suitable for heat-sensitive materials.73
There are several types of membrane processes, each tailored to specific applications.74,75 Microfiltration (MF) is designed to remove larger particles such as suspended solids and bacteria, while ultrafiltration (UF) targets smaller particles including proteins and colloids.76,77 Nanofiltration (NF) is effective for divalent ions and small organic molecules, and reverse osmosis (RO) can eliminate nearly all contaminants, including monovalent ions and small molecules, requiring high pressure for effective separation.78 The choice of membrane process depends on the desired purity of the permeate and the characteristics of the feed solution.79Fig. 4 illustrates the types of membranes on the basis of pore size for specific removal applications.
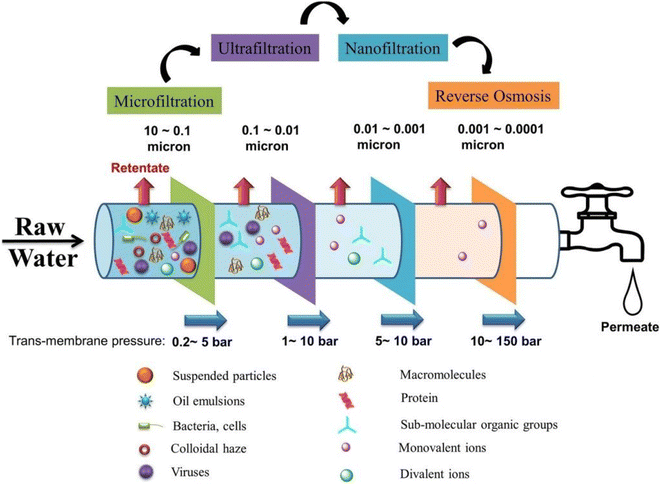 |
| Fig. 4 Illustrating the role of membrane technologies in wastewater treatment (Copyright Nordic membrane 2012–2019).80 | |
In the realm of water and wastewater treatment, membrane technology has emerged as a preferred method for reclaiming water and treating wastewater.81 Its high separation efficiency allows for the effective removal of contaminants, making treated water suitable for various applications including agricultural and industrial uses.82,83 Additionally, membrane technology is instrumental in producing drinking water via processes such as reverse osmosis, which ensures the removal of harmful substances.84
Beyond water treatment, membrane technology plays a crucial role in environmental protection, particularly in reducing pollutants.85 It is employed in applications such as air pollution control and the treatment of gas-phase organic pollutants.86 The ability to operate without chemical additives makes membrane technology an environmentally friendly option compared to conventional methods, contributing to sustainable practices.87 In medical applications, membrane technology is vital for processes such as hemodialysis, where it is used to remove toxins from the blood. Furthermore, artificial lungs utilize membranes to facilitate oxygen transfer without the formation of bubbles, highlighting the technology's importance in healthcare and its potential to improve patient outcomes.88,89 Despite its numerous advantages, membrane technology faces challenges, particularly related to membrane fouling, which can reduce efficiency and increase operational costs.89 Ongoing research aims to develop fouling-resistant membranes and improve cleaning techniques to enhance the longevity and performance of membrane systems.90 Additionally, there is a growing focus on integrating membrane processes with other treatment technologies to create hybrid systems that maximize efficiency and effectiveness in wastewater treatment.91 Overall, membrane technology is a versatile and efficient method for separation processes, with significant potential for addressing environmental challenges and improving water management practices.91
A driving force, such as a semipermeable barrier, controls the rate of component movement through fractional permeation and rejection via pores of varying sizes in a typical membrane mechanism.92 This approach is frequently used in mechanical processes for separating gas or liquid streams. Membranes function as thin barriers for size differential separation, often integrated with chemical and biological treatments or utilized as standalone systems in secondary wastewater treatment.93,94
Extensive research has been conducted to investigate the application of membrane technology for wastewater treatment. Studies95,96 have identified the potential of various membrane technologies, such as RO, MF, UF, and NF, in treating water produced by oil fields and refineries, as well as in removing pollutants from wastewater.
Various advanced wastewater treatment technologies have emerged, encompassing membrane technology,96 along with adsorption, coagulation, advanced oxidation, and magnetization–biomimetic enzyme condensation.97 The utilization of nanotechnology, as explored by,98 has further contributed to enhance the treatment efficiency. Within the chemical industry, prevalent methods include physio-chemical and biological processes, constructed wetlands, and advanced oxidation processes.99 These diverse technologies present promising solutions for wastewater treatment.
Gray water, textile effluents, paper mill wastewater, pharmaceutical wastewater, and hospital effluents are a few industrial wastewater streams treated efficiently by membrane technologies.100 These advanced treatment methods can also remove a wide range of contaminants and clean the water for potential discharge.101
The gray water, which is slightly polluted wastewater from sinks, showers, and washing machines, can be effectively treated using a combination of membrane bioreactors (MBRs) and plastic tube ultrasonic welding machine of reverse osmosis (RO).102 After treatment, the water is separated from biomass through a membrane filtration process in MBR.103 Subsequently, the purified water undergoes further treatment to remove salts and organic matter using RO.104 This hybrid setup ensures that the water meets strict quality standards suitable for various reuse options such as toilet flushing, irrigation, and other non-potable uses.105
Membrane technology combinations are also employed to treat textile industry effluents containing high color, COD, and toxicity.106 The textile industry is a large contributor to water pollution because of the dyes and dangerous chemicals that are dumped into local rivers during production. These contaminants are not easily removed by conventional treatment methods resulting in the exploration of alternative materials such nanofibers. Nanofiltration (NF) and reverse osmosis (RO) processes are utilized to remove dyes, salts, and organic compounds from the textile effluent.107 The implementation of pretreatment procedures such as coagulation or adsorption can help decrease the membrane fouling potential and enhance the overall treatment efficiency.108 The high surface area and easy adsorption nature of nanofibers are considered excellent for treating textile wastewater.109 A study has shown the potential of this approach, where electrospun nanofibers made from thermoresponsive polymers have localized adsorption sites for hydrophobic organic compounds and removed over 90% dyes from distinct populations by selecting sub-optimal dye concentrations.110 Although this technique is versatile, and it allows the production of nanofibers with diameters from 300 to 500 nm, which can be prepared using different polymers such as polyacrylonitrile (PAN) or even incorporating TiO2 in their structure for better dye degradation under UV light, they are frequently linked to electrostatic issues during fiber extension leading unwanted branches on very uniform fibers interfering mainly at interfacial region dye interaction causing adsorption promotion.110 The dual responsivity exhibited by these polymers allows for receptor adaptations to the surrounding conditions, such as temperature or pH.111 The dye removal process can be further accentuated through tuning polymer properties. A copolymer of N-isopropylacrylamide and dimethylaminoethyl methacrylate efficiently removes acidic as well as basic dyes from waste water, which is highly applicable for textile effluent treatment.112 The application of nanofibers for textile wastewater is encouraging in that it enhances the efficiency level of dye removal and aids water resource sustainability.113 By introducing nanofiber technology within the current treatment systems, textile manufacturers reduce their environmental footprint substantially while enabling them to comply with strict regulations on wastewater discharge.114 Moreover, the possible reuse of nanopowders for dye adsorption is economically important because this helps it in providing a low-cost solution, which can be useful for wastewater management among textile industries.115
Another study presents the development and application of novel smart composite materials for the simultaneous removal of organic impurities and toxic heavy metals from industrial wastewater.116 These materials demonstrate high removal efficiencies, reusability, and significant reduction in chemical oxygen demand (COD) levels, making them promising candidates for industrial water remediation and reuse. The smart composite materials are fabricated using a combination of synthetic and natural polymers such as pullulan, which exhibit a temperature-responsive behavior. The materials can efficiently extract organic contaminants such as phenols, anhydrides, textile dyes, pesticides, herbicides, and antibiotics, as well as inorganic heavy metals, from wastewater. The removal efficiency exceeds 90% at optimal concentrations. The high reproducibility in synthesis, properties, and elimination spectrum of these smart composite materials sets them apart as innovative solutions for industrial wastewater treatment. The established 4-cycle reusability and substantial reduction in COD levels further highlight their potential for practical applications in water reuse.
Regarding wastewater from the paper industry, it is characterized by high concentrations of organic matter, suspended solids, and nutrients, which can be effectively treated using a combination of membrane filtration and biological processes.117,118 Ultrafiltration (UF) is responsible for the removal of suspended solids and colloidal matter, while reverse osmosis acts as a post-treatment process to remove dissolved salts and organic compounds.119,120 The treated water can then be reused in various processes within the paper mill to eliminate freshwater usage and reduce the environmental footprint.121
Advanced treatment methods are necessary for the removal of contaminants found in pharmaceutical and hospital wastewaters, which include organic micro pollutants, antibiotics and pathogens.27 These methods typically involve membrane technologies (such as UF or RO), advanced oxidation processes (AOPs), and/or activated carbon adsorption.122 The treated water can then be discharged or reused in compliance with specific requirements and regulations.123
Additionally, some scientists124,125 have highlighted the advantages of membrane bioreactors, which integrate activated sludge treatment with membrane filtration for biomass retention. This approach proves beneficial in the treatment of industrial, domestic, and food processing waste. Collectively, these studies emphasize the promising role of membrane technology in enhancing the efficiency and sustainability of wastewater treatment processes.
4.1.1. Reverse osmosis.
Wastewater treatment utilizes RO technologies to eliminate impurities and contaminants.126 RO membranes play a crucial role in this process, but their efficiency can be compromised by the elevated salinity and organic content found in wastewater.127 To enhance RO performance, nanofiltration membranes are a viable option, reducing wastewater flow and operational costs.128 An alternative method involves employing forward osmosis (FO), a technique with promising applications in water and wastewater treatment. Ongoing research is focused on optimizing FO membranes and draw agents to enhance the overall process.129
However, to treat high salinity or streams that are prone to fouling, the FO process is proving to be an innovative water technology with a lot of potential applications.130 Among its many benefits are its low hydraulic pressure requirements, efficient rejection of solutes and contaminants, and improved fouling resistance in comparison to membrane technologies that are pressure-driven.35,36
In addition to these technologies, phytoremediation, a plant-based approach, offers an eco-friendly and cost-effective means of treating RO wastewater, converting it into useable water.131 Reverse osmosis wastewater (ROWW) contains high levels of salts, heavy metals, and pollutants, making it unsuitable for use.132 Nevertheless, a solution that is both environmentally friendly and economic involves employing phytoremediation—a plant-based method capable of converting ROWW into useable water.133
Diverse phytoremediation techniques such as phytodegradation, phytoextraction, phytoremediation, and phytovolatilization can be efficiently utilized to improve the quality of ROWW. Recent research efforts have been dedicated to utilize plants to purify ROWW, revealing promising results in the advancement of water quality.134
FO is suggested as a method for providing fertilizing solutions to plants, using brackish groundwater as the input solution and calcium ammonium chloride as the extraction solution.135 NF is employed for draw solution regeneration, but high salinity groundwater requires additional post-treatment to reduce nutrient concentrations before applying the fertilizing solution to crops.136 Pilot-scale assessments of FDFO-NF (Fertilizer drawn forward osmosis-nanofiltration) using coal mining saline groundwater as the draw solution aimed to produce irrigation water meeting standards. Among tested solutions, ammonium sulfate exhibited the highest water recovery rate, KH2PO4 showed the highest water flux recovery, and ammonium phosphate monobasic had the lowest final nutrient concentration.11
Mohammed et al. explored a novel approach to enhance polyamide (PA) membranes for desalination and water treatment by modifying the support layer with Y-type zeolites. The resulting PA membranes, formed via interfacial polymerization of piperazine (PIP) and 1,3,5-benzenetricarbonyl trichloride (TMC), achieved a water transport rate of 22.5 ± 2.2 L m−2 h−1 bar−1 and high salt rejection rates (over 99% for MgCl2, MgSO4, and Na2SO4). This work demonstrates the effectiveness of zeolite modification in improving nanofiltration performance. The SEM and AFM characterization of membranes and pure water permeance and rejection results are presented in Fig. 5 and 6 respectively.137
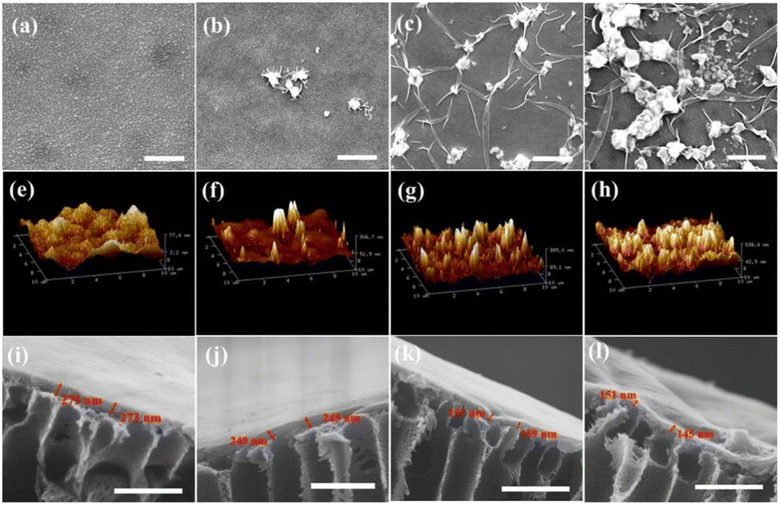 |
| Fig. 5 SEM photographs of all membranes: (a) M-0, (b) M-50, (c) M-100, and (d) M-150. AFM images: (e) M-0, (f) M-50, (g) M-100, and (h) M-150. Cross-sectional photographs of (i) M-0, (j) M-50, (k) M-100, and (l) M-150. (Copyright, Nature 2023), ref. 137. | |
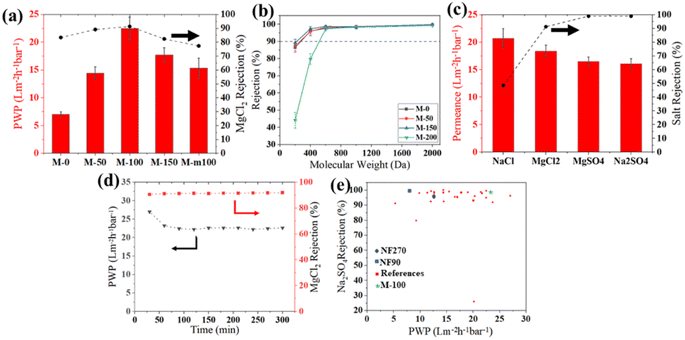 |
| Fig. 6 (a) TFC membrane permeance and rejection for pure water with MgCl2 (1000 ppm), (b) PEG rejection performance, (c) salt solution permeance and rejection towards NaCl, MgCl2, MgSO4, and Na2SO4 with M-100, (d) long-term performance of M-100 and (e) comparison with recently published PIP/TMC membranes tested with 1000 ppm Na2SO4 feed solution (Copyright, Nature 2023) ref. 137. | |
Another study presents a novel nanocomposite membrane for simultaneous oil/water separation and desalination of oily, saline wastewater. The synthetic process and work mechanisms of the hydrogel/GO FO membrane are shown in Fig. 7. The membrane features an oil-repelling, salt-rejecting hydrogel selective layer on a graphene oxide-infused polymeric support. Compared to commercial membranes, this design achieves over three times higher water flux with >99% removal of oils and multivalent ions, making it highly effective for treating challenging wastewaters. The schematic diagram of simultaneous oil/water separation and desalination by the prepared membrane is given in Fig. 8.138
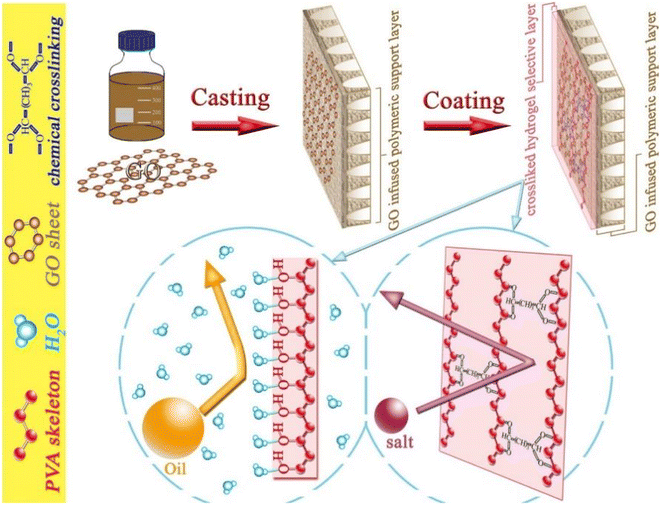 |
| Fig. 7 Illustration of the synthetic process and work mechanisms of the Hydrogel/GO FO membrane (Copyright Nature 2015) ref. 138. | |
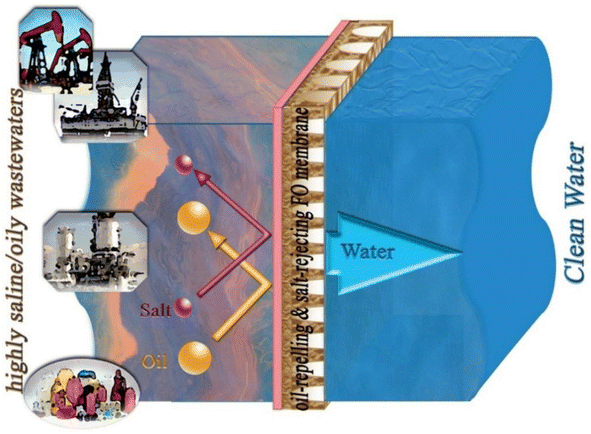 |
| Fig. 8 Schematic of simultaneous oil/water separation and desalination by hydrogel/GO FO membrane (Copyright Nature 2015) ref. 138. | |
4.1.2. Nanofiltration.
Nanofiltration (NF) emerges as a promising, efficient and cost-effective methodology in wastewater treatment, presenting advanced approaches to eliminate both organic and inorganic molecules from water.139 In diverse applications, NF membranes are employed to selectively eliminate ions and organic compounds, doing water softening, color removal, disinfection of by-products, seasonal fouling, and heavy metal removal.140 Specifically, polyether sulfone (PES) nanofiltration membranes stand out for their capacity to effectively remove toxic metal ions from water, owing to their robust thermal, chemical, and mechanical properties.141
For industrial applications such as bio-refinery and petrochemical processes, solvent-resistant nanofiltration (SRNF) membranes have been developed.139 These membranes exhibit stability and excel in efficiently separating organic compounds even under extreme conditions.142
Laurell et al. developed loose nanofiltration (LNF) membranes to effectively remove natural organic matter (NOM) from Finnish surface water (Fig. 9). One LNF membrane achieved over 95% NOM rejection with a 40% hardness rejection rate. While operational costs could increase by 53–69%, environmental impacts may decrease by over 18%. Improved removal of low-molecular-weight compounds could further reduce chlorine usage and costs.143
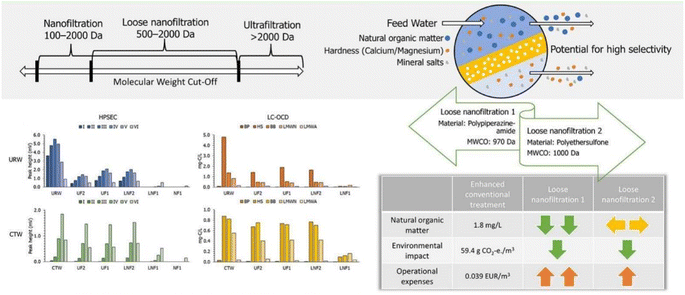 |
| Fig. 9 Illustration of nanofiltration membranes for surface water treatment by Laurell et al. (Copyright 2024) ref. 143. | |
Ghanbari et al. developed Ti3C2Tx MXene@metal–organic framework nanosheets for thin-film polymer membranes (Fig. 10), achieving high salt rejection rates (98.6% for Na2SO4) and a threefold increase in permeation rate (17.1 L m−2 h−1 bar−1). The membranes also rejected over 95% of heavy metal ions and showed a 95.3% flux recovery rate, with long-term performance maintaining 91.5% of the initial permeation rate.144
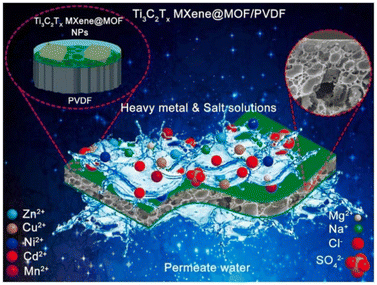 |
| Fig. 10 Schematic of the preparation of MXene/MOF-PVDF nanocomposite membrane (Copyright Elsevier 2023) ref. 144. | |
Another study presents a composite membrane of polyaniline (PANI) and Ti3C2Tx (MXene) that significantly reduces fouling (Fig. 11). The MXene-PANI/PES membrane achieves a 200.9% increase in pure water flux, over 99% retention of bovine serum albumin, and high dye rejection rates. With a conductivity of 0.5 S m−1, applying a negative voltage results in a 93.7% flux recovery rate, showcasing its potential as an efficient anti-fouling conductive membrane.145
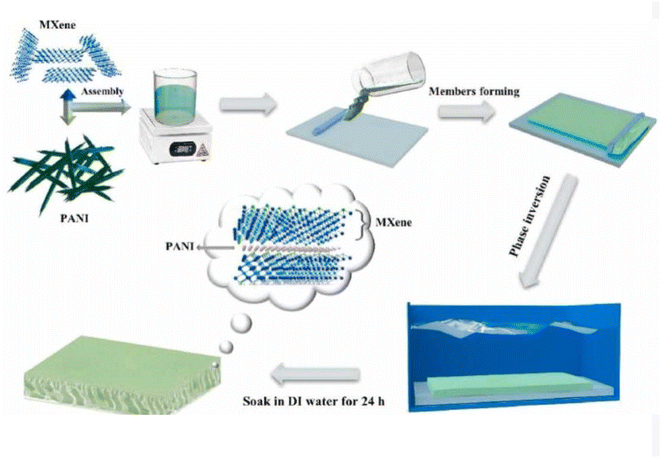 |
| Fig. 11 Synthesis of polyaniline (PANI) and Ti3C2Tx (MXene) composites (Copyright Elsevier 2023) ref. 145. | |
Zhao et al. develops a nanofiltration membrane featuring fast permeation (105 L m−2 h−1 bar−1), high Na2SO4 rejection (99.4%), and Cl−/SO42− selectivity (130). Utilizing graphitic carbon nitride (g-C3N4) to control interfacial polymerization, the membrane's nanoscale-ordered hollow structure enhances performance, making it superior for water purification and desalination applications.146 The obtained results are presented in Fig. 12.
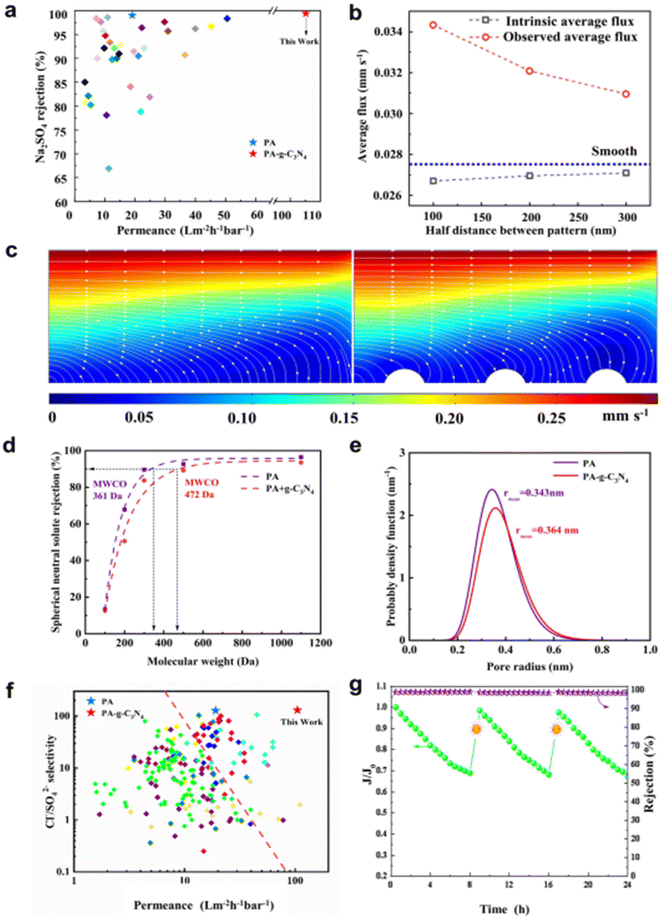 |
| Fig. 12 Separation results for NF membranes. (a) Performance comparison of PA-g-C3N4 and NF membranes, (b) average flux of various half distances among patterns, (c) comparison among flow streamlines of smooth and nanoscale-ordered structures, (d) MWCOs of the PA and the PA-g-C3N4 membrane, (e) mean pore size of the PA and the PA-g-C3N4 membrane, (f) trade-off between Cl−/SO42− selectivity and water permeability of different NF membranes, (g) flux decline and rejection of the PA-g-C3N4 membrane with methylene blue as the feed solute; cleaning was done with visible light. (Copyright Nature 2023) ref. 146. | |
Zeng et al. develops a polyether sulfone (PES) membrane with MXene@TiO2 heterojunctions (MXTM) for oily wastewater treatment (Fig. 13). The membrane achieves a high water flux of 3045 L m−2 h−1 bar−1 and over 99.8% oil/water separation efficiency. It also exhibits exceptional hydrophilicity and self-cleaning properties, maintaining a flux recovery rate of >98% over 10 cycles using visible light for photodegradation. This innovative membrane highlights the potential of MXene-based materials for effective oily wastewater treatment.147
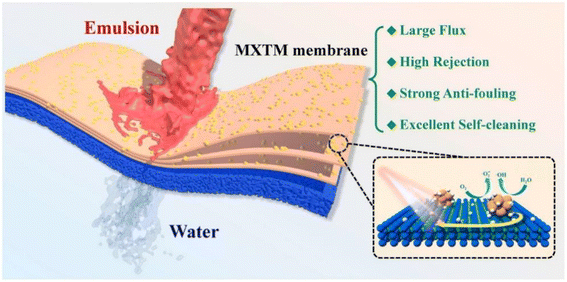 |
| Fig. 13 Polyether sulfone (PES) membrane with MXene@TiO2 heterojunctions (MXTM) for oily wastewater treatment (Copyright Elsevier 2024) ref. 147. | |
4.1.3. Ultrafiltration technologies.
Ultrafiltration (UF) technologies for wastewater treatment have a number of benefits.148 UF can efficiently remove natural organic matter (NOM) from raw water, which is commonly found in all types of water sources used for freshwater production.41 UF is also an affordable method of treating surface water which makes it appropriate for treating wastewater and sea water.42
The water treatment ultrafiltration membrane assembly comprises multiple systems, for effective purification: a plate surface type system, a capillary tube type system, a tubular system, and a uniform dispersion detection system.83 Physical methods are used for fire extinguishing and sterilization. In the tubular system, bacteria are effectively eliminated by heating the water flow with enough stirring.149 In the plate surface-type system, the direction of the water flow is controlled, and all-around UV irradiation sterilization is applied, ensuring a high degree of water sterility.150 The physical operations involved have a short operation time, are generally harmless to humans, and do not significantly increase costs.151 Several operating modes in the tubular system, including aeration, stirring, and impacting, contribute to attaining these effects.152 The technique enables thorough stirring prior to filtration, improving the distribution of inclusions in the water for improved filtering effects.43 UF membranes can also be used in industries such as textile, dairy, beverages, microelectronics, petrochemicals, cosmetics, and pharmaceuticals because of their versatility and capacity to separate and purify different substances.44
In summary, UF technologies offer benefits such as efficient natural organic matter (NOM) removal, cost-effectiveness, and versatility for various industries.153 However, membrane fouling and the scalability of membrane enhancement solutions are important considerations in the application of UF for wastewater treatment.151,154 Moreover, while membrane enhancement solutions show promise, their scalability and economic feasibility remain uncertain.152,155 Although chemical cleaning techniques can effectively reduce membrane fouling, they also run the risk of damaging membranes.45
Yang explores the combined use of powdered activated carbon (PAC) and ozone (O3) pretreatment for ultrafiltration (UF) performance. The 100PAC-5O3 process significantly reduced reversible and irreversible fouling resistance by 82.89% and 58.17%, respectively, and outperformed O3-PAC in degrading natural organic matter (Fig. 14) The findings highlight PAC-O3's potential for enhancing surface water treatment and controlling membrane fouling156 (Fig. 14).
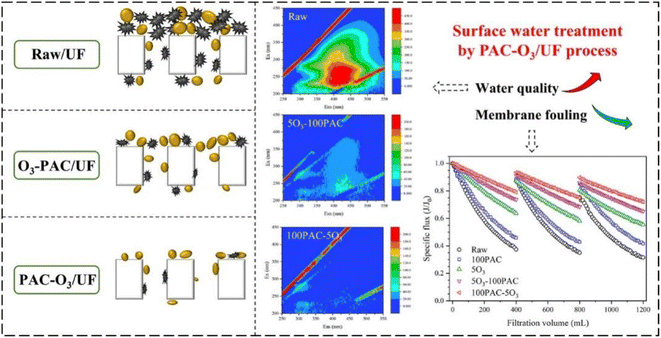 |
| Fig. 14 Comparison of Raw/UF, O3-PAC/UF and PAC-O3/UF's potential for enhancing surface water treatment and controlling membrane fouling (Copyright Elsevier 2023) ref. 156. | |
Liu investigates Fe(II)/PAA (Per Acetic Acid) as a pretreatment for ultrafiltration of secondary wastewater. Fe(II)/200 μM PAA reduced fouling resistance by 90.2% and effectively removed >85% of organic micropollutants and improved phosphorus removal. The process also enhanced nitrogen removal by producing biodegradable byproducts. Fe(II)/PAA shows strong potential for improving water quality in secondary effluent treatment (Fig. 15).157
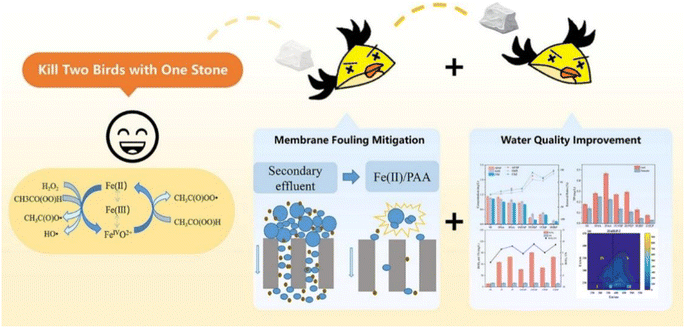 |
| Fig. 15 Representation of Fe(II)/PAA ultrafiltration setup for wastewater treatment (Copyright Elsevier 2023) ref. 157. | |
Ma et al. developed a PEI (polyethylenimine)-modified PES (Poly Ethylene Sulfone) ultrafiltration membrane with a 110 kDa cut-off to enhance the removal of humic acid and copper presented in Fig. 16. Despite reduced flux, the membrane achieved high removal efficiencies due to smaller pores and adsorption. Aluminum coagulants outperformed iron, with better performance at higher dosages. The modified membrane maintained excellent removal after storage and cleaning cycles. This approach shows strong potential for improving water treatment processes.158
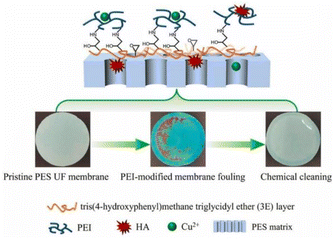 |
| Fig. 16 PEI-modified PES ultrafiltration membrane (Copyright Elsevier 2023) ref. 158. | |
Diallo et al. evaluated three ceramic ultrafiltration membranes (0.02, 0.05, 0.1 μm) for recovering secondary effluent (Fig. 17). The 0.02 μm membrane (UF1) outperformed the others, achieving 75% COD, 72% BOD5, and 96% TSS removal under optimal conditions (1 bar, 1 m s−1). UF1 also retained over 90% of microorganisms, removed 100% of helminth eggs, and reduced TKN by 14% and TP by 50%. The results demonstrate UF1's strong potential for improving water reuse from secondary effluents.39
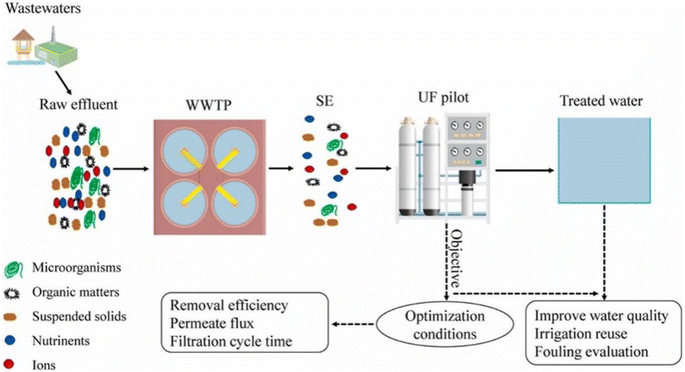 |
| Fig. 17 Ceramic ultrafiltration membranes (0.02, 0.05, 0.1 μm) for recovering secondary effluent (Copyright Elsevier 2024) ref. 39. | |
Ultrafiltration (UF) systems effectively remove particles and organics from water without affecting pH, while conventional water treatment plants (WTPs) can lower pH due to coagulants. Raw water usually has a near-neutral pH of 6.70 to 7.51. UF offers superior contaminant removal and stable pH compared to traditional methods159
4.2. Advanced oxidation processes (AOPs)
The utilization of strong oxidizing agents, to rapidly and effectively degrade organic and inorganic contaminants in wastewater, is what makes advanced oxidation processes (AOPs) an eco-friendly and efficient wastewater treatment technology160. These processes are for the treatment of pollutants that are resistive to traditional treatment technologies.161 The advantages of AOPs include their ability to treat a wide range of pollutants, potential for complete mineralization, and ability to degrade persistent pollutants.162 However, AOPs also have some drawbacks such as high energy requirements, the need for catalysts or chemicals, and the potential formation of harmful byproducts.163,164 AOPs can effectively mineralize or degrade contaminants into non-toxic end products, making them suitable for various wastewater effluents.165
Pharmaceutical compounds (PCs) in water systems pose significant ecological risks, and conventional treatment methods often fail to remove them.166 Integrated adsorption/advanced oxidation process (AOP) systems effectively remove pollutants from wastewater by combining adsorption and AOP, benefiting from simple design, mild conditions, and low costs. The process involves two stages: first, a solid adsorbent captures pollutants, reducing their concentration.167 Next, AOP treatment generates reactive oxidizing species that break down remaining pollutants into less harmful byproducts such as carbon dioxide and water.168 The effectiveness of these systems depends on factors such as the type of adsorbent, the characteristics of the contaminants, and the AOP technique used.169 Overall, integrated adsorption/AOP systems enhance wastewater treatment efficiency and improve the removal of organic contaminants and pathogen (Fig. 18).170
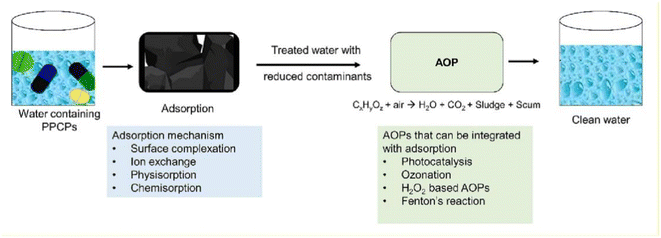 |
| Fig. 18 General mechanism of integrated adsorption/AOP systems (Copyright Elsevier 2023) ref. 170. | |
Yang et al.171 developed graphitic carbon nitride (g-C3N4)-based catalysts that are promising for advanced oxidation processes (AOPs) in water treatment, particularly in Fenton-based processes, catalytic ozonation, and persulfate activation. Recent research highlights their catalytic performance, mechanisms, and the influence of water chemistry factors such as pH and organic matter. While much focus has been on photocatalysis, there is increasing interest in their broader applications, offering both challenges and opportunities for future developments.
Silva et al. evaluated advanced oxidation processes (AOPs), UV-C/H2O2 and UV-C/chlorine, for removing 14 contaminants of emerging concerns (CECs) from municipal secondary effluent (MSE) and its nanofiltration retentate (NFR) (Fig. 19). Their results indicated that UV-C effectively removed CECs in MSE but was less effective in NFR. The addition of 10 mg L−1 of H2O2 or Cl2 enhanced performance, with UV-C/H2O2 being more effective than UV-C/chlorine. Both AOPs eliminated chronic toxicity in MSE towards Chlorella vulgaris, but toxicity persisted in NFR, especially with UV-C/chlorine due to toxic by-products. The nanofiltration permeate (NFP) had low CECs and microbial content, and a single chlorine addition effectively controlled E. coli regrowth for three days, indicating potential for safe crop irrigation reuse. Overall, the findings highlight the effectiveness and limitations of UV-C/H2O2 and UV-C/chlorine in wastewater treatment.172
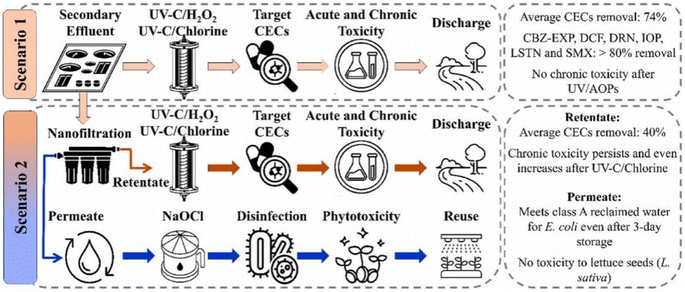 |
| Fig. 19 Schematic of the treatment scenarios in Silva et al.'s work (Copy right Elsevier 2024) ref. 172. | |
Miklos et al. examined the removal of 17 trace organic chemicals (TOrCs) from municipal wastewater using three UV-based advanced oxidation processes (AOPs): UV/H2O2, UV/PDS, and UV/chlorine. UV/chlorine showed the highest effectiveness, followed by UV/H2O2 and UV/PDS, with the latter two exhibiting greater selectivity (Fig. 20). While UV/chlorine was effective, it may generate more oxidation by-products. The study identified UV absorbance (UVA), total fluorescence (TF), and fluorescence peak as useful optical surrogates for predicting TOrC removal. The findings of this study highlight the strengths and limitations of these UV-AOPs in wastewater treatment.173
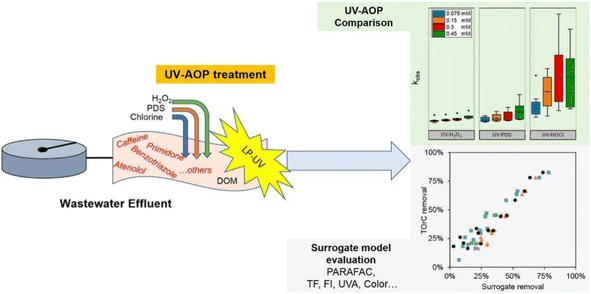 |
| Fig. 20 Wastewater effluent treatment using three UV-based advanced oxidation processes (AOPs) (Copyright Elsevier 2019) ref. 173. | |
4.2.1. UV disinfection and chemical oxidation methods.
UV-based advanced oxidation processes (UV-AOPs) are effective for treating municipal secondary effluents, with UV/H2O2, UV/chlorine, and UV/persulphate studied for converting dissolved effluent organic matter (dEfOM).174 UV/chlorine exhibits potential due to its significant reduction of acute toxicity.175 Compared to UV/H2O2, UV/chlorine is more energy-efficient, effective for disinfection and oxidation, and less impacted by water matrix components.176 In real water treatment, UV/chlorine and UV/persulfate AOPs demonstrate better efficacy and lower energy requirements than UV/H2O2, highlighting the need for further research on the molecular-level transformation of dEfOM in UV-AOPs to enhance municipal wastewater treatment.177,178
4.3. Biological treatment methods
Water treatment processes that use microorganisms to remove pollutants from wastewater are known as biological treatments.179 Biological treatment methods include sludge or biofilm processes.180 Sludge processes have a number of disadvantages such as high rates of sludge production but biofilm processes are more widely used.181 A variety of microbial species, such as Candidatus Accumulibacter Phosphatis (CAP), Spirogyra, Aspergillus luchuensis, and Candida, are used in biological wastewater treatment to remove contaminants.182 These biological treatment technologies are not only efficient and economic, but they also allow for the reclamation of water, which makes them a promising option for wastewater treatment in the future.183–185
Advanced wastewater treatment technologies based on biological processes encompass the sequencing batch reactor (SBR), moving bed biofilm reactor (MBBR), and membrane bioreactor (MBR). The SBR, functioning at the laboratory scale, proves effective in eliminating contaminants such as benzophenone-n (BPs) from commercial products, particularly when extending the hydraulic retention time (HRT) and during the reaction stage.186 MBBR employs biofilms for pollutant removal and has demonstrated successful performance in treating dairy wastewater, showcasing proficiency in organic matter and nutrient removal.187,188 MBR combines membrane filtration and biological treatment to produce high-quality effluent. It has been used to treat gray water and has demonstrated significant removal efficiency for pollutants such as total suspended solids (TSS) and COD.189
Dan A et al. assesses the removal of antibiotics from sewage treatment plant (STP) (Fig. 21) effluent using a hybrid constructed wetland (HCW) and a layered biological filter (LBF) at different hydraulic loading rates (HLRs). Quinolones achieved the highest removal efficiency in HCW (70–95%), followed by macrolides (58–77%) and tetracyclines (59–67%). LBF showed lower efficiencies, especially for macrolides (13–25%) and sulfonamides (<0%). Optimal HLRs were 1.0 m per day for quinolones and 2.0 m per day for tetracyclines and macrolides in HCW, and 6.4 m per day for LBF. Removal mechanisms included adsorption, microbial degradation, and photolysis for quinolones in HCW, while tetracyclines were mainly removed by adsorption. Plant uptake significantly aided macrolide removal in HCW. Overall, HCW outperformed LBF for most antibiotics, although LBF tolerated higher loads.190
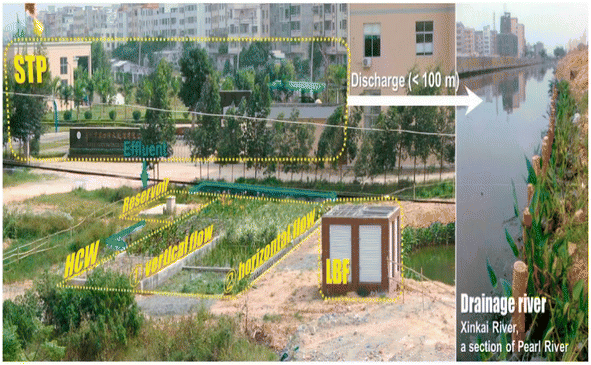 |
| Fig. 21 Layout of the full-scale system for purifying STP discharge (Copyright Elsevier 2021) ref. 190. | |
Antibiotic concentrations in sewage treatment plant effluent are ranked as follows: macrolides (108–2040 ng L−1) > sulfonamides (18.3–433 ng L−1) > quinolones (1.52–536 ng L−1) > tetracyclines (0.13–33.3 ng L−1). Average removal efficiencies are: quinolones in hybrid constructed wetlands (HCW) (70–95%) > macrolides in HCW (58–77%) > tetracyclines in both systems (59–67%) > quinolones in layered biological filters (LBF) (28–64%) > macrolides in LBF (13–25%) > sulfonamides in both systems (<0%). These differences in removal performance between HCW and LBF indicate distinct removal mechanisms in each system (Fig. 22).
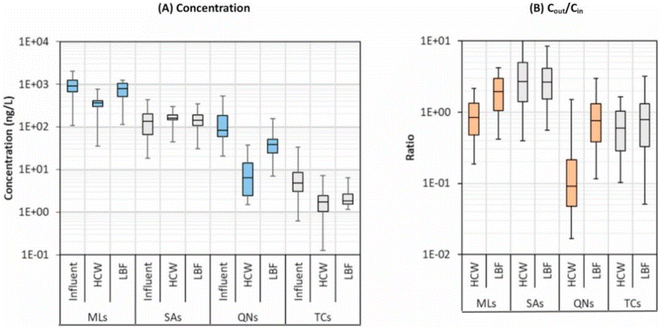 |
| Fig. 22 Total concentrations of four antibiotic categories (A) and their Cout/Cin (outlet concentration/inlet concentration) ratios (B) in the hybrid constructed wetland (HCW) and layered biological filter (LBF) (Copyright Elsevier 2021) ref. 190. | |
Piaskowski et al. investigated the impact of two cationic polyelectrolytes on activated sludge properties and treated wastewater quality in lab-scale sequencing batch reactors (SBRs) (Fig. 23). Dosing the polyelectrolytes improved the sludge volume index (SVI) by 42 to 80% compared to the control but overdosing increased SVI by 10–14% and produced difficult-to-thicken flocs. The polyelectrolytes, Praestol 855BS and Superfloc C-18530, did not significantly change the chemical characteristics of the treated wastewater but reduced turbidity by 54–64%. A simulation of a technological failure showed that Praestol formed stable flocs, while Superfloc led to a temporary decline in wastewater quality due to lower floc cohesion.191
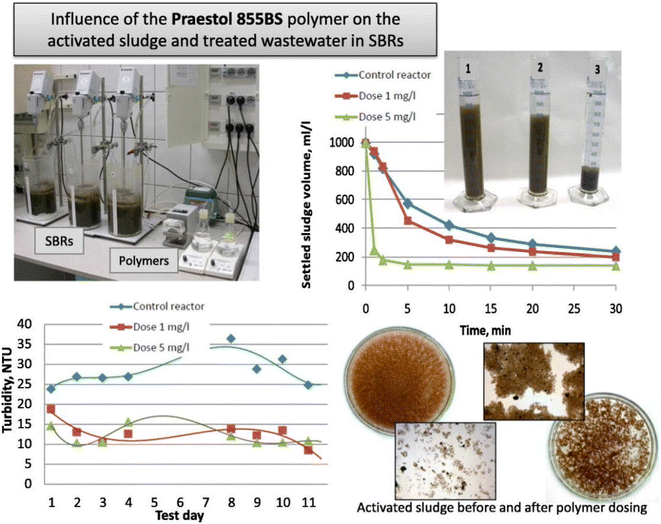 |
| Fig. 23 Influence of Praestol 855BS polymer on the activated sludge and treated wastewater in SBRs (Copyright Elsevier 2023) ref. 191. | |
Based upon the above discussions, the advantages and disadvantages of advanced technologies of wastewater treatment are presented in Fig. 24 and 25.
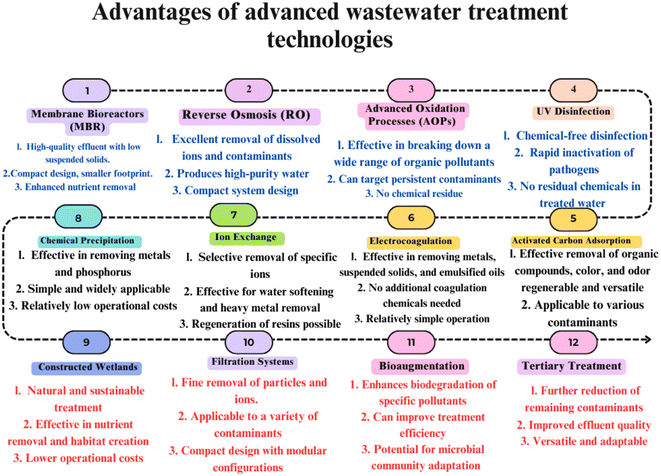 |
| Fig. 24 Advantages of advanced wastewater treatment technologies. | |
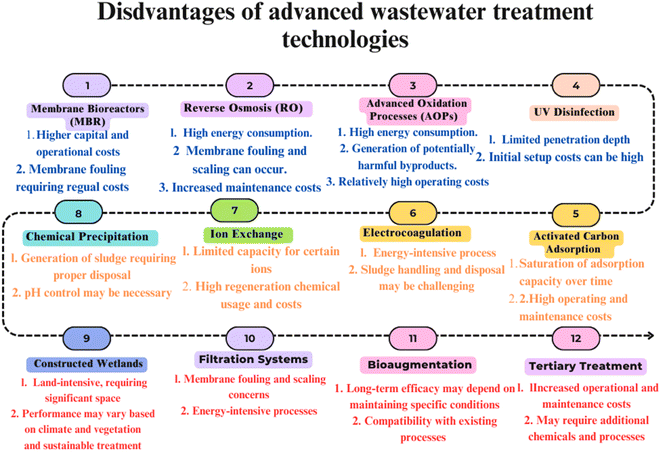 |
| Fig. 25 Disadvantages of advanced wastewater treatment technologies. | |
Wastewater treatment uses diverse methods for pollutant removal, ensuring water resource sustainability through physical, chemical, and biological processes. Rigorously constructed systems uphold stringent water quality standards, safeguarding human health and ecological balance.60 Through a balanced integration of technological advancements, environmental conservation measures, and economic viability considerations, sustainable management of wastewater treatment can be achieved.
Combining traditional methods such as activated sludge with cutting-edge technologies such as membrane bioreactors (MBRs) and electrochemical treatment offers a comprehensive approach to wastewater management.192 Hybrid systems show promise in effectively reducing contaminant levels, particularly chemical oxygen demand (COD), while enabling the recovery of valuable resources from wastewater.105 Nanotechnology emerges as a key focus for wastewater treatment, with nanomaterials demonstrating exceptional adsorption and degradation capabilities against various pollutants such as heavy metals and organic compounds.193 Research efforts should prioritize the development and evaluation of emerging nanomaterials in real-world environmental conditions to ensure performance and safety.194 These responsive composite materials can augment pollutant removal when integrated with traditional treatment methods, paving the way for them to evolve into smart pollution control tools. Furthermore, leveraging principles of circular economy in wastewater treatment can enhance resource recovery and waste minimization, including the extraction of nutrients, biogas, and other valuable compounds from wastewater for industrial reuse.195 By shifting towards a waste-to-resource paradigm, researchers can drive more sustainable practices in wastewater management.196 Collaboration among academia, industry, and policymakers is vital to promptly translating these novel approaches into viable solutions that address global water challenges.197
Summary of the technological innovations in wastewater treatment and their potential to integrate with conventional technologies are presented in Table 2. This table presents the AOP, membrane-based technologies in MBRs, wetlands, biological nutrient removal (BNR), constructed wetland operational conditions in relation to their range of application(s), advantages/challenges, and perceived environmental benefits/economic feasibility/potential integration capacity.
Table 2 Technological innovation in wastewater treatment
Technology |
Applications |
Advantages |
Challenges |
Environmental impact |
Economic feasibility |
Integration potential with conventional systems |
Ref. |
Advanced oxidation processes (AOPs) |
Removal of recalcitrant pollutants, disinfection |
Effective for various pollutants, complete mineralization |
High energy consumption, generation of by-products |
Can generate reactive oxygen species |
Moderate to high |
Can be integrated as a tertiary treatment step |
198 and 199 |
Membrane-based technologies |
Microfiltration, ultrafiltration, nanofiltration, reverse osmosis |
High removal efficiency, energy recovery |
Membrane fouling, high operational cost |
Lower sludge production, energy efficient |
Moderate to high |
Can be integrated at different treatment stages |
25, 200 and 201 |
Biological nutrient removal (BNR) |
Removal of nitrogen and phosphorus |
Cost-effective, energy efficient |
Sensitivity to operational conditions, sludge production |
Reduces nutrient pollution |
Low to moderate |
Can be integrated into activated sludge systems |
202 and 203 |
Constructed wetlands |
Treatment of municipal and industrial wastewater |
Low operational cost, ecological benefits |
Land requirement, slow treatment rate |
Nutrient removal, habitat creation |
Low to moderate |
Can be used as a tertiary treatment step |
204
|
Hybrid systems |
Combination of different technologies |
Enhanced treatment efficiency, resource recovery |
Complexity, higher investment cost |
Reduced environmental impact, energy recovery |
Moderate to high |
Can be customized for specific wastewater characteristics |
205
|
4.4. Monitoring technologies of wastewater treatment systems
Wastewater treatment process benefits from real-time monitoring, automation, and control technologies. A summary of these are presented in Fig. 26. These systems improve the sustainability and efficiency of water management. Real-time monitoring of wastewater is a social responsibility to improve water quality and addresses economic concerns. The transition to renewable energy helps to tackle climate change, yet the intermittent nature of these sources presents challenges for grid operators, requiring improved flexibility and integration.
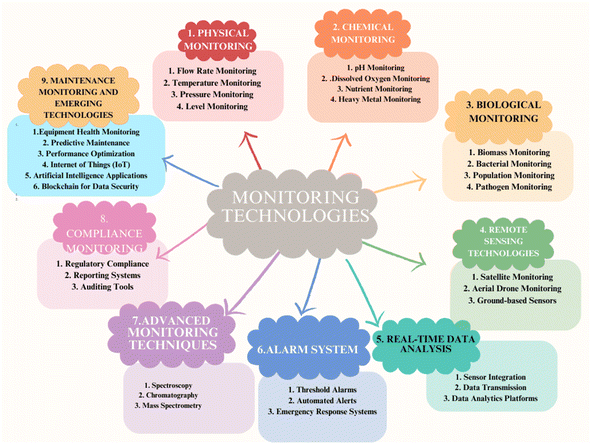 |
| Fig. 26 Monitoring technologies for wastewater treatment plants. | |
Automation and control systems lower operating costs and improve the state of the aquatic environment.206 Integrated control ensures sustainability.207 The utilization of Internet of Things (IoT), digital sensors, and actuators in the monitoring and control of water systems provides advantages such as remote access, accurate performance evaluation, and a decrease in the overall system cost.208Table 3 presents the key findings about monitoring technologies in wastewater treatment systems. Together, these technologies make the system automated, dependable, and integrated.214 In summary, integrating automation and control technologies with real-time monitoring is essential for improving the efficacy and efficiency of wastewater treatment systems.215–218
Table 3 Key discoveries about monitoring technologies in wastewater treatment systems
Key findings |
Advantages |
Challenges |
Ref. |
Real-time monitoring and automation |
Enhances system efficiency, lowers operational costs, and improves water quality. Facilitates remote access and accurate performance evaluation |
Requires initial investment in technology and infrastructure. Potential for technical issues or system failures that can disrupt monitoring |
209
|
Utilization of advanced sensors |
Provides continuous data on critical parameters (e.g., pH, turbidity, dissolved oxygen), enabling proactive decision-making and timely interventions |
Sensor calibration and maintenance can be challenging. Environmental factors may affect sensor accuracy and reliability |
210
|
Benefits of integrated monitoring systems |
Allows for continuous oversight of treatment processes, leading to proactive maintenance and compliance with regulations. Reduces risks and improves operational efficiency |
Integration with existing systems may be complex and require specialized knowledge. Data management and analysis can be resource-intensive |
211
|
Impact on sustainability and social responsibility |
Contributes to sustainability by promoting the use of renewable energy sources and addressing economic and environmental concerns. Enhances social responsibility in water management practices |
Transitioning to renewable energy sources may face infrastructure and regulatory hurdles. Public acceptance and understanding of new technologies can be challenging |
212
|
Cost-effectiveness and flexibility |
Lowers operational costs and improves facility flexibility, allowing better management of renewable energy sources |
Initial costs for automation technology can be high. Flexibility may require ongoing adjustments and updates to the system as conditions change |
213
|
4.5. Integration of renewable energy sources
Incorporating renewable energy sources into advanced wastewater treatment brings numerous benefits including energy conservation, reduced environmental impact, and resource recovery. Renewable sources such as solar, wind, and biomass can effectively support wastewater treatment facilities' energy demands and promote sustainability.67,68 For example, solar energy can be used for both electricity generation and heating, while wind energy supports tasks such as evaporation and concentration.
Environmental and economic benefits of integrating solar and biogas technologies into urban wastewater treatment facilities are substantial, as shown in Table 4. This trend aligns with a broader movement towards renewable energy, including solar, wind, geothermal, and hydroelectric power, to decrease fossil fuel dependence.207 Transitioning to renewable energy helps to reduce greenhouse gas emissions and address climate change, though the intermittent nature of these sources presents challenges for grid operators, requiring improved flexibility and integration.208
Table 4 Analysis of different wastewater treatment technologies for performance, environmental considerations, economic feasibility, trends and areas of improvement
Technology |
Performance indicators |
Environmental considerations |
Economic feasibility |
Trends |
Areas for improvement |
Ref. |
Conventional activated sludge |
BOD removal > 90%, TSS removal > 85% |
High energy consumption, potential for nutrient runoff |
Moderate initial costs, high operational costs |
Widely used, but facing scrutiny due to energy use |
Explore energy-efficient alternatives and nutrient recovery |
219
|
Membrane bioreactors (MBR) |
BOD removal > 95%, TSS removal > 99%, pathogen removal > 99% |
Reduced land footprint, lower sludge production, high energy use |
High capital costs, lower operational costs due to reduced sludge handling |
Increasing adoption in urban areas for space efficiency |
Reduce membrane fouling and improve cost-effectiveness |
219–222
|
Constructed wetlands |
BOD removal > 80%, nutrient removal varies |
Natural filtration, biodiversity enhancement |
Low capital and operational costs, land-intensive |
Growing interest in sustainable and natural treatment methods |
Optimize design for varying climates and pollutant loads |
223–227
|
Artificial intelligence optimization |
Real-time monitoring and predictive maintenance |
Improved efficiency, reduced chemical usage |
Potential for cost savings through optimized operations |
Rapid growth in AI applications for wastewater management |
Ensure data security and integration with existing systems |
33 and 228 |
Biogas production from sludge |
Methane yield > 70% of theoretical maximum |
Reduces greenhouse gas emissions, renewable energy source |
Initial investment in digesters, potential for energy revenue |
Rising interest in circular economy and energy recovery |
Enhance biogas yield and integrate with other processes |
229–231
|
Electrocoagulation |
BOD removal > 90%, TSS removal > 90% |
Generates sludge, energy-intensive |
Moderate capital costs, lower operational costs |
Emerging technology with potential for industrial applications |
Develop energy-efficient systems and better sludge management |
222 and 232–234 |
Advanced oxidation processes (AOP) |
Chemical oxygen demand (COD) reduction > 90% |
Produces hazardous by-products, requires careful management |
High operational costs, effective for specific contaminants |
Increasing use for treating recalcitrant pollutants |
Minimize by-product formation and improve cost-effectiveness |
29, 235 and 236 |
Nanotechnology |
Enhanced removal efficiencies for specific pollutants |
Potential environmental risks from nanoparticles |
High research and development costs, but promising long-term savings |
Innovative applications in pollutant removal |
Research environmental impacts and scalability |
237 and 238 |
Waste-to-energy technologies, such as those that process organic waste such as food waste, wastewater sludge, and animal manure, present significant potential for generating renewable energy and fuel.209,214 Integrating these renewable resources into wastewater treatment facilities not only enhances energy efficiency and environmental sustainability but also creates pathways for resource recovery, advancing the sector toward a low-carbon future.
Table 4 provides a detailed analysis of various wastewater treatment technologies, covering performance indicators, environmental benefits, economic feasibility, and areas for improvement. Conventional activated sludge systems, for example, achieve high biochemical oxygen demand (BOD) reduction (over 90%) and total suspended solid (TSS) removal (over 85%) but are energy-intensive, with moderate initial and high operational costs. Membrane bioreactors (MBRs) achieve even higher removal rates (BOD > 95%, TSS > 99%, pathogen removal > 99%) and require less land; however, they are associated with high capital costs and require improvements to reduce membrane fouling and enhance cost-effectiveness. Constructed wetlands provide BOD removal rates above 80%, with varying levels of nutrient removal, offering natural filtration and biodiversity benefits, though they are land intensive.
Other technologies such as artificial intelligence (AI) optimization, detailed in Table 4 allow for real-time monitoring and predictive maintenance, improving efficiency and reducing chemical use. Biogas production from sludge offers renewable energy by converting organic-rich sludge into methane, achieving methane yields of up to 70% of the theoretical maximum while reducing greenhouse gas emissions. Electrocoagulation, an emerging technology, removes over 90% of both BOD and TSS, although it is energy-intensive and produces sludge that requires effective management.
Advanced oxidation processes (AOP) achieve chemical oxygen demand (COD) reductions exceeding 90%, effectively treating recalcitrant pollutants; however, they produce hazardous by-products and have high operational costs. Nanotechnology, while promising for specific pollutant removal, is currently limited by high costs and potential environmental risks, keeping it largely experimental on a large scale.
A complementary view on the cost, efficiency, and scalability of these technologies is provided in Table 5. Conventional activated sludge systems, though effective for BOD, COD, and suspended solids removal, have moderate to high costs but are scalable for small to large applications. MBRs offer high efficiency for contaminant removal but are limited by membrane replacement costs. Constructed wetlands, while having low energy and maintenance costs, are suitable for various scales but require significant land, which limits their use in urban areas. AI-driven optimization provides cost savings by enhancing operational efficiency across treatment methods. Biogas production is ideal for facilities with high-organic waste and is scalable across treatment scales, while electrocoagulation is effective for certain pollutants but better suited for small- and medium-scale applications due to its energy demands. Both AOPs and nanotechnology show potential for removing hard-to-treat pollutants, though their scalability is restricted by high costs and complex implementation requirements.
Table 5 Analysis of different wastewater treatment technologies for cost, efficiency, and scalability
Technology |
Cost |
Efficiency |
Scalability |
Ref. |
Conventional activated sludge |
Capital and operating costs is moderate to high |
Highly efficient for BOD, COD, and suspended solid removal but may not be so effective for nutrient removal |
Widely applicable for small to large scales |
239–241
|
Membrane bioreactors (MBR) |
Membrane replacement – high capital and operating costs |
Highly efficient in removing BOD, COD, suspended solids as well as nutrients and has ability of high quality effluent. Production |
Costly but scalable for medium to large scales |
242–244
|
Constructed wetlands |
Lower energy and maintenance cost compared to the conventional system, low to moderate capital cost, high land requirement |
Having ability to remove organic matter, nutrients (N, P), suspended solids and trace metals. Long term operation has removal efficiency up to 91% |
Suitable for small to large scales, but requires large land |
245–248
|
Artificial intelligence optimization |
Lower life-cycle cost, moderate to high capital cost for implementation and maintenance |
Provides real time monitoring and optimization of existing treatment processes |
Application on different treatment technologies, highly scalable |
249–251
|
Biogas production from sludge |
May have moderate capital cost and utilizes biogas for energy savings |
Conversion of organic rich sludge to high biogas and nutrient rich digestate with high efficiency. It reduces greenhouse gases and energy consumption |
Ideal for organic waste, which is high, and therefore scalable to various scales |
252–255
|
Electrocoagulation |
High or moderate cost of operating due to energy consumed |
Good at removing suspended solids, heavy metals and a few organic pollutants. It can, however, be energy intensive |
Used as a pretreatment or polishing step, suitable for small to medium scales |
256 and 257 |
Advanced oxidation processes (AOP) |
Chemical consumption and energy that necessitates high capital and operating costs |
Utilized for the highly effective removal of recalcitrant organic compounds and pathogens |
Some of the least expensive catalytic synthesis routes are scalable, but due to high costs of the catalysts they are only used in a polishing step |
198 and 258–260 |
Nanotechnology |
A high capital and operating costs as well as potential environmental risks |
The potential with which it could remove a broad range of pollutants such as heavy metals and organic compounds is promising. For large scale application it is still in the experimental phase |
Due to high costs and experimental nature, has limited scalability |
237 and 261–263 |
5. Conclusion
The issue of water scarcity poses a critical challenge to humanity, stemming from factors such as population expansion and the impact of climate change. The treatment of wastewater emerges as a pivotal measure for safeguarding both human health and the broader environment. Nevertheless, endeavors to enhance the water quality and treatment encounter difficulties in keeping pace with the swift growth of our communities. Human activities including alterations to landscapes and the introduction of pollutants have intricate consequences on water resources, thereby exacerbating the predicament of water scarcity. Applications, advantages and limitations of some wastewater treatment technologies along with their environmental impact, economic feasibility as well as the integration potential were discussed in the paper. Each technology has its own merits and demerits, but an appropriate selection depends on the kind of wastewater composition. Applying bio-electrochemical systems (BESs) in constructed wetlands can contribute to sustainable, resource-efficient and environmentally friendly wastewater treatment. The two-value process is complementary, and the ideal pollutant removal/energy generation balance can be achieved by combining natural processes with technological solutions. The effective management of wastewater treatment necessitates a delicate balance between technological innovation, environmental preservation, and economic feasibility. Addressing these multifaceted challenges comprehensively is imperative to mitigate the adverse impacts of water scarcity and safeguard the well-being of both the environment and communities.
Data availability
As this is a review article, no new experimental data were generated or analysed in this study. All data supporting the findings are derived from the cited literature, which is publicly available in the referenced sources. Any figures or illustrations presented in the article were created by the authors and are available upon request.
Conflicts of interest
The authors declare no conflict of interest.
Acknowledgements
We are thankful to Rawalpindi Women University, Rawalpindi, Pakistan and Singapore Membrane Technology Centre, Nanyang Environment and Water Research Institute, Nanyang Technological University, 1 Cleantech Loop, 637141, Singapore for facilitating research.
References
- R. K. Mishra, Fresh water availability and its global challenge, Br. J. Multidiscip. Adv. stud., 2023, 4(3), 1–78 CrossRef.
-
C. Anastasi, Desalination and Water Security, Taylor & Francis, 2024 Search PubMed.
-
V. Singh, Water Resources, Textbook of Environment and Ecology, Springer, 2024, pp. 107–122 Search PubMed.
- A. Saravanan, P. S. Kumar, S. Jeevanantham, S. Karishma, B. Tajsabreen and P. Yaashikaa,
et al., Effective water/wastewater treatment methodologies for toxic pollutants removal: Processes and applications towards sustainable development, Chemosphere, 2021, 280, 130595 CrossRef CAS.
-
R. K. Sharma, B. Arora, S. Dutta and M. B. Gawande, Photo-oxidation technologies for advanced water treatment, Advanced Nano-Bio Technologies for Water and Soil Treatment, 2020, pp. 221–255 Search PubMed.
-
S. Sharma, R. Bangotra, B. Habib, M. Chib, A. Thakur, R. Mahajan, et al., Wastewater-Derived Biomass for Energy, Sewage and Biomass from Wastewater to Energy, 2024, pp. 195–224 Search PubMed.
-
V. S. Vishal, Seasonal Variations in the Performance of the Amberpet Wastewater Treatment Plant: Assessing the Impact of Temperature and Dissolved Oxygen, International Institute of Information Technology, Hyderabad, 2024 Search PubMed.
- F. Orata, Sewage and wastewater treatment plant performance: challenges and the environmental impact, Adv. Environ. Res., 2017, 137–160 CAS.
- Y. Gao, X. Shi, X. Jin, X. C. Wang and P. Jin, A critical review of wastewater quality variation and in-sewer processes during conveyance in sewer systems, Water Res., 2023, 228, 119398 CrossRef CAS.
-
E. K. Armah, M. Chetty, J. A. Adedeji, D. T. Kukwa, B. Mutsvene, K. P. Shabangu, et al., Emerging trends in wastewater treatment technologies: the current perspective, Promising Techniques for Wastewater Treatment and Water Quality Assessment, ed. I. A. Moujdin and J. K. Summers, 2021, pp. 71–95 Search PubMed.
- M. A. Hafiz, A. H. Hawari and A. Altaee, A hybrid forward osmosis/reverse osmosis process for the supply of fertilizing solution from treated wastewater, J. Water Proc. Eng., 2019, 32, 100975 CrossRef.
-
F. R. Spellman, Handbook of Water and Wastewater Treatment Plant Operations, CRC press, 2008 Search PubMed.
-
Effects of Water Pollution on Human Health and Disease Heterogeneity: A Review, ed. L. Lin, H. Yang and X. Xu, Frontiers in Environmental Science, 2022 Search PubMed.
- K. Javan, A. Altaee, S. BaniHashemi, M. Darestani, J. Zhou and G. Pignatta, A review of interconnected challenges in the water–energy–food nexus: Urban pollution perspective towards sustainable development, Sci. Total Environ., 2023, 169319 Search PubMed.
- A. K. Bourgeois, S. E. Tank, W. C. Floyd, M. B. Emelko and F. Amiri, Hydrology Predominates Over Harvest History and Landscape Variation to Control Water Quality and Disinfection Byproduct Formation Potentials in Forested Pacific Coast Watersheds, ACS ES&T Water, 2024, 4(4), 1335–1345 Search PubMed.
- N. E. Peters and M. Meybeck, Water quality degradation effects on freshwater availability: impacts of human activities, Water Int., 2000, 25(2), 185–193 CrossRef.
- A. Elmahdi, Addressing water scarcity in agricultural irrigation: By exploring alternative water resources for sustainable irrigated agriculture, Irrigat. Drain., 2024, 1–9 Search PubMed.
- R. Agrawal, S. Agrawal, A. Samadhiya, A. Kumar, S. Luthra and V. Jain, Adoption of green finance and green innovation for achieving circularity: An exploratory review and future directions, Geosci. Front., 2024, 15(4), 101669 CrossRef.
- D. Kundu, D. Dutta, A. Joseph, A. Jana, P. Samanta and J. N. Bhakta,
et al., Safeguarding drinking water: A brief insight on characteristics, treatments and risk assessment of contamination, Environ. Monit. Assess., 2024, 196(2), 180 CrossRef.
- A. Basu, D. Saha, R. Saha, T. Ghosh and B. Saha, A review on sources, toxicity and remediation technologies for removing arsenic from drinking water, Res. Chem. Intermed., 2014, 40, 447–485 CrossRef CAS.
- H. Wang, T. Wang, B. Zhang, F. Li, B. Toure and I. B. Omosa,
et al., Water and wastewater treatment in Africa–current practices and challenges, Clean: Soil, Air, Water, 2014, 42(8), 1029–1035 CrossRef CAS.
- S. Ethiraj, M. S. Samuel and S. Indumathi, A comprehensive review of the challenges and opportunities in microalgae-based wastewater treatment for eliminating organic, inorganic, and emerging pollutants, Biocatal. Agric. Biotechnol., 2024, 103316 CrossRef CAS.
- S. Ulitzur, T. Lahav and N. Ulitzur, A novel and sensitive test for rapid determination of water toxicity, Environ. Toxicol., 2002, 17(3), 291–296 CrossRef CAS.
- P. Ziegler, K. Sree and K.-J. Appenroth, Duckweeds for water remediation and toxicity testing, Toxicol. Environ. Chem., 2016, 98(10), 1127–1154 CrossRef CAS.
-
R. Molinari, C. Lavorato and P. Argurio, Hybrid membrane processes in advanced wastewater treatment, Current Trends and Future Developments on (Bio-) Membranes, Elsevier, 2024, pp. 811–844 Search PubMed.
-
L. N. Nthunya, S. Mbakop and S. D. Mhlanga, Emerging nanoenhanced membrane-based hybrid processes for complex industrial wastewater treatment, Membrane-based Hybrid Processes for Wastewater Treatment, Elsevier, 2021, pp. 633–656 Search PubMed.
- J. B. Adeoye, Y. H. Tan, S. Y. Lau, Y. Y. Tan, T. Chiong and N. M. Mubarak,
et al., Advanced oxidation and biological integrated processes for pharmaceutical wastewater treatment: a review, J. Environ. Manage., 2024, 353, 120170 CrossRef CAS PubMed.
- F. Long, D. Ghani, R. Huang and C. Zhao, Versatile electrode materials applied in the electrochemical advanced oxidation processes for wastewater treatment: A systematic review, Sep. Purif. Technol., 2024, 128725 Search PubMed.
- A. Babuponnusami, S. Sinha, H. Ashokan, M. V. Paul, S. P. Hariharan and J. Arun,
et al., Advanced oxidation process (AOP) combined biological process for wastewater treatment: A review on advancements, feasibility and practicability of combined techniques, Environ. Res., 2023, 116944 CrossRef CAS.
- B. J. Singh, A. Chakraborty and R. Sehgal, A systematic review of industrial wastewater management: Evaluating challenges and enablers, J. Environ. Manage., 2023, 348, 119230 CrossRef PubMed.
- I. Ribarova, V. Vasilaki and E. Katsou, Review of linear and circular approaches to on-site domestic wastewater treatment: Analysis of research achievements, trends and distance to target, J. Environ. Manage., 2024, 367, 121951 CrossRef.
- A. Kumar, E. Singh, R. Mishra, S. L. Lo and S. Kumar, Global trends in municipal solid waste treatment technologies through the lens of sustainable energy development opportunity, Energy, 2023, 275, 127471 CrossRef.
- Y. Wang, Y. Cheng, H. Liu, Q. Guo, C. Dai and M. Zhao,
et al., A review on applications of artificial intelligence in wastewater treatment, Sustainability, 2023, 15(18), 13557 CrossRef CAS.
- G. Gedda, K. Balakrishnan, R. U. Devi, K. J. Shah, V. Gandhi and V. Gandh,
et al., Introduction to conventional wastewater treatment technologies: limitations and recent advances, Mater. Res. Found., 2021, 91, 1–36 CAS.
- A. I. Shah, M. U. D. Dar, R. A. Bhat, J. Singh, K. Singh and S. A. Bhat, Prospectives and challenges of wastewater treatment technologies to combat contaminants of emerging concerns, Ecol. Eng., 2020, 152, 105882 CrossRef.
-
D. J. Rodriguez, H. A. Serrano, A. Delgado, D. Nolasco and G. Saltiel, From Waste to Resource: Shifting Paradigms for Smarter Wastewater Interventions in Latin America and the Caribbean, 2020 Search PubMed.
-
S. Ganesan, Promising Technologies in Wastewater Treatment: The Current Standpoint, Sustainable Water Treatment and Ecosystem Protection Strategies, Apple Academic Press, 2024, pp. 313–325 Search PubMed.
-
V. Rajadurai, V. M. Santhi and S. Muthulingam, Removal of micropollutants from industrial wastewater using sequencing batch biofilm reactor, Emerging Innovative Trends in the Application of Biological Processes for Industrial Wastewater Treatment, Elsevier, 2024, pp. 209–235 Search PubMed.
- H. M. Diallo, H. Ayyoub, F. Elazhar, M. Tahaikt, A. Elmidaoui and M. Taky, Evaluation of the potential ultrafiltration to improve the quality of secondary effluents in tertiary treatment and reuse, Phys. Chem. Earth, 2024, 103672 CrossRef.
-
J. P. Chaudhary and P. Jhajharia, Recent Advances in Wastewater Treatment, Integrated Waste Management: A Sustainable Approach from Waste to Wealth, 2024, pp. 289–302 Search PubMed.
- S. Turkar, D. Bharti and G. Gaikwad, Various methods involved in waste water treatment to control water pollution, J. Chem. Pharmaceut. Res., 2011, 3(2), 58–65 CAS.
-
N. A. Wani, N. A. Malik, Y. R. Tantary, I. Jan, T. Ahmad and M. S. Wani. Emerging Techniques for Treatment of Wastewater, Aquatic Contamination: Tolerance and Bioremediation, 2024, pp. 1–23 Search PubMed.
- H. M. Hegab, A. ElMekawy, B. van den Akker, M. Ginic-Markovic, C. Saint and G. Newcombe,
et al., Innovative graphene microbial platforms for domestic wastewater treatment, Rev. Environ. Sci. Bio/Technol., 2018, 17, 147–158 CrossRef CAS.
- A. M. Abdelrahman, S. Kosar, H. Gulhan, B. Cicekalan, G. Ucas and E. Atli,
et al., Impact of primary treatment methods on sludge characteristics and digestibility, and wastewater treatment plant-wide economics, Water Res., 2023, 235, 119920 CrossRef CAS PubMed.
- R. Sangamnere, T. Misra, H. Bherwani, A. Kapley and R. Kumar, A critical review of conventional and emerging wastewater treatment technologies, Sustain. Water Resour. Manag., 2023, 9(2), 58 CrossRef.
-
A. Inobeme, A. I. Ajai, C. O. Adetunji, J. Inobeme, M. Maliki, E. Y. Shaba, et al., Wastewater Treatment Technologies. Industrial Wastewater Reuse: Applications, Prospects and Challenges, Springer, 2023, pp. 201–213 Search PubMed.
-
R. Sharma, N. Verma, Y. Lugani, S. Kumar and M. Asadnia, Conventional and advanced techniques of wastewater monitoring and treatment, Green Sustainable Process for Chemical and Environmental Engineering and Science, Elsevier, 2021, pp. 1–48 Search PubMed.
-
R. Smith, Cost of Conventional and Advanced Treatment of Wastewaters, US Department of the Interior, Federal Water Pollution Control, 1968 Search PubMed.
- A. T. Nguyen and L. Le Tran, A Review About the Occurrence and Effectiveness of Conventional and Advanced Treatment Technologies of Persistent Organic Pollutants in Surface Water, Rev. Environ. Contam. Toxicol., 2024, 262(1), 11 CrossRef CAS.
- N. T. Nguyen, T. S. Vo, P. L. Tran-Nguyen, M. N. Nguyen, R. Matsuhashi and K. Kim,
et al., A comprehensive review of aeration and wastewater treatment, Aquaculture, 2024, 741113 CrossRef CAS.
-
D. M. El-Sherif, A. E. D. Mahmoud and A. N. Saber, The Application of the Algal Approach in the Technological, Socioeconomic, and Wastewater Treatment Domains, Algae as a Natural Solution for Challenges in Water-Food-Energy Nexus: toward Carbon Neutrality, Springer, 2024, pp. 261–295 Search PubMed.
-
M. Sharma, A. Yadav, M. K. Mandal, S. Pandey, S. Pal, H. Chaudhuri, et al., Wastewater treatment and sludge management strategies for environmental sustainability, Circular Economy and Sustainability, Elsevier, 2022, pp. 97–112 Search PubMed.
- S. Manikandan, R. Subbaiya, M. Saravanan, M. Ponraj, M. Selvam and A. Pugazhendhi, A critical review of advanced nanotechnology and hybrid membrane based water recycling, reuse, and wastewater treatment processes, Chemosphere, 2022, 289, 132867 CrossRef CAS.
- P. R. Rout, T. C. Zhang, P. Bhunia and R. Y. Surampalli, Treatment technologies for emerging contaminants in wastewater treatment plants: A review, Sci. Total Environ., 2021, 753, 141990 CrossRef CAS PubMed.
-
W. M. Alley and R. Alley, The Water Recycling Revolution: Tapping into the Future, Rowman & Littlefield, 2022 Search PubMed.
- T. Mahmood, S. Momin, R. Ali, A. Naeem and A. Khan, Technologies for removal of emerging contaminants from wastewater, Wastewater Treat., 2022, 159, 1–20 Search PubMed.
-
Waste Water Treatment Technology by the Anaerobic with IC Reactor, ed. D. U. Nurislamiyati and L. Milana, Seminar Teknologi Majalengka (STIMA), 2022 Search PubMed.
-
A. Devi, A. Singh, M. Mahajan, S. Sahab, V. Srivastava, P. Singh, et al., Trends in Waste Water Treatment using Phycoremediation for Biofuel Production, Bioremediation of Toxic Metal(loid)s, CRC Press, 2022, pp. 153–167 Search PubMed.
- S. Poornima, S. Manikandan, V. Karthik, R. Balachandar, R. Subbaiya and M. Saravanan,
et al., Emerging nanotechnology based advanced techniques for wastewater treatment, Chemosphere, 2022, 303, 135050 CrossRef CAS.
-
B. Verma and C. Balomajumder, Technology of Carbon Nanotubes for Waste Water Treatment, Nanotechnology Applications in Agricultural and Bioprocess Engineering: Farm to Table, 2022, p. 215 Search PubMed.
-
F. K. Donkor, Adaptive Water, Sanitation, and Hygiene Management: Resilient Governance Systems, Clean Water and Sanitation, Springer, 2022, pp. 1–12 Search PubMed.
-
V. G. Lade, Introduction of water remediation processes, Handbook of Nanomaterials for Wastewater Treatment, Elsevier, 2021, pp. 741–777 Search PubMed.
-
O. J. Ajala, J. O. Tijani, M. T. Bankole and A. S. Abdulkareem, Wastewater treatment technologies, Inorganic–Organic Composites for Water and Wastewater Treatment, vol. 1, 2022, pp. 1–28 Search PubMed.
- G. Palani, A. Arputhalatha, K. Kannan, S. K. Lakkaboyana, M. M. Hanafiah and V. Kumar,
et al., Current trends in the application of nanomaterials for the removal of pollutants from industrial wastewater treatment—a review, Molecules, 2021, 26(9), 2799 CrossRef CAS PubMed.
- S. Cheriyamundath and S. L. Vavilala, Nanotechnology-based wastewater treatment, Water Environ. J., 2021, 35(1), 123–132 CrossRef CAS.
- R. Trivedi, T. K. Upadhyay, F. Khan, P. Pandey, R. S. Kaushal and M. Sonkar,
et al., Innovative strategies to manage polluted aquatic ecosystem and agri-food waste for circular economy, Environ. Nanotechnol. Monit. Manag., 2024, 100928 CAS.
-
S. Murad and J. G. Powles, Fluids in Contact with Semi-permeable Membranes, Computational Methods in Surface and Colloid Science, CRC Press, 2019, pp. 775–797 Search PubMed.
- A. Iulianelli and E. Drioli, Membrane engineering: Latest advancements in gas separation and pre-treatment processes, petrochemical industry and refinery, and future perspectives in emerging applications, Fuel Process. Technol., 2020, 206, 106464 CrossRef CAS.
-
A. Mounika, L. Kavitha, S. Akalya and D. Chidanand, Recent Advances in Membrane Processing, Non-Thermal Technologies for the Food Industry, 2024, pp. 247–266 Search PubMed.
- R. Kumar, E. Awino, D. W. Njeri, A. Basu, S. Chattaraj and J. Nayak,
et al., Advancing pharmaceutical wastewater treatment: A comprehensive review on application of catalytic membrane reactor-based hybrid approaches, J. Water Proc. Eng., 2024, 58, 104838 CrossRef.
- M. J. Khan, A. Wibowo, Z. Karim, P. Posoknistakul, B. M. Matsagar and K. C.-W. Wu,
et al., Wastewater Treatment Using Membrane Bioreactor Technologies: Removal of Phenolic Contaminants from Oil and Coal Refineries and Pharmaceutical Industries, Polymers, 2024, 16(3), 443 CrossRef CAS.
- W. Gong, L. Bai and H. Liang, Membrane-based technologies for removing emerging contaminants in urban water systems: Limitations, successes, and future improvements, Desalination, 2024, 117974 CrossRef CAS.
-
N. Ashok, M. Sruthi, T. R. Abraham and S. Thomas, Polymer membrane for desalination and distillation, Polymer Membranes: Increasing Energy Efficiency, 2024, p. 261 Search PubMed.
- Y. Xue, M. Kamali, S. Al-Salem, B. Rossi, L. Appels and R. Dewil, Naturally derived materials to enhance the membrane properties in (waste) water treatment applications-Mechanisms, scale-up challenges and economic considerations, J. Water Proc. Eng., 2024, 57, 104647 CrossRef.
- M. J. Talukder, A. S. Alshami, A. Tayyebi, N. Ismail and X. Yu, Membrane science meets machine learning: future and potential use in assisting membrane material design and fabrication, Sep. Purif. Rev., 2024, 53(2), 216–229 CrossRef.
-
C. G. Matter, Membrane filtration (micro and ultrafiltration) in water purification, Handbook of Water and Used Water Purification, Springer, 2024, pp. 121–137 Search PubMed.
- P. Sanchis-Perucho, D. Aguado, J. Ferrer, A. Seco and A. Á. Robles, A comprehensive review of the direct membrane filtration of municipal wastewater, Environ. Technol. Innovat., 2024, 103732 CrossRef CAS.
- Q. Ma, Q. Lei, F. Liu, Z. Song, B. Khusid and W. Zhang, Evaluation of commercial nanofiltration and reverse osmosis membrane filtration to remove per-and polyfluoroalkyl substances (PFAS): Effects of transmembrane pressures and water matrices, Water Environ. Res., 2024, 96(2), e10983 CrossRef CAS.
- S. Cairone, V. Naddeo, V. Belgiorno, M. J. Taherzadeh and A. Mahboubi, Evaluating the impact of membrane properties and feed pH on concentration and fractionation of volatile fatty acid using nanofiltration, J. Water Proc. Eng., 2024, 65, 105793 CrossRef.
-
Nordic membrane[cited 2024 5-08-2024]. Available from: https://nordicmembrane.com/membrane_technology#.
- D. Suresh, P. Goh, H. Kang, M. Ahmad and A. Ismail, Waste Reutilization in Pollution Remediation: Paving New Paths for Wastewater Treatment, J. Environ. Chem. Eng., 2024, 113570 CrossRef CAS.
- F. A. Akash, S. M. Shovon, W. Rahman, M. A. Rahman, P. Chakraborty and T. A. E. Prasetya,
et al., Advancements in ceramic membrane technology for water and wastewater treatment: A comprehensive exploration of current utilizations and prospective horizons, Desalination Water Treat., 2024, 100569 CrossRef.
- A. Wang, C. Chen, C. Liu, J. Ma, T. Lin and M. Ding,
et al., Critical review on advances and perspectives of ultrasound assisted membrane technologies for water purification, Chem. Eng. J., 2024, 148873 CrossRef CAS.
- A. Qadri and M. Alam, Drinking water treatment using advanced technologies, Int. J. Biol. Chem., 2024, 25(14), 154–163 Search PubMed.
- D. L. Zhao, W. Zhou, L. Shen, B. Li, H. Sun and Z. Qianqian,
et al., New directions on membranes for removal and degradation of emerging pollutants in aqueous systems, Water Res., 2024, 121111 CrossRef CAS.
- D. Baskaran, D. Dhamodharan, U. S. Behera and H.-S. Byun, A comprehensive review and perspective research in technology integration for the treatment of gaseous volatile organic compounds, Environ. Res., 2024, 118472 CrossRef CAS.
- A. Naeem, B. Saeed, H. AlMohamadi, M. Lee, M. A. Gilani and R. Nawaz,
et al., Sustainable and green membranes for chemical separations: A review, Sep. Purif. Technol., 2024, 126271 CrossRef CAS.
-
O. Nwanna-Nzewunwa and S. Keshavamurthy, Evolving Therapies and Technologies in Extracorporeal Membrane Oxygenation, 2024 Search PubMed.
- A. I. Osman, Z. Chen, A. M. Elgarahy, M. Farghali, I. M. Mohamed and A. Priya,
et al., Membrane technology for energy saving: principles, techniques, applications, challenges, and prospects, Adv. Energy Sustainability Res., 2024, 5(5), 2400011 CrossRef.
- Y. Ezaier, A. Hader, A. Latif, M. E. Khan, W. Ali and S. K. Ali,
et al., Solving the fouling mechanisms in composite membranes for water purification: An advance approach, Environ. Res., 2024, 250, 118487 CrossRef CAS PubMed.
- S. Shalaby, M. E. Zayed, F. A. Hammad, A. S. Menesy and A. R. Abd Elbar, Recent advances in membrane distillation hybrids for energy-efficient process configurations:
Technology categorization, operational parameters identification, and energy recovery strategies, Process Saf. Environ. Prot., 2024, 817–838 CrossRef CAS.
-
A. C. Habert, C. P. Borges, F. de Araujo Kronemberger and H. C. Ferraz, R. Nobrega, 5 Membrane Separation, Purification of Biotechnological Products: A Focus on Industrial Applications, 2024, vol. 82 Search PubMed.
- B. Díez and R. Rosal, A critical review of membrane modification techniques for fouling and biofouling control in pressure-driven membrane processes, Nanotechnol. Environ. Eng., 2020, 5, 1–21 CrossRef.
-
E. K. Tetteh, S. Rathilal, M. Chetty, E. K. Armah and D. Asante-Sackey, Treatment of water and wastewater for reuse and energy generation-emerging technologies, Water and Wastewater Treatment, 2019, pp. 53–80 Search PubMed.
- S. Munirasu, M. A. Haija and F. Banat, Use of membrane technology for oil field and refinery produced water treatment—A review, Process Saf. Environ. Prot., 2016, 100, 183–202 CrossRef CAS.
- S. J. Kulkarni, Biological treatment of petroleum wastewater: A review on research and studies, Int. J. Pet. Eng., 2016, 2(2), 17–21 Search PubMed.
- Z. Yang, Current Status and Development Trend of Advanced Treatment Technologies for Waste Water of Paper Industry in China, China Pulp Pap., 2011, 30(10), 56 Search PubMed.
-
K. P. Mehta, Application of nano technology in waste water treatment, Climate Change and Water Security: Select Proceedings of VCDRR 2021, Springer, 2021, pp. 423–432 Search PubMed.
- M. O. Awaleh and Y. D. Soubaneh, Waste water treatment in chemical industries: the concept and current technologies, Hydrol.:Curr. Res., 2014, 5(1), 1–12 Search PubMed.
-
S. Khaturia, H. L. Singh, M. Chahar and A. Bishnoi, Emerging Innovative Technologies for Wastewater Treatment. Emerging Technologies in Wastewater Treatment, CRC Press, 2023, pp. 155–169 Search PubMed.
-
R. Yazdani, S. Harirchi, M. Lak, M. Mirshafiei, S. A. Nojoumi, M. Ramezani, et al., 5 Recent Advances in Conventional and Modern Wastewater Treatment Approaches, Microbial Nexus for Sustainable Wastewater Treatment: Resources, Efficiency, and Reuse, 2024 Search PubMed.
- G. Hooshyari, A. Bose and W. A. Jackson, Integration of Full-Size Graywater Membrane-Aerated Biological Reactor with Reverse Osmosis System for Space-Based Wastewater Treatment, Membranes, 2024, 14(6), 127 CrossRef CAS.
- R. W. Holloway, A. Achilli and T. Y. Cath, The osmotic membrane bioreactor: a critical review, Environ. Sci.:Water Res. Technol., 2015, 1(5), 581–605 RSC.
- S. M. Korotta-Gamage and A. Sathasivan, A review: Potential and challenges of biologically activated carbon to remove natural organic matter in drinking water purification process, Chemosphere, 2017, 167, 120–138 CrossRef CAS.
- J. Kim, B. Wu, S. Jeong, S. Jeong and M. Kim, Recent advances of membrane-based hybrid membrane bioreactors for wastewater reclamation, Front. Membr. Sci. Technol., 2024, 3, 1361433 CrossRef.
- A. P. Periyasamy, Recent advances in the remediation of textile-dye-containing wastewater: prioritizing human health and sustainable wastewater treatment, Sustainability, 2024, 16(2), 495 CrossRef CAS.
- M. A. Alhussaini, B. M. Souza-Chaves, V. Felix and A. Achilli, Comparative analysis of reverse osmosis and nanofiltration for the removal of dissolved contaminants in water reuse applications, Desalination, 2024, 117822 CrossRef CAS.
- Y. Men, X. Wang, S. Cheng, L. Zhu and Z. Li, Pre-coagulation with novel titanium coagulants for mitigating membrane fouling in direct membrane filtration of municipal wastewater, Chem. Eng. J., 2024, 153156 CrossRef CAS.
- D. Zamel and A. U. Khan, New trends in nanofibers functionalization and recent applications in wastewater treatment, Polym. Adv. Technol., 2021, 32(12), 4587–4597 CrossRef CAS.
- J. S. Paneysar, S. Sawant, M. H. Ip, S. K. Bhullar, S. Barton and P. Ambre,
et al., Nanofibers for textile waste water management, Water Pract. Technol., 2019, 14(2), 297–310 CrossRef.
- Z. Abousalman-Rezvani, H. Roghani-Mamaqani, H. Riazi and O. Abousalman-Rezvani, Water treatment using stimuli-responsive polymers, Polym. Chem., 2022, 13(42), 5940–5964 RSC.
- V. Gadore and M. Ahmaruzzaman, Smart materials for remediation of aqueous environmental contaminants, J. Environ. Chem. Eng., 2021, 9(6), 106486 CrossRef CAS.
- F. Asghar and A. Mushtaq, The future of nanomaterial in wastewater treatment: a review, Int. J. Biol. Chem., 2023, 23, 150–157 Search PubMed.
- A. Aldalbahi, M. E. El-Naggar, M. H. El-Newehy, M. Rahaman, M. R. Hatshan and T. A. Khattab, Effects of technical textiles and synthetic nanofibers on environmental pollution, Polymers, 2021, 13(1), 155 CrossRef CAS.
- P. S. Kumar, G. J. Joshiba, C. C. Femina, P. Varshini, S. Priyadharshini and M. Karthick,
et al., A critical review on recent developments in the low-cost adsorption of dyes from wastewater, Desalin. Water Treat., 2019, 172(19), 395–416 CrossRef.
- J. S. Paneysar, S. Jain, N. Ahmed, S. Barton, P. Ambre and E. Coutinho, Novel smart composite materials for industrial wastewater treatment and reuse, SN Appl. Sci., 2020, 2, 1–12 Search PubMed.
- V. Kumar and P. Verma, Pulp-paper industry sludge waste biorefinery for sustainable energy and value-added products development: A systematic valorization towards waste management, J. Environ. Manage., 2024, 352, 120052 CrossRef CAS PubMed.
-
A. R. Rao and G. A. Ravishankar, Algae-Based Bioremediation of Pulp and Paper Mill Wastewater: An Overview, Algae Mediated Bioremediation: Industrial Prospectives, 2024, vol. 2, pp. 377–397 Search PubMed.
-
P. Kumari, S. Malik, J. Bora, S. Mondal, R. Rani and A. Kapoor, Applied aspects of reverse osmosis technology for industrial wastewater treatment, Development in Wastewater Treatment Research and Processes, 2024, pp. 341–366 Search PubMed.
- R. V. P. Patel and H. Raval, Comparative assessment of treatment technologies for minimizing reverse osmosis concentrate volume for industrial applications: A review, Water Sci. Technol., 2024, 90(1), 314–343 CrossRef CAS PubMed.
- F. Ocklind, K. Liback, L. Lundqvist, W. Harge and V. Govindarajan, Optimisation of Water-Use in Pulp and Paper Mills:: A Streamlined Review of Scientific Journal Publications, Stud. Ecol. Bioethicae., 2024, 22(3), 1–15 Search PubMed.
-
N. Kataria, S. Yadav, P. K. Rose and V. K. Garg, Pharmaceutical Contamination in Water and Wastewater: Remediation Technology and Future Challenges, Pharmaceuticals in Aquatic Environments, CRC Press, 2024, pp. 1–20 Search PubMed.
- J. Iqbal, N. S. Shah, J. A. Khan, M. Naushad, G. Boczkaj and F. Jamil,
et al., Pharmaceuticals wastewater treatment via different advanced oxidation processes: Reaction mechanism, operational factors, toxicities, and cost evaluation–A review, Sep. Purif. Technol., 2024, 127458 CrossRef CAS.
- T. Melin, B. Jefferson, D. Bixio, C. Thoeye, W. De Wilde and J. De Koning,
et al., Membrane bioreactor technology for wastewater treatment and reuse, Desalination, 2006, 187(1–3), 271–282 CrossRef CAS.
- X. Hao, J. Li, M. Van Loosdrecht and T. Li, A sustainability-based evaluation of membrane bioreactors over conventional activated sludge processes, J. Environ. Chem. Eng., 2018, 6(2), 2597–2605 CrossRef CAS.
-
M. Al-Obaidi, C. Kara-Zaitri and I. M. Mujtaba, Wastewater Treatment by Reverse Osmosis Process, CRC Press, 2020 Search PubMed.
- I. A. Tałałaj, P. Biedka and I. Bartkowska, Treatment of landfill leachates with biological pretreatments and reverse osmosis, Environ. Chem. Lett., 2019, 17, 1177–1193 CrossRef.
- Z. Yang, Y. Zhou, Z. Feng, X. Rui, T. Zhang and Z. Zhang, A review on reverse osmosis and nanofiltration membranes for water purification, Polymers, 2019, 11(8), 1252 CrossRef CAS PubMed.
-
A. Tiraferri, Forward osmosis for water treatment and desalination, Current Trends and Future Developments on (Bio-) Membranes, Elsevier, 2020, pp. 53–81 Search PubMed.
- J. Wang and X. Liu, Forward osmosis technology for water treatment: Recent advances and future perspectives, J. Clean. Prod., 2021, 280, 124354 CrossRef CAS.
- A. U. Khan, A. N. Khan, A. Waris, M. Ilyas and D. Zamel, Phytoremediation of pollutants from wastewater: A concise review, Open Life Sci., 2022, 17(1), 488–496 CrossRef CAS.
- Z. Mohebi and M. Nazari, Phytoremediation of wastewater using aquatic plants, A review, J. Appl. Res. Water Wastewater, 2021, 8(1), 50–58 CAS.
- C. A. Syamlal, A. George and D. Sayantan, Phytoremediation: An Eco-Friendly Solution for Environmental Contamination, Int. Adv. Res. Sci. Commun. Technol., 2024, 4(1), 553–577 Search PubMed.
- K. K. Awasthi, G. Awasthi, Y. Tiwari, T. Singh, A. Awasthi and S. Srivastava,
et al., Quality Improvement of Reverse Osmosis Waste Waterthrough Plant-Based Techniques: A Mini-Review, Int. J. Plant Sci., 2020, 6(02), 156–161 Search PubMed.
- B. M. Ibraheem, S. A. Aani, A. A. Alsarayreh, Q. F. Alsalhy and I. K. Salih, Forward osmosis membrane: review of fabrication, modification, challenges and potential, Membranes, 2023, 13(4), 379 CrossRef CAS PubMed.
-
M. R. Lu, A Review of Forward Osmosis Application on Fertigation and Desalination, University of California, Irvine, 2022 Search PubMed.
- S. Mohammed, J. Aburabie and R. Hashaikeh, Facile morphological tuning of thin film composite membranes for enhanced desalination performance, npj Clean Water, 2023, 6(1), 55 CrossRef CAS.
- D. Qin, Z. Liu, D. Delai Sun, X. Song and H. Bai, A new nanocomposite forward osmosis membrane custom-designed for treating shale gas wastewater, Sci. Rep., 2015, 5(1), 14530 CrossRef CAS.
-
Short review on solvent resistance nanofiltration membranes for the treatment of waste water, AIP Conference Proceedings, ed. O. Agboola, O. Fayomi, R. Sadiku, A. Popoola, A. Adegbola, L. Moropeng, et al., AIP Publishing, 2022 Search PubMed.
-
N. Patel, A. Dhasmana, S. Kumari, R. Sharma, S. Nayanam and S. Malik, Nanofiltration Applications for Potable Water, Treatment, and Reuse, Advanced and Innovative Approaches of Environmental Biotechnology in Industrial Wastewater Treatment, Springer, 2023, pp. 135–146 Search PubMed.
-
A. Kanwal, A. A. Yaqoob, A. Siddique, M. N. M. Ibrahim and A. Ahmad, Polyethersulfone (PES) nanofiltration membrane for treatment of toxic metal contaminated water, Emerging Techniques for Treatment of Toxic Metals from Wastewater, Elsevier, 2023, pp. 319–341 Search PubMed.
-
Investigation of Water Treatment Produced by Nanofiltration, 2023 IEEE 3rd International Maghreb Meeting of the Conference on Sciences and Techniques of Automatic Control and Computer Engineering (MI-STA), ed. A. J. Boukar and R. R. Alfarah, IEEE, 2023 Search PubMed.
- P. Laurell, K. Sivonen, M. Hesampour, T. Tuutijärvi and R. Vahala, Application and environmental impact of loose nanofiltration in surface water treatment, J. Water Proc. Eng., 2024, 60, 105174 CrossRef.
- R. Ghanbari, E. N. Zare, A. C. Paiva-Santos and N. Rabiee, Ti3C2Tx MXene@ MOF decorated polyvinylidene fluoride membrane for the remediation of heavy metals ions and desalination, Chemosphere, 2023, 311, 137191 CrossRef CAS.
- N. Li, T.-J. Lou, W. Wang, M. Li, L.-C. Jing and Z.-X. Yang,
et al., MXene-PANI/PES composite ultrafiltration membranes with conductive properties for anti-fouling and dye removal, J. Membr. Sci., 2023, 668, 121271 CrossRef CAS.
- C. Zhao, Y. Zhang, Y. Jia, B. Li, W. Tang and C. Shang,
et al., Polyamide membranes with nanoscale ordered structures for fast permeation and highly selective ion-ion separation, Nat. Commun., 2023, 14(1), 1112 CrossRef CAS.
- Q. Zeng, X. Zhou, L. Shen, D. L. Zhao, N. Kong and Y. Li,
et al., Exceptional self-cleaning MXene-based membrane for highly efficient oil/water separation, J. Membr. Sci., 2024, 700, 122691 CrossRef CAS.
- M. Cuartucci, Ultrafiltration, a cost-effective solution for treating surface water to potable standard, Water Pract. Technol., 2020, 15(2), 426–436 CrossRef.
- L. Peng, H. Zhu, H. Wang, Z. Guo, Q. Wu and C. Yang,
et al., Hydrodynamic tearing of bacteria on nanotips for sustainable water disinfection, Nat. Commun., 2023, 14(1), 5734 CrossRef CAS PubMed.
-
M. S. K. Rai, Principles and Practices in Water and Wastewater Engineering, Academic Guru Publishing House, 2024 Search PubMed.
- K. Castro and R. Abejón, Removal of Heavy Metals from Wastewaters and Other Aqueous Streams by Pressure-Driven Membrane Technologies: An Outlook on Reverse Osmosis, Nanofiltration, Ultrafiltration and Microfiltration Potential from a Bibliometric Analysis, Membranes, 2024, 14(8), 180 CrossRef CAS PubMed.
- G. Fadillah, N. T. Alarifi, I. W. K. Suryawan and T. A. Saleh, Advances in designed reactors for water treatment process: A review highlighting the designs and performance, J. Water Proc. Eng., 2024, 63, 105417 CrossRef.
- N. Maroufi and N. Hajilary, Nanofiltration membranes types and application in water treatment: a review, Sustain. Water Resour. Manag., 2023, 9(5), 142 CrossRef.
- J. Zhang, G. Li, X. Yuan, P. Li, Y. Yu and W. Yang,
et al., Reduction of ultrafiltration membrane fouling by the pretreatment removal of emerging pollutants: a review, Membranes, 2023, 13(1), 77 CrossRef CAS PubMed.
- A. Politano, R. A. Al-Juboori, S. Alnajdi, A. Alsaati, A. Athanassiou and M. Bar-Sadan,
et al., roadmap on membrane desalination technology at the water-energy nexus, J. Phys.: Energy, 2024, 6(2), 021502 CAS.
- J. Yang, Y. Liu, J. Zhao, H. Wang, G. Li and H. Liang, Controlling ultrafiltration membrane fouling in surface water treatment via combined pretreatment of O3 and PAC: mechanism investigation on impacts of technological sequence, Sci. Total Environ., 2023, 895, 165168 CrossRef CAS.
- B. Liu, Y. Jun, C. Zhao, C. Zhou, T. Zhu and S. Shao, Using Fe (II)/Fe (VI) activated peracetic acid as pretreatment of ultrafiltration for secondary effluent treatment: water quality improvement and membrane fouling mitigation, Water Res., 2023, 244, 120533 CrossRef CAS PubMed.
- B. Ma, Q. Ke and M. Ulbricht, Simultaneous removal of natural organic matters and copper (II) with ultrafiltration for drinking water treatment, J. Membr. Sci., 2023, 671, 121408 CrossRef CAS.
- E. Skoronski, A. B. Rosa and F. J. Simioni, Is conventional drinking water treatment more economically viable than ultrafiltration in Brazil? A technical, economic, comparative study with risk assessment, Water Supply, 2024, 24(6), 2092–2104 CrossRef.
- Q. Peng, J. Yu, Y. Pang, L. Deng, J. Tang and J. Wang,
et al., Prospects of advanced oxidation processes for high-salinity coking wastewater treatment: A strategy to support sustainable management, Resour. Conserv. Recycl., 2025, 212, 107880 CrossRef CAS.
- S. O. Ganiyu, S. Sable and M. G. El-Din, Advanced oxidation processes for the degradation of dissolved organics in produced water: A review of process performance, degradation kinetics and pathway, Chem. Eng. J., 2022, 429, 132492 CrossRef CAS.
- J. G. Mahy, C. Wolfs, C. Vreuls, S. Drot, S. Dircks and A. Boergers,
et al., Advanced oxidation processes for waste water treatment: From laboratory-scale model water to on-site real waste water, Environ. Technol., 2021, 42(25), 3974–3986 CrossRef CAS PubMed.
-
K. Agrawal and P. Verma, Advanced oxidative processes: An overview of their role in treating various wastewaters, Advanced Oxidation Processes for Effluent Treatment Plants, 2021, pp. 87–102 Search PubMed.
-
Treatment of waste water from a winery with an advanced oxidation process (AOP), IOP Conference Series: Earth and Environmental Science, ed. N. Ippolito, S. Zueva, F. Ferella, V. Corradini, E. Baturina and F. Vegliò, IOP Publishing, 2021 Search PubMed.
-
S. H. Khan and V. K. Yadav, Advanced oxidation processes for wastewater remediation: An overview, Removal of emerging contaminants through microbial processes, 2021, pp. 71–93 Search PubMed.
- N. Guettai, Y. Kadmi, M. Puri, K. Kerkich and B. Bouargane, Occurrence, analysis and removal processes of emerging pharmaceuticals from waters for the protection and preservation of a sustainable environment: a review, J. Clean. Prod., 2024, 142654 CrossRef CAS.
- M. E. Fawzy, H. M. Ahmed and H. F. Nassar, Advanced Treatment Technologies for Pollutants Removal in Wastewater, Key Eng. Mater., 2024, 983, 99–115 Search PubMed.
- M. H. Dehghani, S. Ahmadi, S. Ghosh, M. S. Khan, A. Othmani and W. A. Khanday,
et al., Sustainable remediation technologies for removal of pesticides as organic micro-pollutants from water environments: A review, Appl. Surf. Sci. Adv., 2024, 19, 100558 CrossRef.
- A. E. Gahrouei, S. Vakili, A. Zandifar and S. Pourebrahimi, From wastewater to clean water: Recent advances on the removal of metronidazole, ciprofloxacin, and sulfamethoxazole antibiotics from water through adsorption and advanced oxidation processes (AOPs), Environ. Res., 2024, 119029 CrossRef CAS PubMed.
- A. K. Thakur, R. Kumar, A. Kumar, R. Shankar, N. A. Khan and K. N. Gupta,
et al., Pharmaceutical waste-water treatment via advanced oxidation based integrated processes: An engineering and economic perspective, J. Water Proc. Eng., 2023, 54, 103977 CrossRef.
- Y. Yang,
et al. Recent advances in application of graphitic carbon nitride-based catalysts for degrading organic contaminants in water through advanced oxidation processes beyond photocatalysis: a critical review, Water Res., 2020, 184, 116200 CrossRef CAS PubMed.
- F. Rodrigues-Silva, C. S. Santos, J. A. Marrero, R. Montes, J. B. Quintana and R. Rodil,
et al., Continuous UV-C/H2O2 and UV-C/Chlorine applied to municipal secondary effluent and nanofiltration retentate: Removal of contaminants of emerging concern, ecotoxicity, and reuse potential, Chemosphere, 2024, 361, 142355 CrossRef CAS PubMed.
- D. Miklos, W.-L. Wang, K. Linden, J. Drewes and U. Hübner, Comparison of UV-AOPs (UV/H2O2, UV/PDS and UV/Chlorine) for TOrC removal from municipal wastewater effluent and optical surrogate model evaluation, Chem. Eng. J., 2019, 362, 537–547 CrossRef CAS.
- M. Farzanehsa, L. C. Vaughan, A. Zamyadi and S. J. Khan, Comparison of UV-Cl and UV-H2O2 advanced oxidation processes in the degradation of contaminants from water and wastewater: A review, Water Environ. J., 2023, 37(4), 633–643 CrossRef CAS.
- I. Sánchez-Montes, G. O. Santos, A. J. Dos Santos, C. H. Fernandes, R. S. Souto and P. Chelme-Ayala,
et al., Toxicological aspect of water treated by chlorine-based advanced oxidation processes: A review, Sci. Total Environ., 2023, 878, 163047 CrossRef PubMed.
-
J. Li, Applications for Advanced Oxidation of Drinking Water Using UV Leds, 2024 Search PubMed.
-
K. Guo, Z. Wu, J. Fang. UV-based advanced oxidation process for the treatment of pharmaceuticals and personal care products. Contaminants of Emerging Concern in Water and Wastewater: Elsevier; 2020. p. 367–408 Search PubMed.
- B. Zhang, X. Wang, Z. Fang, S. Wang, C. Shan and S. Wei,
et al., Unravelling molecular transformation of dissolved effluent organic matter in UV/H2O2, UV/persulfate, and UV/chlorine processes based on FT-ICR-MS analysis, Water Res., 2021, 199, 117158 CrossRef CAS.
- D. Singh, D. Singh, V. Mishra, J. Kushwaha, M. Sengar and S. Sinha,
et al., Strategies for biological treatment of waste water: A critical review, J. Clean. Prod., 2024, 142266 CrossRef CAS.
- Z. Aslam, P. Alam, R. Islam, A. H. Khan, H. Samaraweera and A. Hussain,
et al., Recent developments in moving bed biofilm reactor (MBBR) for the treatment of phenolic wastewater-A review, J. Taiwan Inst. Chem. Eng., 2024, 105517 Search PubMed.
- R. K. Parihar, P. K. Burnwal, S. P. Chaurasia and M. O. Midda, Unveiling the evolution of anaerobic membrane bioreactors: applications, fouling issues, and future perspective in wastewater treatment, Rev. Environ. Sci. Bio/Technol., 2024, 1–40 Search PubMed.
-
A. Kumar, T. Chalotra, A. K. Pathak and N. Raina, Role of microbes in wastewater treatment and Energy generation potentials: a sustainable approach, Microbial Biotechnology: Role in Ecological Sustainability and Research, 2022, pp. 265–311 Search PubMed.
- C. Prasse, D. Stalter, U. Schulte-Oehlmann, J. Oehlmann and T. A. Ternes, Spoilt for choice: A critical review on the chemical and biological assessment of current wastewater treatment technologies, Water Res., 2015, 87, 237–270 CrossRef CAS PubMed.
-
Y. Dzihora, K. A. da Silva, K. Korczyk, A. B. T. Nelabhotla, L. A. Kjeldsberg, R. Rasooli, et al., Granular and moving bed biofilm reactor-based wastewater treatment plant: an industrial perspective, Material-Microbes Interactions, Elsevier, 2023, pp. 439–468 Search PubMed.
- N. Mpongwana and S. Rathilal, Exploiting Biofilm Characteristics to Enhance Biological Nutrient Removal in Wastewater Treatment Plants, Appl. Sci., 2022, 12(15), 7561 CrossRef CAS.
- C.-L. Su, S. H. Lau, H.-Y. Yeh and Y.-T. Chang, Biological treatment of benzophenone-type UV filter wastewater in a sequencing batch reactor (SBR), Int. Biodeterior. Biodegrad., 2023, 177, 105534 CrossRef CAS.
- E. El Bestawy, S. M. Easa and D. Freez, Microbial Remediation of Dairy Industrial Wastewater Using Batch Mode Moving Bed Biofilm Reactor (MBBR), Egypt. Acad. J. Biol. Sci., G Microbiol., 2022, 14(2), 57–75 Search PubMed.
- S. Madan, R. Madan and A. Hussain, Advancement in biological wastewater treatment using hybrid moving bed biofilm reactor (MBBR): a review, Appl. Water Sci., 2022, 12(6), 141 CrossRef CAS.
- R. Boavida-Dias, J. R. Silva, A. D. Santos, R. C. Martins, L. M. Castro and R. M. Quinta-Ferreira, A comparison of biosolids production and system efficiency between activated sludge, moving bed biofilm reactor, and sequencing batch moving bed biofilm reactor in the dairy wastewater treatment, Sustainability, 2022, 14(5), 2702 CrossRef CAS.
- A. Dan, C.-x. Chen, M.-y Zou, Y.-y Deng, X.-m Zhang and J.-j Du,
et al., Removal efficiency, kinetic, and behavior of antibiotics from sewage treatment plant effluent in a hybrid constructed wetland and a layered biological filter, J. Environ. Manage., 2021, 288, 112435 CrossRef PubMed.
- K. Piaskowski, R. Świderska-Dąbrowska and T. Dąbrowski, Impact of cationic polyelectrolytes on activated sludge morphology and biological wastewater treatment in a Sequential Batch Reactor (SBR), J. Water Proc. Eng., 2023, 52, 103500 CrossRef.
- K. O. El, G. Lesage, F. Zaviska, A. Sauvêtre, M. Heran and F. Lestremau, Synergistic approach for enhanced wastewater treatment: Harnessing the potential of bioelectrochemical systems in integration with anaerobic membrane bioreactors, J. Environ. Chem. Eng., 2024, 113162 Search PubMed.
- P. Thamarai, R. Kamalesh, A. Saravanan, P. Swaminaathan and V. Deivayanai, Emerging trends and promising prospects in nanotechnology for improved remediation of wastewater contaminants: Present and future outlooks, Environ. Nanotechnol. Monit. Manag., 2024, 100913 CAS.
- X. Huang, M. Auffan, M. J. Eckelman, M. Elimelech, J.-H. Kim and J. Rose,
et al., Trends, risks and opportunities in environmental nanotechnology, Nat. Rev. Earth Environ., 2024, 1–16 Search PubMed.
- O. S. Olugbenga, P. G. Adeleye, S. B. Oladipupo, A. T. Adeleye and K. I. John, Biomass-derived biochar in wastewater treatment-a circular economy approach, Waste Manag. Bull., 2024, 1(4), 1–14 CrossRef.
-
S. Shangdiar, 12 Circular Resource Economy Recovery on from Industrial Wastewater, Circular Economy: Applications for Water Remediation, 2024, p. 209 Search PubMed.
- M. Ateia, H. Wei and S. Andreescu, Sensors for Emerging Water Contaminants: Overcoming Roadblocks to Innovation, Environ. Sci. Technol., 2024, 58(6), 2636–2651 CrossRef CAS PubMed.
- R. S. Dhamorikar, V. G. Lade, P. V. Kewalramani and A. B. Bindwal, Review on integrated advanced oxidation processes for water and wastewater treatment, J. Ind. Eng. Chem., 2024, 104–122 CrossRef.
- A. S. Stasinakis, Use of selected advanced oxidation processes (AOPs) for wastewater treatment–a mini review, Global NEST J., 2008, 10(3), 376–385 Search PubMed.
-
M. A. Abdulhamid, N. Alqadhi, C. Osornio and G. Szekely, Green Nanotechnology for Water Purification, Nanomaterials for Air-and Water Purification, 2024, pp. 77–114 Search PubMed.
- H. Li, B. Zhang and Y. Wu, Highly efficient removal of emulsified oil from oily wastewater by microfiltration carbon membranes made from phenolic resin/coal, Environ. Technol., 2024, 45(18), 3692–3705 CrossRef CAS.
- H. Salvadó, O. Canals, B. Pérez-Uz, L. Arregui and S. Serrano, Deactivation of internal recirculation in a full-scale Biological Nutrient Removal (BNR) Wastewater Treatment Plant (WWTP) reveals outstanding microeukaryotic bioindicators, J. Water Proc. Eng., 2024, 57, 104669 CrossRef.
-
S. Dhara, N. S. Samanta, P. P. Das and M. K. Purkait, Biological nitrogen removal and recovery from wastewater, Development in Waste Water Treatment Research and Processes, Elsevier, 2024, pp. 159–179 Search PubMed.
- S. X. de Campos and M. Soto, The Use of Constructed Wetlands to Treat Effluents for Water Reuse, Environments, 2024, 11(2), 35 CrossRef.
- L. Verhaeghe, J. Verwaeren, G. Kirim, S. Daneshgar, P. A. Vanrolleghem and E. Torfs, Towards good modelling practice for parallel hybrid models for wastewater treatment processes, Water Sci. Technol., 2024, 89(11), 2971–2990 CrossRef CAS.
-
Internet of Things Based Real Time Monitoring and Control of Industrial Waste Water, 2022 5th International Conference on Contemporary Computing and Informatics (IC3I), ed. D. Kumar, S. Kumar and P. Anand, IEEE, 2022 Search PubMed.
- B. B. Ashagre, G. Fu and D. Butler, Automation and real-time control of urban wastewater systems: a review of the move towards sustainability, J. Water Supply: Res. Technol. – AQUA, 2020, 69(8), 751–768 CrossRef.
-
Towards real-time monitoring and control of water networks, 2022 American Control Conference (ACC), ed. A. Elkhashap, D. Rüschen and D. Abel, IEEE, 2022 Search PubMed.
-
Real-time implementation of wastewater monitoring system on the communal wastewater treatment plant using the IoT technology, IOP Conference Series: Earth and Environmental Science, ed. A. Soetedjo, E. Hendriarianti, S. Wibowo, J. Novrian, A. Nugroho, M. Roby, et al., IOP Publishing, 2022 Search PubMed.
- Z. Parsa, R. Dhib and M. Mehrvar, Dynamic Modelling, Process Control, and Monitoring of Selected Biological and Advanced Oxidation Processes for Wastewater Treatment: A Review of Recent Developments, Bioengineering, 2024, 11(2), 189 CrossRef CAS PubMed.
- M. Alidoust, Y. Saito, H. Takada, K. Mizukawa, T. Karlsson and S. Brosché,
et al., A Unique Monitoring Method for Fecal and Sewage-Derived Chemical Pollution Utilizing International Pellet Watch Approach, Environ. Sci. Technol., 2024, 58(10), 4761–4771 CrossRef CAS PubMed.
- A. Thakur and P. Devi, A comprehensive review on water quality monitoring devices: materials advances, current status, and future perspective, Crit. Rev. Anal. Chem., 2024, 54(2), 193–218 CrossRef CAS.
- A. Moretti, H. L. Ivan and J. Skvaril, A review of the state-of-the-art wastewater quality characterization and measurement technologies. Is the shift to real-time monitoring nowadays feasible?, J. Water Proc. Eng., 2024, 60, 105061 CrossRef.
-
Integrated water monitoring and control system-IWMCS, 2020 IEEE Bangalore Humanitarian Technology Conference (B-HTC), ed. S. Hattaraki, A. Patil and S. Kulkarni, IEEE, 2020 Search PubMed.
- G. O. Baş and M. A. Köksal, Environmental and techno-economic analysis of the integration of biogas and solar power systems into urban wastewater treatment plants, Renewable Energy, 2022, 196, 579–597 CrossRef.
- S. K. Bhatia, H.-S. Joo and Y.-H. Yang, Biowaste-to-bioenergy using biological methods–a mini-review, Energy Convers. Manag., 2018, 177, 640–660 CrossRef CAS.
- Y. Shen, J. L. Linville, M. Urgun-Demirtas, M. M. Mintz and S. W. Snyder, An overview of biogas production and utilization at full-scale wastewater treatment plants (WWTPs) in the United States: challenges and opportunities towards energy-neutral WWTPs, Renew. Sustain. Energy Rev., 2015, 50, 346–362 CrossRef CAS.
- A. Tabasová, J. Kropáč, V. Kermes, A. Nemet and P. Stehlík, Waste-to-energy technologies: Impact on environment, Energy, 2012, 44(1), 146–155 CrossRef.
- J. A. Silva, Wastewater treatment and reuse for sustainable water resources management: a systematic literature review, Sustainability, 2023, 15(14), 10940 CrossRef CAS.
- T. Gao, K. Xiao, J. Zhang, X. Zhang, X. Wang and S. Liang,
et al., Cost-benefit analysis and technical efficiency evaluation of full-scale membrane bioreactors for wastewater treatment using economic approaches, J. Clean. Prod., 2021, 301, 126984 CrossRef CAS.
- S. Gabarrón, M. Dalmau, J. Porro, I. Rodriguez-Roda and J. Comas, Optimization of full-scale membrane bioreactors for wastewater treatment through a model-based approach, Chem. Eng. J., 2015, 267, 34–42 CrossRef.
- T. U. Rahman, H. Roy, M. R. Islam, M. Tahmid, A. Fariha and A. Mazumder,
et al., The advancement in membrane bioreactor (MBR) technology toward sustainable industrial wastewater management, Membranes, 2023, 13(2), 181 CrossRef CAS.
- G. Zhang,
et al. Recent advances in constructed wetland for wastewater treatment, Bioresour. Technol., 2021, 321, 124378 CrossRef CAS.
- D. Q. Zhang, K. Jinadasa, R. M. Gersberg, Y. Liu, W. J. Ng and S. K. Tan, Application of constructed wetlands for wastewater treatment in developing countries–a review of recent developments (2000–2013), J. Environ. Manag., 2014, 141, 116–131 CrossRef CAS.
-
Commission WR, Wastewater Treatment Technologies: A Basic Guide, Water Research Commission: Gezina, South Africa, 2016 Search PubMed.
- C. T. Finnerty, A. E. Childress, K. M. Hardy, E. M. Hoek, M. S. Mauter and M. H. Plumlee,
et al., The Future of Municipal Wastewater Reuse Concentrate Management: Drivers, Challenges, and Opportunities, Environ. Sci. Technol., 2023, 58(1), 3–16 CrossRef PubMed.
- Q. Mahmood, A. Pervez, B. S. Zeb, H. Zaffar, H. Yaqoob and M. Waseem,
et al., Natural treatment systems as sustainable ecotechnologies for the developing countries, BioMed Res. Int., 2013, 2013(1), 796373 Search PubMed.
- A. Malviya and D. Jaspal, Artificial intelligence as an upcoming technology in wastewater treatment: a comprehensive review, Environ. Technol. Rev., 2021, 10(1), 177–187 CrossRef.
- D. Elalami, H. Carrere, F. Monlau, K. Abdelouahdi, A. Oukarroum and A. Barakat, Pretreatment and co-digestion of wastewater sludge for biogas production: Recent research advances and trends, Renew. Sustain. Energy Rev., 2019, 114, 109287 CrossRef CAS.
- P. Neumann, S. Pesante, M. Venegas and G. Vidal, Developments in pre-treatment methods to improve anaerobic digestion of sewage sludge, Rev. Environ. Sci. Bio/Technol., 2016, 15, 173–211 CrossRef CAS.
- E. Sarikaya and G. N. Demirer, Biogas production from broiler manure, wastewater treatment plant sludge, and greenhouse waste by anaerobic co-digestion, J. Renewable Sustainable Energy, 2013, 5, 043126 CrossRef.
-
N. Fayad, The Application of Electrocoagulation Process for Wastewater Treatment and for the Separation and Purification of Biological Media, Université Clermont Auvergne [2017–2020], 2017 Search PubMed.
-
G. A. Vazquez, An Electrochemical Process for Decentralized Treatment of High-Strength Wastewater, Michigan State University, 2023 Search PubMed.
-
A. Ghaly, R. Emam, E. Abdelrahman, M. El, M. Sayed, E. Mostafa, et al., Physical, Chemical and Biological Greywater Treatment Technologies for Effective Reuse: Critical and Comparative Analyses, 2021 Search PubMed.
- M. Coha, G. Farinelli, A. Tiraferri, M. Minella and D. Vione, Advanced oxidation processes in the removal of organic substances from produced water: Potential, configurations, and research needs, Chem. Eng. J., 2021, 414, 128668 CrossRef CAS.
- A. Giwa, A. Yusuf, H. A. Balogun, N. S. Sambudi, M. R. Bilad and I. Adeyemi,
et al., Recent advances in advanced oxidation processes for removal of contaminants from water: A comprehensive review, Process Saf. Environ. Prot., 2021, 146, 220–256 CrossRef CAS.
- S. Rathod, S. Preetam, C. Pandey and S. P. Bera, Exploring synthesis and applications of green nanoparticles and the role of nanotechnology in wastewater treatment, Biotechnol. Rep., 2024, e00830 CrossRef CAS.
- S. Sharma, P. Dhingra and S. Jain, Advancements & challenges of nanotechnology in waste water treatment, Mater. Today: Proc., 2023, 80, 18–23 CAS.
- J. Canals, A. Cabrera-Codony, O. Carbó, M. Baldi, B. Gutiérrez and A. Ordoñez,
et al., Nutrients removal by high-rate activated sludge and its effects on the mainstream wastewater treatment, Chem. Eng. J., 2024, 479, 147871 CrossRef CAS.
- Z. Zhang, Y. Wang, X. Wang, Y. Zhang, T. Zhu and L. Peng,
et al., Towards scaling-up implementation of polyhydroxyalkanoate (PHA) production from activated sludge: Progress and challenges, J. Clean. Prod., 2024, 141542 CrossRef CAS.
- S. Nath, Electrochemical Wastewater Treatment Technologies Through Life Cycle Assessment: A Review, ChemBioEng Rev., 2024, 11(4), e202400016 CrossRef CAS.
- H. Boleydei and C. Vaneeckhaute, Advancements and challenges in membrane bioreactor performance for decentralized and low-temperature applications: A comprehensive review, J. Water Proc. Eng., 2024, 63, 105523 CrossRef.
- D. P.-H. Tran, S.-J. You, X.-T. Bui, Y.-F. Wang and A. Ramos, Anaerobic membrane bioreactors for municipal wastewater: Progress in resource and energy recovery improvement approaches, J. Environ. Manage., 2024, 366, 121855 CrossRef PubMed.
- J. Jayaraman, J. Kumaraswamy, Y. K. Rao, M. Karthick, S. Baskar and M. Anish,
et al., Wastewater treatment by algae-based membrane bioreactors: a review of the arrangement of a membrane reactor, physico-chemical properties, advantages and challenges, RSC Adv., 2024, 14(47), 34769–34790 RSC.
- E. A. Hamedani, A. Abasalt and S. Talebi, Application of microbial fuel cells in wastewater treatment and green energy production: A comprehensive review of technology fundamentals and challenges, Fuel, 2024, 370, 131855 CrossRef.
- X. Zhang, Y. Lin, H. Lin and J. Yan, Constructed wetlands and hyperaccumulators for the removal of heavy metal and metalloids: A review, J. Hazard. Mater., 2024, 135643 CrossRef CAS.
- G. Chen, Y. Mo, X. Gu, E. Jeppesen, T. Xie and Z. Ning,
et al., Sustainability of global small-scale constructed wetlands for multiple pollutant control, npj Clean Water, 2024, 7(1), 45 CrossRef CAS.
- A. J. Peralta Vega, V. Vergara Flórez, O. Marín-Peña, S. G. García-Aburto and L. C. Sandoval Herazo, Treatment of Domestic Wastewater in Colombia Using Constructed Wetlands with Canna Hybrids and Oil Palm Fruit Endocarp, Water, 2024, 16(16), 2290 CrossRef.
- R. Alsabt, W. Alkhaldi, Y. A. Adenle and H. M. Alshuwaikhat, Optimizing waste management strategies through artificial intelligence and machine learning-An economic and environmental impact study, Clean. Waste Syst., 2024, 8, 100158 CrossRef.
- K. L. Yu, H. C. Ong and H. B. Zaman, Integrated energy informatics technology on microalgae-based wastewater treatment to bioenergy production: A review, J. Environ. Manage., 2024, 368, 122085 CrossRef CAS PubMed.
- D. Narayanan, M. Bhat, N. Samual, N. Khatri and A. Saroliya, Artificial intelligence driven advances in wastewater treatment: Evaluating techniques for sustainability and efficacy in global facilities, Desalination Water Treat., 2024, 100618 CrossRef.
- L. Engstam, L. Janke, C. Sundberg and Å. Nordberg, Optimising power-to-gas integration with wastewater treatment and biogas: A techno-economic assessment of CO2 and by-product utilisation, Appl. Energy, 2025, 377, 124534 CrossRef CAS.
- D. A. Jadhav, Z. Yu, M. Hussien, J.-H. Kim, W. Liu and T. Eisa,
et al., Paradigm shift in NEW (Nutrient-Energy-Water) sustainable wastewater
treatment system through synergy of bioelectrochemical system and anaerobic digestion, Bioresour. Technol., 2024, 130404 CrossRef CAS.
- K. Archana, A. Visckram, P. S. Kumar, S. Manikandan, A. Saravanan and L. Natrayan, A review on recent technological breakthroughs in anaerobic digestion of organic biowaste for biogas generation: Challenges towards sustainable development goals, Fuel, 2024, 358, 130298 CrossRef CAS.
- M. Zielińska and K. Bułkowska, Sustainable Management and Advanced Nutrient Recovery from Biogas Energy Sector Effluents, Energies, 2024, 17(15), 3705 CrossRef.
-
E. Abdollahzadeh Sharghi, M. Farzin and M. Talaeian Earaqi, Gaining Comprehensive Insight into the Effect of Electrocoagulation Integrated in a Membrane Bioreactor on the Detergent Manufacturing Plant Wastewater Treatment and Membrane Fouling, 2024, pp. 1–51 Search PubMed.
- P. T. P. Aryanti, F. A. Nugroho, C. Phalakornkule and A. Kadier, Energy efficiency in electrocoagulation processes for sustainable water and wastewater treatment, J. Environ. Chem. Eng., 2024, 114124 CrossRef CAS.
-
R. M. Katika, R. N. Mandapati, S. Tondepu, S. B. Davuluri and S. Boddu, Perspectives of Biochar-Aided Advanced Oxidation Processes for the Remediation of Emerging Dyeing Contaminants, 2024 Search PubMed.
- V. Fabregat and J. M. Pagán, Technical–Economic Feasibility of a New Method of Adsorbent Materials and Advanced Oxidation Techniques to Remove Emerging Pollutants in Treated Wastewater, Water, 2024, 16(6), 814 CrossRef CAS.
- T. P. Brdarić, D. D. Aćimović, Ľ. Švorc and D. D. Vasić Anićijević, Bibliometric Study of Electrochemical Advanced Oxidation Processes (EAOPs) for Wastewater Treatment, Coatings, 2024, 14(8), 1060 CrossRef.
- S. Pandit, N. Yadav, P. Sharma, A. Prakash and A. Kuila, Life cycle assessment and techno-economic analysis of nanotechnology-based wastewater treatment: Status, challenges and future prospectives, J. Taiwan Inst. Chem. Eng., 2024, 105567 Search PubMed.
- H. Lu, L. Hou, Y. Zhang, X. Cao, X. Xu and Y. Shang, Pilot-scale and large-scale Fenton-like applications with nano-metal catalysts: from catalytic modules to scale-up applications, Water Res., 2024, 122425 CrossRef CAS.
- N. O. Solomon, S. Kanchan and M. Kesheri, Nanoparticles as Detoxifiers for Industrial Wastewater, Water, Air, Soil Pollut., 2024, 235(3), 214 CrossRef CAS.
|
This journal is © The Royal Society of Chemistry 2025 |
Click here to see how this site uses Cookies. View our privacy policy here.