Synthesis of novel diesters as potential fuel oxygenates and surfactants of renewable origin from carbohydrate-derived 5-(chloromethyl)furfural†
Received
10th September 2024
, Accepted 20th December 2024
First published on 20th December 2024
Abstract
5-(Chloromethyl)furfural (CMF) has received enormous interest over the past two decades as a carbohydrate-derived platform chemical for synthesizing organic chemicals of commercial significance. This work reports a general synthetic protocol for synthesizing several known and novel mono- and diesters of CMF with potential applications as chemical intermediates, neutral surfactants, and plasticizers. The functional groups on CMF were selectively activated using relatively innocuous reagents, and the products were isolated with satisfactory yields (79–90%). The three-step process starts by oxidizing the aldehyde group into a carbonyl chloride using tert-butyl hypochlorite as a selective oxidant. The resulting carbonyl chloride was reacted with an alcohol reagent in the same pot to form the monoesters. The chloromethyl group was then reacted with the triethylammonium salt of a carboxylic acid by a nucleophilic substitution reaction to prepare the diesters. The reactions were optimized for temperature, molar ratio of reagents, and solvents. Depending on the choice of alcohol and the carboxylic acid reagents, the mono- and diester products can be made entirely biorenewable.
Sustainability spotlight
Cellulosic biomass plays a crucial role in the carbon-neutral bioeconomy for the sustainable production of transportation fuels, organic chemicals, and synthetic polymers. Cellulose-derived 5-(chloromethyl)furfural (CMF) is a prominent platform chemical that can be transformed into various classes of organic chemicals of commercial significance. This work reports the synthesis of various monoesters and diesters of CMF with potential applications as fuel oxygenates, surfactants, and plasticizers. The reactions were performed under organic solvent-free conditions, ensuring good to excellent isolated yields of the targeted esters. The oxidative esterification step was performed using tert-butyl hypochlorite (TBHC) as a selective, inexpensive, and relatively non-toxic oxidant. TBHC is produced from household bleach and tert-butanol. The carbonyl chloride intermediate was then reacted with alcohols at a slightly elevated temperature to produce the ester. The chloromethyl group in the monoesters was nucleophilically substituted by reacting with the triethylammonium salt of carboxylic acids. Many of the alcohols and carboxylic acids chosen in this study can be produced from biomass through chemical-catalytic or enzymatic pathways. Therefore, the diesters produced from CMF can be made entirely biorenewable by choosing the appropriate alcohol and carboxylic acid reagents. The general synthetic protocol developed in this work is high-yielding, scalable, and cost-effective. Our work emphasizes the importance of the following UN sustainable development goals: affordable and clean energy (SDG 7), industry, innovation, and infrastructure (SDG 9), ensuring sustainable consumption (SDG 12), and climate action (SDG 13).
|
1. Introduction
Over the past three decades, serious deliberations have been made to identify a sustainable carbon-based feedstock to defossilize, at least partially, the organic chemical industry.1,2 Biomasses, especially the terrestrial lignocellulosic and algal biomass feedstock, have shown promise owing to the social, economic, and environmental incentives associated with converting them into organic chemicals and materials.3 5-(Hydroxymethyl)furfural (HMF) a carbohydrate-derived platform chemical, is at the forefront of biorefinery research.4 HMF is produced by the acid-catalyzed dehydration of abundant hexose sugars (e.g., glucose and sucrose), polymeric carbohydrates (e.g., starch and cellulose), and untreated cellulosic biomass.4 The first synthesis of HMF from carbohydrates was reported over a century ago. However, it has received renewed attention since the early 2000s as a platform chemical in carbohydrate-centric biorefineries.5 HMF has been synthetically upgraded into various classes of organic chemicals of commercial significance following eco-friendly pathways.6 However, despite years of research, the scalability and techno-economic feasibility of HMF production from cellulosic biomass remains questionable due to its inherent hydrophilicity and hydrolytic instability in aqueous acids.7 Although high isolated yields of HMF can be obtained from simple sugars, satisfactory yields of HMF from polymeric carbohydrates and untreated biomass require special reaction conditions.7 The commercial feasibility of producing HMF derivatives in a biorefinery setting is intimately linked with the scalability and process economics of HMF production. In this regard, various hydrophobic analogs of HMF have received serious attention over the past decade as they can be produced directly from isolated carbohydrates and untreated biomass.8 These analogs have significantly better thermal, storage, and hydrolytic stability than HMF. Moreover, due to the hydrophobicity of these HMF analogs, they can be conveniently extracted from aqueous or polar reaction media using a suitable organic solvent. 5-(Chloromethyl)furfural (CMF) is a hydrophobic analog of HMF that has received maximum attention.9 CMF can be produced from carbohydrates and cellulosic biomass using media with a high concentration of chloride ions, such as concentrated aqueous hydrochloric acid and/or chloride salt.10,11 High isolated yields of CMF can be achieved from polymeric carbohydrates and untreated cellulosic biomass.12 CMF production has been attempted in batch, semi-batch, and continuous flow reactors.10,13,14 The derivative chemistry of CMF has expanded over the past several years, encompassing virtually all the major classes of organic products. Moreover, CMF can be conveniently hydrolyzed into HMF when required, and all the derivative chemistry of the latter can be accessed.15 Unlike HMF, the commercial production of CMF from cellulosic biomasses has been attempted by multiple ventures worldwide.9 CMF has three main reactive centers: the chloromethyl group, the aldehyde group, and the furan ring.16 Selective reagents (catalytic and stoichiometric) and reaction conditions have been developed to exploit one or more of these reaction centers for the synthetic upgrading of CMF. The chloromethyl group in CMF makes its reactivity different than that of HMF. For example, the etherification of HMF starts by protonating the hydroxymethyl group in HMF, whereas the esterification follows the Fischer esterification pathway.17,18 Kumar et al. reported a bifunctional ionic liquid catalyst 1-sulfonic acid-3-methylimidazolium tetrachloroferrate [SMIM][FeCl4], containing Brønsted and Lewis acidic sites, for esterifying HMF with biogenic carboxylic acids.18 Moderate to good yields (53–78%) of the HMF esters were obtained under optimized conditions. Tiwari et al. esterified HMF with LA using tin-exchanged tungstophosphoric acid supported on montmorillonite clay (K-10) as a heterogeneous acid catalyst.19 The catalyst showed high activity, selectivity, and recyclability. An 89% yield of HMF–levulinate was ensured under optimized parameters (1
:
5 molar ratio of HMF and LA, 20 wt% catalyst, 1000 rpm, 110 °C, and 2 h). The esterification and transesterification of HMF with carboxylic acid and esters were reported using immobilized lipase as a biocatalyst, and the HMF–esters were separated using a deep-eutectic solvent.20 HMF was transformed into 5-(acetoxymethyl)furfural (AcMF) by a transesterification reaction in the presence of an organic or inorganic base as a catalyst.21 Nilaphai et al. reported the synthesis of a series of monoesters of HMF with fatty acids using cyanuric chloride as the catalyst.22 Conversely, CMF reacts with alcohol or acids by nucleophilic substitution of the chloride. Moreover, CMF is considerably more stable than HMF in aqueous acids but decomposes rapidly upon coming in contact with many inorganic bases.23 Therefore, the synthetic protocol developed for certain HMF derivatives cannot be extrapolated to CMF directly.
The hydroxymethyl and the aldehyde groups in HMF can be esterified directly by oxidative esterification.24 Alternatively, in a two-step process, the aldehyde is first oxidized into a carboxylic acid, and the latter is then esterified following the Fischer esterification protocol. The hydroxymethyl group in HMF can be esterified by reacting with a carboxylic acid molecule using a suitable acid catalyst. The mono-and diesters of HMF have potential applications as renewable chemical intermediates, fuel oxygenates, surfactants, plasticizers, and even bioactive molecules.18,22,25,26 In some studies, HMF–esters are directly produced by the acid-catalyzed dehydration of sugars followed by concomitant esterification of the HMF formed in the reaction medium as a transient intermediate.27 Interestingly, many carboxylic acids used for making the HMF–esters can be sourced renewably from biomass.19 Therefore, the mono- and diesters of HMF can be made entirely biorenewable by carefully choosing the starting materials. We have recently reported the esterification of CMF (on the chloromethyl site) by reacting it with the triethylammonium salt of carboxylic acids.23 In an earlier report, CMF was esterified (on the aldehyde site) by a two-step process.28 In the first step, CMF was converted into 5-(chloromethyl)furan-2-carbonyl chloride (CMFCC) by reacting with tert-butyl hypochlorite (TBHC). In the second step, CMFCC was reacted with an alcohol to prepare the esters (Scheme 1). CMFCC showed significantly different reactivity compared to CMF, wherein the chloromethyl group resisted nucleophilic substitution. Interestingly, the chloromethyl group in CMF can be easily substituted with small-chain alkyl alcohols, even at room temperature. In this work, we report the synthesis of novel diesters of CMF using selective, sequential esterification of its side chains. The products were isolated in good to excellent isolated yields, and chromatographic purification was often avoided. This work adds to the recent efforts by several research groups worldwide to synthesize sustainable organic chemicals using CMF as a biobased platform chemical. Moreover, the reactivity patterns for CMF and its derivatives discussed in this work will help expand its derivative chemistry and sustainably synthesize new products of commercial interest.
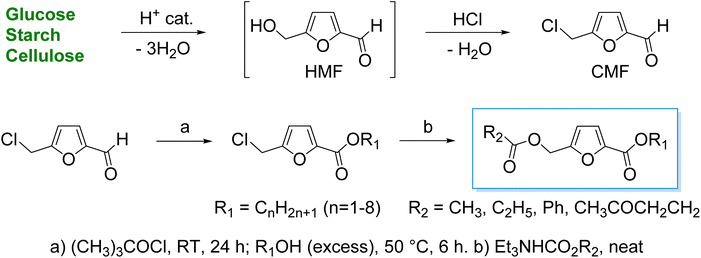 |
| Scheme 1 Synthesis of mono- and diesters of carbohydrate-derived CMF. | |
2. Experimental section
2.1 Materials
D-Fructose (99%), sodium sulfate (anhydrous, 99%), silica gel (60–120 mesh), anhydrous TEA (99%), levulinic acid (98%), 1-hexanol (98%), zinc chloride (anhydrous, 95%), and n-heptane (99%) were purchased from Spectrochem. Petroleum ether (60–80 °C, 99%), acetic acid glacial (>99%), propanoic acid (99%), benzoic acid (99.5%), aqueous hydrochloric acid (35%), DCE (99%), and 1-propanol (99.5%) were purchased from Loba Chemie Pvt. Ltd. 1-Butanol and tert-butanol were purchased from Molychem. Ethanol was supplied by Nirani Sugar Limited. 1-Pentanol (99%), 1-heptanol (99%), and 1-octanol (99%) were purchased from SRL. All the chemicals were used as such without further purification.
2.2 Instruments
The synthesized compounds were analyzed using Fourier transform infrared (FTIR) spectroscopy, nuclear magnetic resonance (NMR) spectroscopy, and the high-resolution mass spectrometry (HRMS) technique. The FTIR spectrum was acquired using the ATR method on a Bruker Alpha II FTIR spectrometer fitted with silicon carbide as an IR source. Each sample was scanned 24 times with a spectral resolution of 4 cm−1. The synthesized compounds were dissolved in deuterated chloroform solvent for NMR spectroscopy. The 1H-NMR spectra were acquired using a Bruker NanoBay® instrument operating at 300 MHz. The 13C-NMR spectra were acquired using the same instrument at a frequency of 75 MHz. Some NMR spectra were collected using 400 MHz and 800 MHz instruments. The HRMS data of the synthesized compounds were collected using a Xevo multi-reflecting time-of-flight (MRT) mass spectrometer (XEVO-G2-XS-QTOF).
2.3 Synthetic procedures
2.3.1 Synthesis of ethyl 5-(chloromethyl)furan-2-carboxylate.
CMF (2.000 g, 13.84 mmol) and tert-butyl hypochlorite (4.51 g, 41.50 mmol) were charged in a 50 mL round-bottomed flask wrapped with aluminum foil. The mixture was magnetically stirred at room temperature for 24 h under a nitrogen atmosphere. Absolute ethanol (10 mL) was added to the reaction mixture, and heated for 6 h at 50 °C. The reaction mixture was then cooled to room temperature and diluted with ethyl acetate (10 mL). The reaction mixture was rinsed with water (10 mL). The ethyl acetate layer was separated in a separatory funnel, dried over anhydrous Na2SO4, and evaporated in a rotary evaporator under reduced pressure to get the crude product. The crude product was purified using column chromatography (petroleum ether/ethyl acetate, silica gel 60–120 mesh) to get purified ethyl 5-(chloromethyl)furan-2-carboxylate 1 (2.166 g, 83%).28 The general synthesis strategy was extended to synthesize various alkyl 5-(chloromethyl)furan-2-carboxylates.
2.3.2 Synthesis of ethyl 5-(acetoxymethyl)furan-2-carboxylate.
Purified CMF monoester 1 (0.324 g, 1.72 mmol) was introduced into a round-bottomed flask (50 mL), and triethylammonium acetate (TEAA) (0.415 g, 2.58 mmol) was added in a single portion. The reaction mixture was placed in a pre-heated (80 °C) oil bath and magnetically stirred for 3 h. The progress of the reaction was monitored by thin-layer chromatography, and the reaction was stopped after complete conversion of 1. After completion, the reaction mixture was diluted with ethyl acetate (10 mL) and washed with a saturated aqueous sodium bicarbonate solution (10 mL). The ethyl acetate layer was separated in a separatory funnel, dried over anhydrous Na2SO4, and filtered through a plug of silica gel (60–120 mesh) to afford pure 1a (0.310 g, 85%).23 Alternatively, the crude mixture was purified by triturating in n-heptane and ethyl acetate (20 mL, 1
:
1, v/v). The synthetic protocol was extended to synthesize alkyl 5-(alkoxymethyl)furan-2-carboxylates.
2.3.3 One-pot synthesis of ethyl 5-(acetoxymethyl)furan-2-carboxylate from CMF.
CMF (0.500 g, 3.46 mmol) and tert-butyl hypochlorite (1.13 g, 10.37 mmol) were charged in a 50 mL round-bottomed flask wrapped with aluminium foil. The mixture was magnetically stirred at room temperature for 24 h under a nitrogen atmosphere. Absolute ethanol (3 mL) was added to the reaction mixture, and the reaction mixture was heated for 6 h at 50 °C. Excess ethanol was evaporated in a rotary evaporator under reduced pressure to get the crude ethyl 5-(chloromethyl)furan-2-carboxylate 1. Triethylammonium acetate (TEAA) (0.693 g, 4.31 mmol) and acetonitrile (5 mL) were introduced. The reaction mixture was placed in a pre-heated (80 °C) oil bath and magnetically stirred for 3 h. The progress of the reaction was monitored by thin-layer chromatography, and the reaction was stopped after the complete conversion of 1. After completion, the reaction mixture was triturated with n-heptane and ethyl acetate (20 mL, 1
:
1, v/v). Evaporation of the solvent under reduced pressure afforded pure 1a (0.440 g, 60%).
3. Results and discussion
The synthesis of alkyl 5-(alkoxymethyl)furan-2-carboxylates was attempted using a combination of oxidative esterification of the aldehyde group and nucleophilic substitution of the chloromethyl group in carbohydrate-derived CMF. In a previous report, the oxidative esterification of CMF was performed in a one-pot, two-step process. In the first step, CMF was selectively oxidized into CMFCC using TBHC as an inexpensive and selective oxidant. TBHC is a relatively stable organic hypochlorite soluble in common organic solvents. The reaction between CMF and TBHC can be performed at room temperature under organic solvent-free conditions. CMFCC is relatively stable and does not easily hydrolyze into the corresponding acid. CMFCC has two potential electrophilic sites, i.e., the acyl chloride and the chloromethyl group. However, the acyl chloride is significantly more reactive towards nucleophiles. Therefore, CMFC can be converted into esters by reacting with suitable alcohol reagents at slightly elevated temperatures. The chloromethyl group is unreactive towards alcohols at relatively low temperatures (<80 °C). This observation is contrary to the results obtained from CMF. Even at room temperature, the chloromethyl group in CMF can be easily substituted with alcohols, such as ethanol. Therefore, oxidation of the aldehyde group in CMF into an ester significantly alters the reactivity of the chloromethyl group. Ethyl 5-(chloromethyl)furan-2-carboxylate 1a, produced by reacting CMFCC with excess absolute ethanol in an 83% isolated yield, was then subjected to a reaction with excess glacial acetic acid under reflux. However, no substitution of the chloromethyl group in 1a was observed. This result can be explained by carboxylic acids being less nucleophilic than alcohols. Therefore, the chloromethyl group in 1 was reacted with triethylammonium acetate (TEAA), which was produced by reacting equimolar amounts of glacial acetic acid and triethylamine. Interestingly, the reaction was completed within 3 h at 80 °C, affording an 85% isolated yield of ethyl 5-(acetoxymethyl)furan-2-carboxylate 1a. The result may be explained by the improved nucleophilicity of the carboxylate ion compared to carboxylic acid. The use of sodium acetate or potassium acetate resulted in lower yields of 1a.
In an alternative approach, when the aldehyde group in AcMF was esterified by oxidative esterification, the acetoxy group was cleaved by ethanolysis in the second step. TBHC required for the crucial oxidation to form CMFCC from CMF was synthesized from commercial bleach (6.5% aqueous sodium hypochlorite) solution following a literature process. The reaction involved bleach solution, tert-butanol, and acetic acid under controlled parameters (10 °C, 8 min), and water-insoluble TBHC was phase-separated from the aqueous reaction medium.29 The reaction between CMF and TBHC proceeds through a radical mechanism, where the chlorine atom in TBHC gets incorporated into CMFCC, and tert-butanol is formed as the byproduct. As CMFCC is reacted with an alcohol (e.g., ethanol), the chlorine atom is released as HCl.
With the successful synthesis of 1 and 1a in satisfactory isolated yields, the substrate scope of the synthesis was explored. The proposed applications of these molecules include fuel oxygenates, surfactants, and plasticizers of renewable origin. Therefore, the synthesis of mono- and diesters with alcohols and carboxylic acids (aliphatic and aromatic) with different alkyl chain lengths was attempted. The short-chain (C1–C4) alkyl alcohols can be renewably produced from biomass following chemical-catalytic and enzymatic pathways. Analogously, acetic to butyric acids can also be produced from biomass. Levulinic acid (LA) used as a carboxylic acid reagent in this study, is obtained by the acid-hydrolysis of carbohydrates through the intermediary of HMF. Therefore, many of the diesters of CMF reported in this study can be produced exclusively using sustainable biogenic carbon. When CMFCC was reacted with alkyl alcohols of different chain lengths, only a slight change in reactivity was observed. With the increasing chain length of the alcohol reagent, a marginal increase in the yield of the corresponding monoester was observed. The improved nucleophilicity of alcohols with longer alkyl chain lengths justifies this observation. Interestingly, we found some CMF monoesters mentioned in the literature.30 However, the synthetic process for these compounds is obscure, and no spectroscopic characterization data were found for these compounds. Hence, these compounds were characterized by spectroscopic techniques (FTIR, 1H-NMR & 13C-NMR) and HRMS (Fig. S1–S105, ESI†). The synthesized monoesters exhibited excellent storage stability under ambient conditions. No observable decomposition of the monoesters was found even after four months. The excellent storage stability improves the potential of these compounds for targeted applications and as platform chemicals for downstream synthetic value addition.
The previously published literature reported the synthesis of 1 at room temperature using six equivalents of TBHC with respect to CMF. During this study, it was observed that only three equivalents of TBHC were enough to complete the reaction without compromising the yield of CMFCC. The reaction mixture containing CMFCC was initially quenched with excess ethanol at room temperature, and then heated to 50 °C to produce 1. Analogously, CMFCC was prepared and reacted with monohydric alkyl alcohols of increasing chain length (ethanol through 1-octanol) to synthesize the monoesters 1–7 (Table 1). All the monoesters were characterized by FTIR, NMR (1H and 13C), and HRMS.
Table 1 List of monoesters synthesized by the oxidative esterification of CMF through a one-pot, two-step processa
Entry |
Alcohol reagent |
Product structure & code |
Yield (%) |
Reaction conditions: CMF (2.000 g, 13.84 mmol), (CH3)3COCl (4.51 g, 41.50 mmol), RT, 24 h; R1OH (10 mL), 50 °C, 6 h.
|
1 |
Ethanol |
|
83 |
2 |
1-Propanol |
|
82 |
3 |
1-Butanol |
|
85 |
4 |
1-Pentanol |
|
85 |
5 |
1-Hexanol |
|
86 |
6 |
1-Heptanol |
|
89 |
7 |
1-Octanol |
|
89 |
As evident from Table 1, all the alcohols afforded excellent isolated yields of the corresponding monoester. There was a marginal increase in the yield of the monoester when alcohols with longer alkyl chain lengths were used. For example, 1-propanol gave an 83% isolated yield of 2 (entry 2, Table 1), whereas 1-octanol gave an 89% isolated yield of the monoester 7 (entry 7, Table 1). The result may be attributed to the increased nucleophilicity of the alcohol reagent with longer alkyl chain lengths.
The monoesters 1–7 were then reacted with carboxylic acid salt as reagents to synthesize the diesters 1a–7a, 1b–7b, 1c–7c, and 1d–7d (Table 2). The nucleophilic substitution reaction was performed using the triethylammonium salt of various alkyl and aryl carboxylic acids. The alkyl carboxylic acids used in this study are acetic acid, propanoic acid, and levulinic acid, whereas benzoic acid was used as the aryl carboxylic acid. Triethylamine (TEA) was used as the organic base since it is inexpensive, available in bulk quantities, relatively non-toxic, and conveniently recoverable by distillation. The TEA salt of the carboxylic acids was prepared by reacting equimolar amounts of TEA and carboxylic acids at 80 °C. The reaction took roughly around 3 h to complete for all the carboxylic acids studied.23 When 1 was reacted with 1.5 equivalent of pre-formed TEAA at 80 °C under organic solvent-free conditions, the reaction was completed within 3 h. The progress of the reaction was monitored by TLC to observe the disappearance of 1. After the stipulated duration, the crude reaction mixture was quenched with distilled water, and the product was extracted using ethyl acetate. The product 1a was purified from color impurities by simply passing it through a plug of silica gel. An 85% isolated yield of 1a was obtained, and its spectroscopic characterization data matched well with the published literature.31 When 1 was reacted with triethylammonium propionate, the resulting 1b was obtained in nearly 80% yield.32
Table 2 The list of diesters synthesized by the nucleophilic substitution of the chloromethyl group in monoesters using triethylammonium salt of the carboxylic acidsa
Carboxylate reagent |
Product structure, code & yield |
Product structure, code & yield |
Reaction conditions: Et3NHCO2R2 (1.5 equiv.), monoester (1 equiv.), 80 °C, 3 h.
|
|
|
|
|
|
|
|
|
|
|
|
|
|
|
|
|
|
|
|
|
|
|
|
|
|
|
|
|
|
|
|
|
|
|
|
|
When the nucleophilic substitution reaction was attempted at room temperature under neat conditions by employing equimolar amounts of the monoester 1 and TEAA, no observable conversion of the former occurred even after 8 h. The reaction produced only trace amounts of the product after stirring for 24 h at RT. When the amount of TEAA was increased to 1.2 equivalent, the product started forming after 1 h, even though the kinetics remained extremely sluggish. When 1.8 equivalent of TEAA was used, the yield of 1a increased to 20% after 8 h.
It was found that around 1.5 equivalents (with respect to the quantity of monoesters 1–7) of the triethylammonium salt of the alkyl carboxylic acids were required to ensure satisfactory isolated yields of the corresponding diesters. When the reaction was performed at 80 °C using 1–1.2 equivalent of the triethylammonium salt of the alkyl carboxylic acids, the conversion of monoester was not quantitative even after 8 h. With an equivalent amount of the carboxylate reagent, the yield of diester 1a reached only 45% after 24 h at 80 °C, where around 50% of 1 remained unreacted in the reaction mixture. When the equivalent was increased to 1.2 (with respect to 1), the yield improved to 60% under the same reaction parameters. Increasing the equivalent of TEAA to 1.5, the reaction was completed within 3 h at 80 °C, and an 85% isolated yield of 1a was obtained. Increasing the amount of carboxylate to 1.8 equivalent had no significant impact on the reaction kinetics and isolate yield of 1a (Fig. 1).
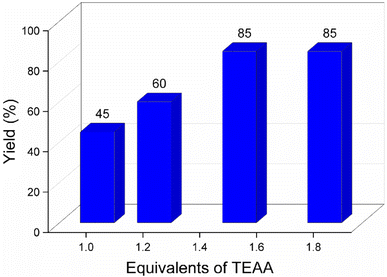 |
| Fig. 1 The effect of TEAA equivalents on the yield of 1a. Reaction conditions: 80 °C, 3 h. | |
Temperatures above 80 °C led to increased decomposition of the triethylammonium salts and lower yields of the diesters. However, lower temperatures (50–70 °C) did not afford good yields of 1a due to incomplete reaction even after 24 h using 1.8 equivalent of TEAA. On the other hand, only 1.2 equivalent of the triethylammonium salt of aryl carboxylic acid was enough to complete the reaction and achieve good isolated yields of the diester under identical reaction conditions (i.e., 80 °C, 3 h). This observation can be explained by the lower thermal stability of the triethylammonium salt of alkyl carboxylic acids. Using an organic solvent slowed down the kinetics but had no positive impact on the yield of the diesters.
The one-pot synthesis of diesters could be adopted as a step-efficient method for synthesis. When triethylammonium carboxylate was added to the reaction mixture in the presence of excess alcohol, only a trace amount of the diester was formed. The reaction kinetics was very slow, and even after 12 h of reaction, no significant change was observed. Moreover, partial transesterification was also observed since alcohol reacted with the alkyl ester. Therefore, the excess alcohol used in the first step had to be removed by evaporation before introducing the TEAA. An organic solvent, acetonitrile added in the reaction mixture for the improvement of product yield. Two equivalents of TEAA are required to complete the reaction due to the in situ generation of hydrochloric acid. The yield of 1a in the one-pot, two-step synthesis was 60%, compared to around 70% in the two-step synthesis.
Green metrics like atom economy (AE), carbon economy (CE), and process mass intensity (PMI) have been calculated to estimate the sustainability of the synthetic route and to find ways to improve it further. The CE is 100% for the mono- and diesters reported in this work since no molecular fragment containing carbon atoms is eliminated during the mechanistic pathway. The AE for synthesizing 1a using the two-step synthesis is 46%. On the other hand, the PMI for the two-step synthesis of 1a was calculated to be 80.5 when the crude product was purified by filtering through a plug of silica gel. For the actual process, when the solvents are recovered and the byproducts like tert-butanol are recycled, the PMI will decrease drastically. When 1c, which is produced from carbohydrate-derived CMF, ethanol, and LA, was taken as an example, the AE was found to be 51.9%. The CE for 1c starting from glucose was calculated to be 86.7%. Carbon atoms of glucose are lost as CO2 during the fermentative preparation of ethanol and also lost as formic acid during LA production.
4. Conclusion
In conclusion, a general synthetic protocol was developed for the high-yielding synthesis of several monoesters and diesters of carbohydrate-derived CMF. The aldehyde group in CMF was selectively oxidized to a carbonyl chloride by using TBHC as the oxidant. The carbonyl chloride was then used as a reactive intermediate to form monoesters by treating with suitable alcohol reagents at slightly elevated temperatures under organic solvent-free conditions. The monoesters were then reacted with the triethylammonium salt of various alkyl- and aryl carboxylic acids to form diesters by a nucleophilic substitution reaction. All the products were isolated in excellent yields under moderate conditions and without using organic solvents as the reaction medium. The alcohol and carboxylic acid reagents are often bio-derived, and therefore, the monoesters and diesters with potential applications as fuel oxygenate and surfactants can be made entirely biorenewable. The esters 1 and 1a were prepared on a 10 g scale to demonstrate the scalability of the reported synthetic protocol. Moreover, n-heptane and ethyl acetate were employed as innocuous organic solvents for isolating and purifying the products. Chromatographic purification was avoided in the second step by using trituration as an alternative purification method. Future research will focus on the recyclability of all reagents used and the scalability of the synthetic steps.
Data availability
The data supporting this article have been included as part of the ESI.† The ESI† includes calculations for the green metrics, spectroscopic (FTIR, 1H-NMR, and 13C-NMR) characterization data, processed spectra, and HRMS data.
Author contributions
Sandeep Kumar Yadav synthesized the compounds, analyzed the spectroscopic data, and edited the manuscript. Saikat Dutta conceptualized the work, supervised the progress, and wrote the original manuscript.
Conflicts of interest
The authors declare no competing interest.
Acknowledgements
The authors thank the Central Research Facility (CRF), NITK, Surathkal, NMR Facility, Mangalore University, and TIFR, Mumbai, for their assistance in collecting the NMR data and School of Advance Sciences, VIT, Vellore and the Central Instrument Facility (CIF), IIT Jammu for collecting the HRMS data. SD thanks DST-SERB for funding under the Core Research Grant (CRG) scheme (file no. CRG/2021/001084 and CRG/2022/009346).
References
- G. Centi and S. Perathoner, Status and Gaps toward Fossil-Free Sustainable Chemical Production, Green Chem., 2022, 24(19), 7305–7331, 10.1039/D2GC01572B.
- L. Wollensack, K. Budzinski and J. Backmann, Defossilization of Pharmaceutical Manufacturing, Curr. Opin. Green Sustainable Chem., 2022, 33, 100586, DOI:10.1016/j.cogsc.2021.100586.
- Y. Zhang, Z. Ding, M. Shahadat Hossain, R. Maurya, Y. Yang, V. Singh, D. Kumar, E.-S. Salama, X. Sun, R. Sindhu, P. Binod, Z. Zhang and M. Kumar Awasthi, Recent Advances in Lignocellulosic and Algal Biomass Pretreatment and Its Biorefinery Approaches for Biochemicals and Bioenergy Conversion, Bioresour. Technol., 2023, 367, 128281, DOI:10.1016/j.biortech.2022.128281.
- R.-J. Van Putten, J. C. Van Der Waal, E. De Jong, C. B. Rasrendra, H. J. Heeres and J. G. De Vries, Hydroxymethylfurfural, a Versatile Platform Chemical Made from Renewable Resources, Chem. Rev., 2013, 113(3), 1499–1597, DOI:10.1021/cr300182k.
- L. Hu, Y. Jiang, Z. Wu, X. Wang, A. He, J. Xu and J. Xu, State-of-the-Art Advances and Perspectives in the Separation of Biomass-Derived 5-Hydroxymethylfurfural, J. Cleaner Prod., 2020, 276, 124219, DOI:10.1016/j.jclepro.2020.124219.
- X. Kong, Y. Zhu, Z. Fang, J. A. Kozinski, I. S. Butler, L. Xu, H. Song and X. Wei, Catalytic Conversion of 5-Hydroxymethylfurfural to Some Value-Added Derivatives, Green Chem., 2018, 20(16), 3657–3682, 10.1039/C8GC00234G.
- C. Rosenfeld, J. Konnerth, W. Sailer-Kronlachner, P. Solt, T. Rosenau and H. W. G. Van Herwijnen, Current Situation of the Challenging Scale-Up Development of Hydroxymethylfurfural Production, ChemSusChem, 2020, 13(14), 3544–3564, DOI:10.1002/cssc.202000581.
- H. N. Anchan and S. Dutta, Recent Advances in the Production and Value Addition of Selected Hydrophobic Analogs of Biomass-Derived 5-(Hydroxymethyl)furfural, Biomass Convers. Biorefin., 2023, 13(4), 2571–2593, DOI:10.1007/s13399-021-01315-1.
- B. J. Moron, F. Arbore, G. P. M. Van Klink, M. Mascal and G. M. Gruter, Industrial Routes from Sugars and Biomass to CMF and Other 5-(Halomethyl)furfurals, ChemSusChem, 2024, e202400495, DOI:10.1002/cssc.202400495.
- M. Mascal and E. B. Nikitin, Dramatic Advancements in the Saccharide to 5-(Chloromethyl)furfural Conversion Reaction, ChemSusChem, 2009, 2(9), 859–861, DOI:10.1002/cssc.200900136.
- S. B. Onkarappa and S. Dutta, Phase Transfer Catalyst Assisted One-Pot Synthesis of 5-(Chloromethyl)furfural from Biomass-Derived Carbohydrates in a Biphasic Batch Reactor, ChemistrySelect, 2019, 4(25), 7502–7506, DOI:10.1002/slct.201901347.
- B. J. Moron, G. Van Klink and G.-J. M. Gruter, Production and Downstream Integration of 5-(Chloromethyl)furfural from Lignocellulose, ACS Sustainable Chem. Eng., 2023, 11(49), 17492–17509, DOI:10.1021/acssuschemeng.3c05525.
- B. A. Smith, P. Champagne and P. G. Jessop, A Semi-Batch Flow System for the Production of 5-Chloromethylfurfural, Chem. Methods, 2021, 1(10), 438–443, DOI:10.1002/cmtd.202100031.
- P. Rojahn, K. D. P. Nigam and F. Schael, Experimental Study and Kinetic Modeling of Continuous Flow Conversion of Fructose to 5-(Chloromethyl)furfural Using Micro- and Millistructured Coiled Flow Inverter, Chem. Eng. J., 2022, 450, 138243, DOI:10.1016/j.cej.2022.138243.
- M. Mascal, 5-(Chloromethyl)furfural is the New HMF: Functionally Equivalent But More Practical in Terms of Its Production from Biomass, ChemSusChem, 2015, 8(20), 3391–3395, DOI:10.1002/cssc.201500940.
- S. Dutta, Valorization of Biomass-Derived Furfurals: Reactivity Patterns, Synthetic Strategies, and Applications, Biomass Convers. Biorefin., 2023, 13(12), 10361–10386, DOI:10.1007/s13399-021-01924-w.
- K. Kumar, S. Pathak, A. K. Rajora, A. Halog and S. Upadhyayula, Kinetic and Thermodynamic Studies of HMF-Ester Synthesis Using Brønsted–Lewis Acidic Ionic Liquid Catalyst, RSC Sustainability, 2023, 1(2), 282–293, 10.1039/D2SU00058J.
- K. Kumar, A. Dahiya, T. Patra and S. Upadhyayula, Upgrading of HMF and Biomass-Derived Acids into HMF Esters Using Bifunctional Ionic Liquid Catalysts under Solvent Free Conditions, ChemistrySelect, 2018, 3(22), 6242–6248, DOI:10.1002/slct.201800903.
- M. S. Tiwari, D. Wagh, J. S. Dicks, J. Keogh, M. Ansaldi, V. V. Ranade and H. G. Manyar, Solvent Free Upgrading of 5-Hydroxymethylfurfural (HMF) with Levulinic Acid to HMF Levulinate Using Tin Exchanged Tungstophosphoric Acid Supported on K-10 Catalyst, ACS Org. Inorg. Au, 2023, 3(1), 27–34, DOI:10.1021/acsorginorgau.2c00027.
- M. Krystof, M. Pérez-Sánchez and P. Domínguez de María, Lipase-Catalyzed (Trans)Esterification of 5-Hydroxy-Methylfurfural and Separation from HMF Esters Using Deep-Eutectic Solvents, ChemSusChem, 2013, 6(4), 630–634, DOI:10.1002/cssc.201200931.
- D. Rigo, D. Polidoro, A. Perosa and M. Selva, Diversified Upgrading of HMF via Acetylation, Aldol Condensation, Carboxymethylation, Vinylation and Reductive Amination Reactions, Mol. Catal., 2021, 514, 111838, DOI:10.1016/j.mcat.2021.111838.
- O. Nilaphai, S. Thepwatee, P. Kaeopookum, L. C. Chuaitammakit, C. Wongchaichon, O. Rodjang, P. Pudsong, W. Singhapon, T. Burerat, S. Kamtaw, S. Chuepeng and S. Kongsriprapan, Synthesis of 5-(Hydroxymethyl)furfural Monoesters and Alcohols as Fuel Additives toward Their Performance and Combustion Characteristics in Compression Ignition Engines, ACS Omega, 2023, 8(19), 17327–17336, DOI:10.1021/acsomega.3c02385.
- N. S. Bhat, S. L. Hegde, S. Dutta and P. Sudarsanam, Efficient Synthesis of 5-(Hydroxymethyl)furfural Esters from Polymeric Carbohydrates Using 5-(Chloromethyl)furfural as a Reactive Intermediate, ACS Sustainable Chem. Eng., 2022, 10(18), 5803–5809, DOI:10.1021/acssuschemeng.1c08571.
- H. Weerathunga, S. Sarina, H.-Y. Zhu and E. R. Waclawik, Oxidative Esterification of 5-Hydroxymethylfurfural into Dimethyl 2,5-Furandicarboxylate Using Gamma Alumina-Supported Gold Nanoparticles, ACS Omega, 2021, 6(7), 4740–4748, DOI:10.1021/acsomega.0c05541.
- C. Moriana Herraiz, K. S. Arias, M. J. Climent, S. Iborra and A. Corma, Chemoenzymatic Synthesis of Amino-Esters as Precursors of Ammonium Salt-Based Surfactants from 5-Hydroxymethylfurfural (HMF), Green Chem., 2024, 26(16), 9118–9131, 10.1039/D4GC00425F.
- K. S. Arias, J. M. Carceller, M. J. Climent, A. Corma and S. Iborra, Chemoenzymatic Synthesis of 5-Hydroxymethylfurfural (HMF)-Derived Plasticizers by Coupling HMF Reduction with Enzymatic Esterification, ChemSusChem, 2020, 13(7), 1864–1875, DOI:10.1002/cssc.201903123.
- N. S. Bhat, A. K. Yadav, M. Karmakar, A. Thakur, S. S. Mal and S. Dutta, Preparation of 5-(Acyloxymethyl)furfurals from Carbohydrates Using Zinc Chloride/Acetic Acid Catalyst System and Their Synthetic Value Addition, ACS Omega, 2023, 8(8), 8119–8124, DOI:10.1021/acsomega.3c00143.
- S. Dutta, L. Wu and M. Mascal, Production of 5-(Chloromethyl)furan-2-carbonyl Chloride and Furan-2,5-dicarbonyl Chloride from Biomass-derived 5-(Chloromethyl)furfural (CMF), Green Chem., 2015, 17(7), 3737–3739, 10.1039/C5GC00936G.
- M. J. Mintz and C. Walling, t-Butyl hypochlorite, Org. Synth., 1969, 49, 9, DOI:10.15227/orgsyn.049.0009.
- J. Kováč and A. J. Kováč, 2,5-Disubstituted furan derivatives. II. Preparation and infrared spectra of esters of 5-chloromethylpyromucic acid, Chem. Pap., 1964, 18(9), 682–687 Search PubMed.
- E. M. Serum, C. A. Sutton, A. C. Renner, D. Dawn and M. P. Sibi, New AB Type Monomers from Lignocellulosic Biomass, Pure Appl. Chem., 2019, 91(3), 389–396, DOI:10.1515/pac-2018-0913.
-
M. E. Janka, Oxidation Process to Produce 5-(Alkoxycarbonyl)furan-2-Carboxylic Acids (ACFC), US Pat., US11655334B2, May 23, 2023, https://patents.google.com/patent/US11655334B2/en Search PubMed.
|
This journal is © The Royal Society of Chemistry 2025 |
Click here to see how this site uses Cookies. View our privacy policy here.