Protein-modified hollow copper sulfide nanoparticles carrying indocyanine green for photothermal and photodynamic therapy†
Received
26th September 2015
, Accepted 17th November 2015
First published on 17th November 2015
Abstract
A novel phototherapy nanoplatform is prepared by coating hollow CuS nanoparticles with a bovine serum albumin–folic acid (BSA–FA) complex. The obtained CuS–BSA–FA nanoparticles are used as drug-delivery vehicles to transport a near-infrared-absorbing phototherapeutic agent (indocyanine green, ICG) into HeLa cells, after loading ICG onto CuS–BSA–FA. In this manner, a combined therapy approach is established consisting of photothermal therapy (by CuS–BSA–FA nanocarriers) and cytotoxic effects of photodynamic and photothermal therapy (by ICG upon 808 nm laser irradiation). The encapsulation of ICG onto CuS–BSA–FA significantly improves the stability and reduces the dark toxicity of free ICG. This therapeutic system exhibits an obviously higher photothermal heating effect and capability of 1O2 generation under laser irradiation compared with bare nanocarriers. In addition, the grafted FA segments on the surface of CuS–BSA–FA are proved to enhance the internalization of nanoparticles by FA-receptor-mediated endocytosis.
1. Introduction
Phototherapy induced by near-infrared (NIR) irradiation is capable of selectively killing cancer cells. In comparison with traditional hyperthermia ablation, this inside-out heating approach rules out the possible damage to normal tissues in the dark. This makes the phototherapy a promising alternative or supplement to traditional cancer therapies.1–3 Some inorganic nanomaterials, e.g., gold nanostructures,4 carbon materials,5,6 palladium nanosheets,7 copper chalcogenide semiconductors8,9 and MoS2 nanosheets,10 have the capability to convert the absorbed NIR optical energy into heat, and thus lead to the thermal ablation of cancer cells. This has been extensively investigated for NIR photothermal therapy (PTT). Organic compounds such as indocyanine green (ICG),11 polyaniline nanoparticles12 and porphysomes13 have also been widely exploited as PTT agents in vitro and in vivo. In parallel, photodynamic therapy (PDT) provides further potential for killing cancer cells by cytotoxic singlet oxygen (1O2) or reactive oxygen species (ROS) generated from photosensitizer molecules under illumination and in the presence of oxygen.14 In addition, some organic PSs such as porphyrin and phthalocyanines,15 as well as metal nanoparticles (Au, Ag and Pt),16 TiO217 and quantum dots,14,18 have also been demonstrated to possess PDT capability.
ICG is an ideal NIR light absorber for laser-mediated PTT and PDT, and it is currently used for diagnostic applications, e.g., blood volume determination, fluorescence angiography, cardiac output and hepatic function.15,19–21 However, ICG is unstable in aqueous medium and tends to be eliminated rapidly from the living body. To overcome the high degradation rate and the short plasma half-life, various nanocarriers such as mesoporous silica, calcium phosphate and lipid–polymer nanoparticles have been investigated to encapsulate ICG.22–24
Semiconductor CuS nanoparticles are a new class of promising photothermal agents attributing to their low cost, high photo-stability and low cytotoxicity. The d–d energy band transition of Cu2+ ions endows them with NIR light absorption.25,26 Among the wide range of nanostructures, hollow CuS nanostructures obtained by a template-assisted method exhibit a large specific surface area, numerous mesoporous pores, and good biocompatibility and their elimination from the living body is readily feasible. These features make them favorable drug-delivery vehicles to transport therapeutic agents into cancer cells.27–29 Thus, hollow CuS nanoparticles exhibit great potential in the application as both photothermal agents and drug-delivery carriers.
In the present work, hollow CuS nanoparticles are functionalized with BSA–FA complexes to improve the stealthiness of the nanomedicine in biological systems and the capability to target cancer cells. The CuS–BSA–FA nanoparticles are then applied as nanocarriers for ICG, to trigger simultaneous production and delivery of heat and singlet oxygen species to cancer cells. On account of the combined effects of NIR photothermal and photodynamic therapy, the delivery system could achieve enhanced anticancer efficacy.
2. Experimental
2.1. Materials
(NH4)2S aqueous solution (20 wt%), folic acid, fluorescein isothiocyanate (FITC), N-hydroxysuccinimide (NHS), N-[3-(dimethylamino)propyl]-N′-ethylcarbodiimide hydrochloride (EDC), folic acid (FA), polyethylenimine (PEI), polyvinylpyrrolidone (PVP K30) and 1,3-diphenylisobenzofuran (DPBF) are purchased from Aladdin Reagent (Shanghai, China). Bovine serum albumin (BSA) and indocyanine green (ICG) are purchased from Sigma (St. Louis, MO, USA). DMEM (high glucose), penicillin/streptomycin, trypsin and fetal bovine serum (FBS) are obtained from Thermo Scientific (Logan, Utah, USA). The 3-(4,5-dimethylthiazol-2-yl)-2,5-diphenyltetrazolium bromide (MTT) assay kit is received from Nanjing KeyGEN Biotech (Nanjing, China). Other chemicals used in this study are purchased from Sinopharm Chemical Reagent (Shenyang, China). Deionized water of 18 MΩ cm−1 is used throughout the experiments.
2.2. Characterization
UV-vis absorption spectra are recorded on a UH5300 UV-vis spectrophotometer (Hitachi, Japan) at room temperature. Transmission electron microscopy (TEM) images are obtained by using a Tecnai G2 20 microscope (Philips, Holland). SEM images are taken on a ZEISS Ultra/Plus scanning electron microscope (ZEISS, Germany). X-ray diffraction (XRD) patterns are acquired on a D8 Advance diffractometer (Bruker, Germany) using CuKα radiation (λ = 1.54 Å). X-ray photoelectron spectroscopy (XPS) analysis is carried out on an ESCALAB 250 X-ray photoelectron spectrometer (Thermo Ltd, USA) to measure the composition of hollow CuS nanoparticles. Dynamic light scattering (DLS) and zeta potential measurements are performed using a Zetasizer Nano ZS/ZEN3690 instrument (Malvern, England). The copper content is determined by inductively coupling plasma mass spectrometry (ICP-MS, Agilent 7500a, USA). The photothermal properties of the materials are assessed by irradiating with a diode infrared laser (MDL-III-808 nm-2.5 W-14100192, Changchun New Industries Optoelectronics Tech. Co. Ltd, China).
2.3. Preparation of the hollow CuS nanoparticles
Hollow CuS nanospheres are prepared according to a previously reported method.27 Briefly, CuCl2·2H2O (0.1 mmol) is added to 60 mL of 2-propanol containing PVP K30 (0.2 g) in a round flask under magnetic stirring. A NaOH solution (1 mmol) is added to the mixture, followed by the addition of N2H4·H2O (35 wt%, 0.4 mL). After stirring for 10 min, the obtained Cu2O nanospheres are collected by centrifugation, washed with deionized water, and then re-dispersed in water. (NH4)2S aqueous solution (20 wt%, 35 μL) is then added to the Cu2O nanosphere suspension and stirred for 2 h at room temperature. Finally, the produced hollow CuS nanoparticles are centrifuged and re-suspended in 20 mL of deionized water for further use.
2.4. Preparation of the CuS–BSA–FA composites
Hollow CuS nanoparticles are firstly modified with PEI by mixing the nanoparticles (20 mL) with PEI aqueous solution (4 mg mL−1, 20 mL) for 4 h at room temperature. The PEI-modified CuS nanoparticles are centrifuged and re-dispersed in 40 mL of deionized water. FA is covalently conjugated to BSA following a literature approach:15 1 mL of FA (3 mg mL−1) in 2-(N-morpholino)ethanesulfonic acid (MES) buffer solution (50 mM, pH 6.0) is mixed with 3 mg of EDC and 3 mg of NHS, and reacts for 20 min. 0.5 mL of the above solution is mixed with 100 mL of BSA solution (0.4 mg mL−1, MES) for 12 h, and then the product is dialyzed for 24 h against MES buffer solution with a cellulose membrane (MWCO 5000 Da). The BSA–FA solution (20 mL) is then mixed with the aqueous solution of PEI-coated CuS, followed by the addition of 24 mg of EDC and 24 mg of NHS. After stirring for 12 h, the CuS–BSA–FA nanoconjugates are centrifuged for 15 min at 5000 rpm to eliminate the unadsorbed BSA–FA. This process is repeated three times and the product is re-suspended in PBS (pH 7.4) buffer solution.
2.5. ICG loading onto CuS–BSA–FA and its subsequent release
For the loading of ICG, different volumes (10–100 μL) of ICG solution (4.5 mg mL−1, DMSO) are mixed with 646 μg of CuS–BSA–FA in PBS buffer (pH 7.4). The mixture is diluted with PBS buffer to 1.0 mL and then shaken for 4 h at room temperature. The resulting CuS–BSA–FA/ICG is collected by centrifugation, and washed with PBS twice to remove the unadsorbed ICG. To evaluate the loading ratio of ICG, the supernatant and the washing solution are collected and the concentration of ICG is determined by measuring the absorbance at 780 nm. The mass of unadsorbed ICG (muICG) is achieved by multiplying the concentration of ICG by the volume of the above solution. The loading ratio of ICG to CuS–BSA–FA is expressed as:
Loading ratio = (mICG − muICG)/mCuS–BSA–FA × 100% |
where mICG and mCuS–BSA–FA are the mass of ICG and CuS–BSA–FA, respectively.
The release behavior of ICG is evaluated at 37 °C in PBS buffer (pH 7.4, 6.3, and 5.2). 2 mL of CuS–BSA–FA/ICG (ICG content: 80 μg) is placed in a centrifuge tube and maintained in a 37 °C bath with constant shaking. For every 24 h, 0.5 mL of the mixture is taken and centrifuged. The released ICG in the supernatant is quantified by spectrophotometry at 780 nm. The CuS–BSA–FA/ICG sediment is re-suspended in 0.5 mL of fresh buffer and then mixed with the rest 1.5 mL of CuS–BSA–FA/ICG in PBS buffer for further releasing tests. The release of ICG in DMEM and DMEM supplemented with 10% fetal bovine serum is performed in the same way.
2.6. NIR-laser induced temperature increase and singlet oxygen generation
A continuous wave fiber-coupled diode laser source with an 808 nm wavelength is used to perform the temperature increment tests. PBS buffer, CuS–BSA–FA, free ICG and CuS–BSA–FA/ICG are placed in the centrifuge tubes and irradiated for 10 min individually, and the temperature is recorded using a thermometer (IKA Ltd).
The generation of 1O2 is detected by using DPBF as a 1O2 sensor. 2 mL of CuS–BSA–FA (60 μg mL−1), ICG (1 μg mL−1) and CuS–BSA–FA/ICG (60 μg mL−1 for CuS–BSA–FA and 1 μg mL−1 for ICG) in DMSO are mixed with 20 μL of DPBF (8 mM, DMSO), respectively. After stirring and irradiating using an NIR laser (1 W cm−2) for 10 min, the UV-vis absorption spectra of these mixtures are recorded.
2.7.
In vitro phototherapy and cell imaging
HeLa and A549 cell lines are used for in vitro studies. Cells are grown in DMEM supplemented with 10% fetal bovine serum and 1% penicillin/streptomycin in standard culture (37 °C, 5% CO2).
For in vitro combined PDT/PTT experiments, HeLa cells are seeded in a 24-well plate at a density of 5 × 104 cells per well and incubated with various concentrations of CuS–BSA–FA, free ICG and CuS–BSA–FA/ICG, and irradiated by an NIR laser at 1 W cm−2 for 5 min. Dead cells are stained by 0.4% trypan blue and observed using a fluorescence microscope. The mixture is incubated at 37 °C for additional 24 h to implement the MTT assay. After treatment, 20 μL of MTT solution is added to each well of the plate and incubated for 4 h. The media are removed, and 500 μL of DMSO is then added to dissolve the formazan crystals. The absorbance of each well is measured at 550 nm by using a microplate reader.
For the targeted cell imaging, a fluorescent molecule FITC is loaded into CuS–BSA–FA and CuS–BSA, respectively. The folate-receptor-positive HeLa cells are then incubated with 120 μg mL−1 of FITC-loaded CuS–BSA–FA and CuS–BSA for 6 h. The folate-receptor-negative A549 cells are used as control cells. After washing with PBS, these cells are observed using a fluorescence microscope (0.4% trypan blue is added to quench the fluorescence signals outside the cells).
3. Results and discussion
3.1. Preparation and characterization of the CuS–BSA–FA composites
Scheme 1 illustrates the strategy for the construction of the hybrid nanocarriers CuS–BSA–FA. The hollow CuS nanoparticles are prepared by a template-assisted approach followed by modification with PEI to introduce amino groups on their surface. Afterwards, the nanoparticles are functionalized with BSA–FA complexes through the formation of amide bonds to acquire full stealthiness under physiological conditions.15,30,31
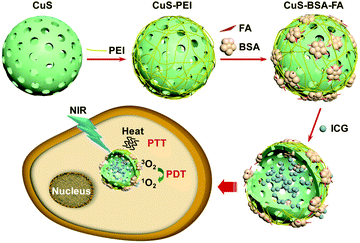 |
| Scheme 1 The schematic illustration of the construction of CuS–BSA–FA as a vehicle to deliver ICG and the mechanism of PDT and PTT therapies based on this system. | |
The TEM and SEM images reveal that the CuS nanoparticles are around 120 nm in diameter with hollow interiors and porous shells of ca. 20 nm in thickness as illustrated in Fig. 1A and D, which is further verified by the particle size distribution of CuS measured by DLS (Fig. S1, ESI†). The process of functionalization with PEI and BSA–FA barely affects the structure of these nanoparticles (Fig. 1B and C). The Brunauer–Emmett–Teller (BET) surface area and the pore volume are derived to be 16.8 m2 g−1 and 0.16 cm3 g−1, respectively (Fig. S2, ESI†). Nitrogen adsorption–desorption isotherms and pore size distribution of the hollow CuS indicate the presence of mesopores and macropores in the shell and core of the hollow CuS, which is consistent with the observations in TEM images.
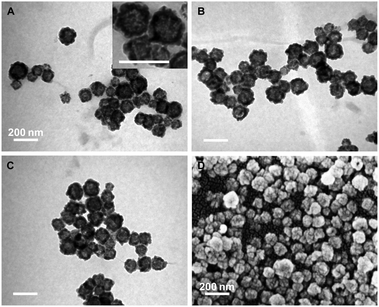 |
| Fig. 1 TEM images of CuS (A), CuS–PEI (B) and CuS–BSA–FA (C). Inset in (A): magnified TEM image of CuS; scale bar: 200 nm. SEM image of the hollow CuS nanoparticles (D). | |
XRD patterns indicate that all the diffraction peaks of hollow CuS nanoparticles are consistent with the standard data of covellite-phase CuS (PDF card No.: 00-001-1281). The diffraction peaks located at 2θ of 29.49, 31.98, 33.06, 48.14 and 59.55 are, respectively, attributed to (102), (103), (006), (110) and (116) planes (Fig. 2A). The high-resolution XPS spectra of Cu and S in the 2p region for hollow CuS nanoparticles (Fig. 2B and C) indicate the binding energies of Cu 2p3/2 and Cu 2p1/2 at 931.9 and 951.8 eV, respectively. These are typical values for Cu2+ in CuS. Meanwhile the binding energies of S 2p3/2 and S 2p1/2 are 161.4 and 162.6 eV, respectively. This indicates the presence of S2− in the product.32–34 The chemical composition of the as-synthesized hollow CuS is evaluated by ICP-MS, and an approximate Cu/S ratio of 1
:
1 is achieved. Furthermore, the hollow CuS nanoparticles are confirmed to display intensive optical absorbance in the NIR region (700–900 nm) by UV-vis-NIR spectroscopy (Fig. 2D).
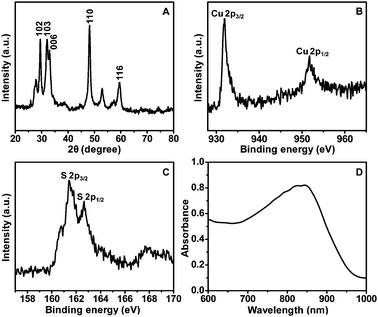 |
| Fig. 2 XRD pattern (A), XPS spectra (B and C), and UV-vis-NIR absorption spectrum (D) of the hollow CuS nanoparticles. | |
To verify the grafting of BSA–FA on the surface of hollow CuS nanoparticles, the variation of surface charges of the nanoparticles during the modification process is investigated by zeta potential measurements (Fig. 3A). The initial CuS nanoparticles exhibit a zeta potential of −15.0 mV, while it changes to +13.5 mV by coating with PEI. After BSA–FA grafting, the zeta potential of the obtained CuS–BSA–FA is significantly dropped to −2.5 mV. Meanwhile, virtually no change is observed for the absorption spectra during the modification process with PEI and BSA–FA complexes (Fig. 3B). The concentration of BSA is determined by staining with Coomassie brilliant blue G250, and a CuS/BSA mass ratio of (14.2 ± 0.8)
:
1 is obtained. The experiments showed that CuS–BSA–FA nanoparticles are well dispersed in water, PBS and cell medium without any noticeable agglomeration after one-week storage. This indicates the favourable stability of these nanoparticles (Fig. S3, ESI†), which is important for their biomedical applications.
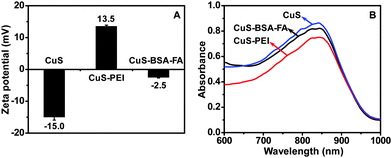 |
| Fig. 3 Zeta potential (A) and UV-vis-NIR absorption spectra (B) of CuS, CuS–PEI and CuS–BSA–FA aqueous solution (240 μg mL−1). | |
3.2.
In vitro loading and release of ICG
It has been previously reported that serum albumin serves as an excellent nanocarrier for drug delivery,35–37 and it is capable of binding ICG via hydrophobic interaction between the ICG molecule and the hydrophobic domain of the protein.38 Considering the hollow structure of CuS–BSA–FA and the presence of BSA ligands on its surface, the CuS–BSA–FA nanoparticles are promising vehicles for the delivery of ICG. As indicated in Fig. 4A, the drug loading ratio, i.e., the mass ratio of the loaded ICG to CuS–BSA–FA, increases with the increase of the loaded amount of ICG and finally reaches a maximum at a drug concentration of above 400 μg mL−1. This observation indicates that it is feasible to control the loading level of ICG. The maximum loading ratio of ICG is derived to be 54.8% (m/m).
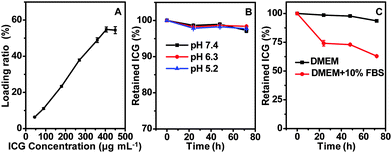 |
| Fig. 4 The dependence of the loading ratio on the drug concentration of ICG (A). The release profiles of ICG from CuS–BSA–FA in PBS buffer (B) and cell medium (C) at 37 °C. | |
The cumulative release profiles of ICG from CuS–BSA–FA/ICG in PBS buffer (pH 7.4, 6.3 and 5.2) at 37 °C are presented in Fig. 4B. It is seen that after 72 h of incubation in PBS buffer, only a very small amount of loaded ICG, i.e., <3%, is released. This illustrated that the release behaviour is barely affected by pH. Then, cumulative release profiles in both DMEM and DMEM supplemented with 10% fetal bovine serum are conducted. Fig. 4C shows no significant release of ICG from CuS–BSA–FA/ICG after incubation in DMEM. However, the release is accelerated in the presence of fetal bovine serum, probably due to the hydrophobic interaction between ICG and proteins in the serum. Most of the drug, i.e., >60%, retains in the carriers even over 72 h, making it possible to transport the phototherapeutic agent to the lesion site.
3.3. Stability of ICG in aqueous medium
UV-vis-NIR absorption spectra of CuS–BSA–FA/ICG and free ICG are recorded (Fig. 5A). Within 600–900 nm, the absorbance of CuS–BSA–FA/ICG is much higher than that of CuS–BSA–FA itself. This observation implies that the photothermal effect of the therapuetic system could be enhanced by the loading of ICG. It is seen that the broad peak of CuS–BSA–FA/ICG exhibits an obvious red-shift with respect to that of the free ICG, indicating that the local environment is different within the nanocarriers as compared to the free ICG.19 Considering that ICG has unstable optical properties and it tends to quickly degradate in aqueous medium, the stability of free ICG and the loaded ICG, i.e., that part of ICG on CuS–BSA–FA/ICG, is further investigated and compared. It is obvious that the absorbance at 780 nm for free ICG significantly dropped to 6% of its original value, while that for the loaded ICG maintains 70% of its original absorbance within the same period of 12 days (Fig. 5B). The free and loaded ICG are then exposed to a xenon light source for irradiation for various time periods. It is seen that the absorbance of free ICG rapidly declined to 70% after 3 h (Fig. 5C), meanwhile, the absorbance remains virtually unchanged for the loaded ICG. These results indicate that the encapsulation of ICG by the CuS–BSA–FA nanocarriers endows ICG with a superior stability.
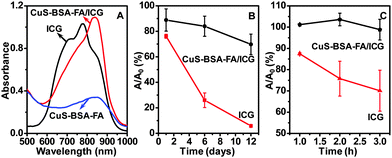 |
| Fig. 5 UV-vis-NIR absorption spectra of free ICG, CuS–BSA–FA and CuS–BSA–FA/ICG (A). The time-dependent variation of absorbance for free ICG and CuS–BSA–FA/ICG at 37 °C (B) and under continuous illumination with a xenon light source (C) (A and A0 represent the absorbance recorded at a given time, and the initial absorbance at 780 nm, respectively). Free ICG: 8 μg mL−1; CuS–BSA–FA/ICG: 120 μg mL−1 for CuS–BSA–FA and 8 μg mL−1 for ICG. | |
3.4. Photothermal effect and singlet oxygen generation
For the purpose of performing photothermal therapy by using the nanoparticles, the temperature increase generated by NIR laser irradiation is among the most important parameters. The photothermal effect of CuS–BSA–FA exhibits an obvious irradiation energy-dependent and concentration-dependent manner (Fig. 6A and B). Meanwhile, after 5 min of irradiation, the temperature increase is ca. 27 °C for CuS–BSA–FA/ICG, ca. 13 °C for CuS–BSA–FA and 19 °C for free ICG molecules (Fig. 6C). This result confirms that the photothermal heating effect of this therapuetic system is significantly improved by the combination of loaded ICG and CuS–BSA–FA nanocarriers. Therefore, it is expected that CuS–BSA–FA/ICG would significantly enhance the PTT effects on cancer cells attributing to the high photothermal energy conversion efficiency.
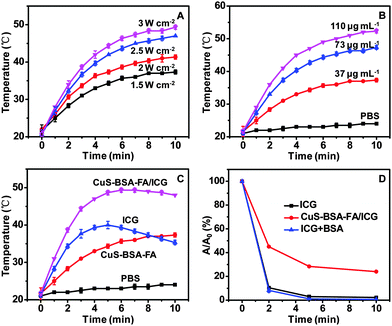 |
| Fig. 6 The photothermal heating curves of CuS–BSA–FA aqueous solution (A) at various irradiation energies (37 μg mL−1 for CuS–BSA–FA); (B) with different concentrations of CuS–BSA–FA (1.5 W cm−2). (C) Photothermal heating curves of CuS–BSA–FA (37 μg mL−1), ICG (21 μg mL−1) and CuS–BSA–FA/ICG (21 μg mL−1 for ICG and 37 μg mL−1 for CuS–BSA–FA) at an irradiation energy level of 1.5 W cm−2. (D) The time-dependent variation of absorbance for DPBF after illumination at 1 W cm−2. ICG: 1 μg mL−1; CuS–BSA–FA/ICG: 60 μg mL−1 for CuS–BSA–FA and 1 μg mL−1 for ICG; BSA: 100 μg mL−1. | |
The possibility of using the CuS–BSA–FA/ICG nanocarriers as potential PDT agents is investigated by measuring their 1O2 generation capability under laser irradiation, with 1,3-diphenylisobenzofuran (DPBF) as a 1O2 sensor.39,40 It is seen that when exposing CuS–BSA–FA/ICG to NIR laser irradiation, a significant decrease in the absorbance of DPBF at 420 nm is observed, which indicates the generation of 1O2. While no 1O2 production is found when bare CuS–BSA–FA is exposed to laser irradiation under the same conditions (Fig. S4, ESI†). In contrast, neither CuS–BSA–FA/ICG nor CuS–BSA–FA shows obvious bleaching of the absorption at 420 nm without laser irradiation. These results well confirm that the introduction of ICG enables CuS–BSA–FA/ICG to produce 1O2 under laser irradiation. Furthermore, the generation of 1O2 is quantitatively determined by following the absorbance intensity variation of DPBF at 420 nm. As illustrated in Fig. 6D, the 1O2 production of CuS–BSA–FA/ICG is lower than that of free ICG at similar concentration. For CuS–BSA–FA/ICG, the laser energy is simultaneously absorbed by the loaded ICG and CuS to produce 1O2 and heat, resulting in a loss of effective energy for the production of 1O2.41,42 Additionally, the result rules out the possible consumption of the singlet oxygen caused by BSA coating on the particle surface.
The above elucidations prove that CuS–BSA–FA/ICG has the potential for simultaneous PTT and PDT treatment activated by NIR light illumination, rather than a single mode of phototherapy or activated by visible light.43–45
3.5. Cell toxicity and in vitro phototherapy
A good photosensitizer should cause no damage to the normal cells in the dark until it reaches the target and starts to kill the cancer cells under NIR laser irradiation. The cell toxicity of ICG, CuS–BSA–FA, and CuS–BSA–FA/ICG in the dark is firstly investigated at three concentration levels of CuS and ICG via the MTT assay in HeLa cells. As depicted in Fig. 7A, the cellular viability is estimated to be higher than 70% after 24 h of incubation in the presence of 120 μg mL−1 of CuS–BSA–FA. The free ICG exhibits a much higher toxicity with 38% of cellular viability at an ICG concentration of 4 μg mL−1. As a comparison, 70% of cells are alive by incubating with CuS–BSA–FA/ICG at the same concentration of ICG. These results indicate that the loading of ICG on CuS–BSA–FA significantly reduces the dark toxicity of ICG to cells. The lower cytotoxicity of CuS–BSA–FA/ICG with respect to free ICG can be explained by the minimal leakage of ICG from CuS–BSA–FA.
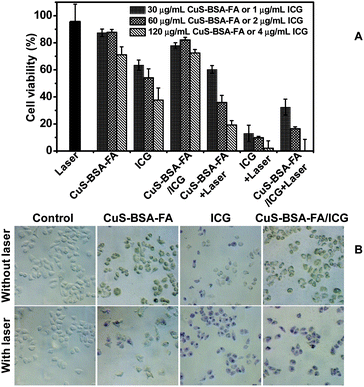 |
| Fig. 7 (A) Cytotoxicity assays of HeLa cells in the presence of CuS–BSA–FA, ICG and CuS–BSA–FA/ICG with or without NIR irradiation at an irradiation energy level of 1 W cm−2 for 5 min. (B) The images of HeLa cells in the presence of CuS–BSA–FA (60 μg mL−1), ICG (2 μg mL−1) and CuS–BSA–FA/ICG (60 μg mL−1 for CuS–BSA–FA and 2 μg mL−1 for ICG) with and without laser irradiation (1 W cm−2). | |
To compare the phototherapy efficiency of ICG, CuS–BSA–FA, and CuS–BSA–FA/ICG, the in vitro cytotoxicity test under NIR laser irradiation is further undertaken at the same concentration of CuS–BSA–FA and ICG. CuS–BSA–FA/ICG exhibits much more improved capability against cancer cells with respect to CuS–BSA–FA (see Fig. 7A). This confirms that the loaded ICG significantly improves phototherapy effects. Although the phototherapy efficiency of CuS–BSA–FA/ICG is less than that of ICG, CuS–BSA–FA/ICG is still preferable with the improved stability and minimized cell toxicity in the dark with respect to that for free ICG.
Cell staining is further conducted to identify the cell viability, and dead cells are stained by trypan blue (Fig. 7B). No obvious cell death is observed when treating the cells with CuS–BSA–FA and CuS–BSA–FA/ICG (60 μg mL−1 for CuS–BSA–FA), and the uptake of CuS–BSA–FA results in a green color for the cells. After incubation with 2 μg mL−1 of ICG, a part of the cells are killed and stained with a blue color. When the cells are exposed to NIR irradiation, they are all destroyed after being incubated with CuS–BSA–FA/ICG, while a part of the cells remain alive by treating with CuS–BSA–FA. This observation further illustrated that a synergistic phototherapy effect is achieved in the CuS–BSA–FA/ICG delivery system.
3.6. Cellular uptake of CuS–BSA–FA
It is known that folic acid has a strong affinity for the folate receptors on the cell membrane, and thus nanocarriers are frequently modified on the surface with folic acid to improve their drug delivery capability to cancer cells rich in folate receptors.46,47 Therefore, the folic acid on the surface of CuS–BSA–FA is supposed to improve the total accumulation of CuS–BSA–FA in the target cells. FITC-loaded CuS–BSA–FA and CuS–BSA are used to investigate the targeting effect toward HeLa cells. As shown in Fig. 8, although the cellular uptake of both nanoparticles is observed in HeLa cells, the cells incubated with CuS–BSA–FA/FITC show a much stronger fluorescence intensity than the cells incubated with CuS–BSA/FITC. To further confirm the receptor-mediated endocytosis mechanism of CuS–BSA–FA, HeLa cells are pre-treated with free FA and then incubated with CuS–BSA–FA/FITC under the same conditions. As a result, the fluorescence intensity of the FA-pre-incubated cells decreases significantly, demonstrating that the specific uptake of CuS–BSA–FA/FITC can be blocked by free FA. In parallel, the folate-receptor-negative A549 cells are incubated with CuS–BSA–FA/FITC for comparison. The weaker fluorescence intensity is detected in A549 cells with respect to HeLa cells. These results confirm that CuS–BSA–FA/FITC can be internalized more efficiently by receptor-mediated endocytosis, and the significant selectivity of CuS–BSA–FA towards cancer cells with overexpressed FA receptors is vital for the delivery of therapeutic reagents and diminishing the side effect of the drugs.
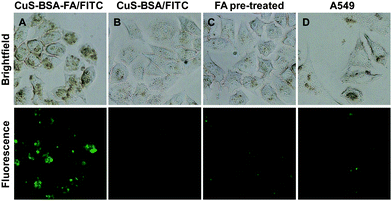 |
| Fig. 8 Fluorescence microscopy images that show the uptake of CuS–BSA–FA/FITC (A) and CuS–BSA/FITC (B) into HeLa cells, CuS–BSA–FA/FITC (C) into free FA pre-treated HeLa cells, and CuS–BSA–FA/FITC (D) into the A549 cells (6 h, 120 μg mL−1). | |
4. Conclusions
A therapeutic system composing of BSA–FA modified hollow CuS nanoparticles and the phototherapeutic agent (ICG) is constructed. The hollow CuS nanoparticles act as excellent photothermal agents and nanocarriers for drug delivery at the same time. The modifier BSA–FA complex offers the stealthiness for the CuS–BSA–FA nanoparticle in the biological system and the capability to target HeLa cells. By encapsulating ICG with CuS–BSA–FA, the CuS–BSA–FA/ICG nanomedicine exhibits superior stability and low cytotoxicity in the dark in comparison with free ICG. In terms of phototherapy efficiency, CuS–BSA–FA/ICG possesses higher cytotoxicity than CuS–BSA–FA under NIR laser irradiation attributing to the efficiently combined PTT and PDT. This simple therapeutic system could be a promising candidate as a phototherapeutic agent for cancer treatment.
Acknowledgements
The authors appreciate financial support from the Natural Science Foundation of China (21575020, 21235001 and 21475017), and Fundamental Research Funds for the Central Universities (N140505003 and N141008001).
References
- Z. J. Zhang, J. Wang and C. Y. Chen, Adv. Mater., 2013, 25, 3869 CrossRef CAS PubMed
.
- L. Cheng, C. Wang, L. Z. Feng, K. Yang and Z. Liu, Chem. Rev., 2014, 114, 10869 CrossRef CAS PubMed
.
- K. Yang, H. Xu, L. Cheng, C. Y. Sun, J. Wang and Z. Liu, Adv. Mater., 2012, 24, 5586 CrossRef CAS PubMed
.
- R. Huschka, J. Zuloaga, M. W. Knight, L. V. Brown, P. Nordlander and N. J. Halas, J. Am. Chem. Soc., 2011, 133, 12247 CrossRef CAS PubMed
.
- H. S. Jung, W. H. Kong, D. K. Sung, M. Y. Lee, S. E. Beack, D. H. Keum, K. S. Kim, S. H. Yun and S. K. Hahn, ACS Nano, 2014, 8, 260 CrossRef CAS PubMed
.
- Y. Wang, K. Y. Wang, R. Zhang, X. G. Liu, X. Y. Yan, J. X. Wang, E. Wagner and R. Q. Huang, ACS Nano, 2014, 8, 7870 CrossRef CAS PubMed
.
- X. Huang, S. Tang, X. Mu, Y. Dai, G. Chen, Z. Zhou, F. Ruan, Z. Yang and N. Zheng, Nat. Nanotechnol., 2011, 6, 28 CrossRef CAS PubMed
.
- M. Zhou, R. Zhang, M. Huang, W. Lu, S. L. Song, M. P. Melancon, M. Tian, D. Liang and C. Li, J. Am. Chem. Soc., 2010, 132, 15351 CrossRef CAS PubMed
.
- Q. W. Tian, F. R. Jiang, R. J. Zou, Q. Liu, Z. G. Chen, M. F. Zhu, S. P. Yang, J. L. Wang, J. H. Wang and J. Q. Hu, ACS Nano, 2011, 5, 9761 CrossRef CAS PubMed
.
- S. S. Chou, B. Kaehr, J. Kim, B. M. Foley, M. De, P. E. Hopkins, J. X. Huang, C. J. Brinker and V. P. Dravid, Angew. Chem., Int. Ed., 2013, 52, 4160 CrossRef CAS PubMed
.
- W. R. Chen, R. L. Adams, A. K. Higgins, K. E. Bartels and R. E. Nordquist, Cancer Lett., 1996, 98, 169 CrossRef CAS PubMed
.
- J. Yang, J. Choi, D. Bang, E. Kim, E. K. Lim, H. Park, J. S. Suh, K. Lee, K. H. Yoo, E. K. Kim, Y. M. Huh and S. Haam, Angew. Chem., Int. Ed., 2011, 50, 441 CrossRef CAS PubMed
.
- J. F. Lovell, C. S. Jin, E. Huynh, H. Jin, C. Kim, J. L. Rubinstein, W. C. W. Chan, W. Cao, L. V. Wang and G. Zheng, Nat. Mater., 2011, 10, 324 CrossRef CAS PubMed
.
- J. C. Ge, M. H. Lan, B. J. Zhou, W. M. Liu, L. Guo, H. Wang, Q. Y. Jia, G. L. Niu, X. Huang, H. Y. Zhou, X. M. Meng, P. F. Wang, C. S. Lee, W. J. Zhang and X. D. Han, Nat. Commun., 2014, 5, 4596 CAS
.
- A. Topete, M. Alatorre-Meda, P. Iglesias, E. M. Villar-Alvarez, S. Barbosa, J. A. Costoya, P. Taboada and V. Mosquera, ACS Nano, 2014, 8, 2725 CrossRef CAS PubMed
.
- R. Vankayala, A. Sagadevan, P. Vijayaraghavan, C. L. Kuo and K. C. Hwang, Angew. Chem., Int. Ed., 2011, 50, 10640 CrossRef CAS PubMed
.
- L. Zeng, W. Z. Ren, L. C. Xiang, J. J. Zheng, B. Chen and A. G. Wu, Nanoscale, 2013, 5, 2107 RSC
.
- A. C. S. Samia, X. B. Chen and C. Burda, J. Am. Chem. Soc., 2003, 125, 15736 CrossRef CAS PubMed
.
- J. Yu, D. Javier, M. A. Yaseen, N. Nitin, R. Richards-Kortum, B. Anvari and M. S. Wong, J. Am. Chem. Soc., 2010, 132, 1929–1938 CrossRef CAS PubMed
.
- X. H. Zheng, F. F. Zhou, B. Y. Wu, W. R. Chen and D. Xing, Mol. Pharmaceutics, 2012, 9, 514 CrossRef CAS PubMed
.
- A. K. Kirchherr, A. Briel and K. Mäder, Mol. Pharmaceutics, 2009, 6, 480 CrossRef CAS PubMed
.
- P. F. Zhao, M. B. Zheng, C. X. Yue, Z. Y. Luo, P. Gong, G. H. Gao, Z. H. Sheng, C. F. Zheng and L. T. Cai, Biomaterials, 2014, 35, 6037 CrossRef CAS PubMed
.
- J. S. Souris, C. H. Lee, S. H. Cheng, C. T. Chen, C. S. Yang, J. A. Ho, C. Y. Mou and L. W. Lo, Biomaterials, 2010, 31, 5564 CrossRef CAS PubMed
.
- E. I. Altınoglu, T. J. Russin, J. M. Kaiser, B. M. Barth, P. C. Eklund, M. Kester and J. H. Adair, ACS Nano, 2008, 2, 2075–2084 CrossRef PubMed
.
- S. Ramadan, L. R. Guo, Y. J. Li, B. F. Yan and W. Lu, Small, 2012, 8, 3143 CrossRef CAS PubMed
.
- Y. B. Li, W. Lu, Q. A. Huang, M. A. Huang, C. Li and W. Chen, Nanomedicine, 2010, 5, 1161 CrossRef CAS PubMed
.
- K. Dong, Z. Liu, Z. H. Li, J. S. Ren and X. G. Qu, Adv. Mater., 2013, 25, 4452 CrossRef CAS PubMed
.
- L. G. Guo, D. D. Yan, D. F. Yang, Y. J. Li, X. D. Wang, O. Zalewski, B. F. Yan and W. Lu, ACS Nano, 2014, 8, 5670 CrossRef CAS PubMed
.
- L. G. Guo, I. Panderi, D. D. Yan, K. Szulak, Y. J. Li, Y. T. Chen, H. Ma, D. B. Niesen, N. Seeram, A. Ahmed, B. F. Yan, D. Pantazatos and W. Lu, ACS Nano, 2013, 7, 8780 CrossRef CAS PubMed
.
- M. S. Strozyk, M. Chanana, I. Pastoriza-Santos, J. Pérez-Juste and L. M. Liz-Marzán, Adv. Funct. Mater., 2012, 22, 1436 CrossRef CAS
.
- J. S. Choi, J. C. Park, H. Nah, S. Woo, J. Oh, K. M. Kim, G. J. Cheon, Y. Chang, J. Yoo and J. Cheon, Angew. Chem., Int. Ed., 2008, 47, 6259 CrossRef CAS PubMed
.
- J. Zhang, J. G. Yu, Y. M. Zhang, Q. Li and J. R. Gong, Nano Lett., 2011, 11, 4774 CrossRef CAS PubMed
.
- J. G. Yu, J. Zhang and S. W. Liu, J. Phys. Chem. C, 2010, 114, 13642 CAS
.
- Q. W. Shu, C. M. Li, P. F. Gao, M. X. Gao and C. Z. Huang, RSC Adv., 2015, 5, 17458 RSC
.
- F. Dosio, P. Brusa, P. Crosasso, S. Arpicco and L. Cattel, J. Controlled Release, 1997, 47, 293 CrossRef CAS
.
- F. Kratza and U. Beyer, Drug Delivery, 1998, 5, 281 CrossRef PubMed
.
- Q. Chen, C. Wang, L. Cheng, W. W. He, Z. P. Cheng and Z. Liu, Biomaterials, 2014, 35, 2915 CrossRef CAS PubMed
.
- T. Desmettre, J. M. Devoisselle and S. Mordon, Surv. Ophthalmol., 2000, 45, 15 CrossRef CAS PubMed
.
- K. R. Weishaupt, C. J. Gomer and T. J. Dougherty, Cancer Res., 1976, 36, 2326 CAS
.
- M. L. Yin, Z. H. Li, E. G. Ju, Z. Z. Wang, K. Dong, J. S. Ren and X. G. Qu, Chem. Commun., 2014, 50, 10488 RSC
.
- B. Tian, C. Wang, S. Zhang, L. Feng and Z. Liu, ACS Nano, 2011, 5, 7000 CrossRef CAS PubMed
.
- B. Jang, J. Y. Park, C. H. Tung, I. H. Kim and Y. Choi, ACS Nano, 2011, 5, 1086 CrossRef CAS PubMed
.
- Q. W. Tian, F. R. Jiang, R. J. Zou, Q. Liu, Z. G. Chen, M. F. Zhu, S. P. Yang, J. L. Wang, J. H. Wang and J. Q. Hu, ACS Nano, 2011, 5, 9761 CrossRef CAS PubMed
.
- R. Chen, J. F. Zhang, Y. Wang, X. F. Chen, J. A. Zapien and C. S. Lee, Nanoscale, 2015, 7, 17299 RSC
.
- J. F. Zhang, Y. C. Liang, X. D. Lin, X. Y. Zhu, L. Yan, S. L. Li, X. Yang, G. Y. Zhu, A. L. Rogach, P. K. N. Yu, P. Shi, L. C. Tu, C. C. Chang, X. H. Zhang, X. F. Chen, W. J. Zhang and C. S. Lee, ACS Nano, 2015, 9, 9741 CrossRef CAS PubMed
.
- X. J. Yang, Z. Liu, Z. H. Li, F. Pu, J. S. Ren and X. G. Qu, Chem. – Eur. J., 2013, 19, 10388 CrossRef CAS PubMed
.
- L. N. Zhang, H. H. Deng, F. L. Lin, X. W. Xu, S. H. Weng, A. L. Liu, X. H. Lin, X. H. Xia and W. Chen, Anal. Chem., 2014, 86, 2711 CrossRef CAS PubMed
.
Footnote |
† Electronic supplementary information (ESI) available. See DOI: 10.1039/c5tb02002f |
|
This journal is © The Royal Society of Chemistry 2016 |
Click here to see how this site uses Cookies. View our privacy policy here.